DOI:
10.1039/C5SC02211H
(Edge Article)
Chem. Sci., 2015,
6, 6725-6730
Importance of dipole moments and ambient polarity for the conformation of Xaa–Pro moieties – a combined experimental and theoretical study†
Received
18th June 2015
, Accepted 27th July 2015
First published on 12th August 2015
Abstract
NMR spectroscopic studies with a series of proline derivatives revealed that the polarity of the environment has a significant effect on the trans
:
cis isomer ratio of Xaa–Pro bonds. Computational studies showed that this effect is due to differences in the overall dipole moments of trans and cis conformers. Comparisons between the conformational properties of amide and ester derivatives revealed an intricate balance between polarity effects and n → π* interactions of adjacent carbonyl groups. The findings have important implications for protein folding and signaling as well as the performance of proline-based stereoselective catalysts.
Introduction
Proline, the only cyclic proteinogenic amino acid, is often critically involved in protein folding and signaling.1 Prominent examples are collagen and proline-rich protein domains with two or more adjacent proline residues.2,3 Key to this unique role of proline is the isomerization of tertiary Xaa–Pro amide bonds between cis and trans conformers (Fig. 1).1–4 These moieties are present in water exposed domains of proteins as well as hydrophobic environments within membrane proteins.1,5,6 Furthermore, peptides bearing Xaa–Pro moieties have become popular as metal-free catalysts for a range of different reactions, including stereoselective C–C bond formations and acyl transfer reactions.7,8 The majority of these peptidic catalysts perform best in organic solvents. Understanding the factors that influence the trans–cis equilibrium of Xaa–Pro bonds in both aqueous and hydrophobic environment is therefore important.
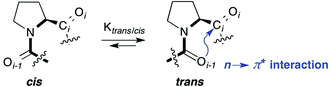 |
| Fig. 1
Trans and cis conformers of Xaa–Pro bonds. | |
The trans conformer is favored over the cis conformer in Xaa–Pro bonds by an interaction between the adjacent carbonyl groups (Fig. 1). This n → π* interaction involves the donation of electron density from the oxygen Oi−1 lone pair (n) into the π* orbital of the adjacent carbonyl group (Ci = Oi).9,10 In addition, steric effects can further favor or disfavor the trans over the cis conformer.11
To tune the conformational and functional properties of peptides and proteins, numerous proline derivatives with electron withdrawing, sterically demanding, or H-bond donating substituents, e.g. at C(4), have been developed that favor or disfavor the trans over the cis conformer compared to unsubstituted proline residues.12–21
Acetylated methyl esters (Ac-Xaa-OMe) of proline and proline derivatives are commonly used model compounds to analyze the factors that determine the trans
:
cis conformer ratio (Fig. 2, left).13–18 They are preferred models compared to secondary amides Ac-Xaa-NHMe (Fig. 2, middle) that favor the trans conformer by donating an intramolecular H-bond and thereby obscure weaker interactions.22 Yet, esters are more electrophilic than amides and are therefore also not ideal models for analyzing the factors that affect the trans
:
cis amide equilibrium of Xaa–Pro bonds. Tertiary amides on the other hand cannot engage in an intramolecular H-bond and have a comparable electrophilicity as secondary amides (Fig. 2, right). They reflect in particular segments of peptides and proteins with two adjacent proline residues, which are common in collagen and proline-rich protein domains.2,3 We therefore envisioned acetylated dimethyl amides Ac-Xaa-NMe2 as appropriate models to allow insight into the factors that influence the trans
:
cis conformer ratio of Xaa–Pro bonds.
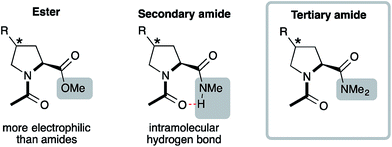 |
| Fig. 2 Acetylated model compounds bearing an ester (Ac-Xaa-OMe), secondary amide (Ac-Xaa-NHMe) and tertiary amide (Ac-Xaa-NMe2). | |
Herein we examined the conformational properties of dimethyl amide proline derivatives in aqueous and organic media by NMR spectroscopic and computational studies. We show that the trans
:
cis ratio of Xaa–Pro bonds is significantly affected by the polarity of the environment. Comparisons between different proline derivatives revealed that the polarity effects are in a fine balance with n → π* interactions. Furthermore, we demonstrate how the solvent affects the conformation and the catalytic performance of a tripeptidic catalyst.
Results and discussion
Conformational properties of proline derivatives Ac-Pro-OMe and Ac-Pro-NMe2 in polar and apolar solvents
NMR spectroscopic studies.
We started by analyzing the conformational properties of the acetylated methyl ester and dimethyl amide of proline, Ac-Pro-OMe (1-OMe) and Ac-Pro-NMe2 (1-NMe2), by 1H NMR spectroscopy. Spectra of 1-OMe and 1-NMe2 were recorded in the polar solvents D2O and DMSO-d6 as well as CDCl3 and dioxane-d8 as representatives of less polar solvents. All spectra showed two sets of signals corresponding to minor cis and major trans conformers due to their slow interconversion.23 In agreement with previous studies, trans
:
cis ratios of 4.6 and 3.8 were observed for 1-OMe and 1-NMe2 in D2O, respectively (Fig. 3, light blue).9 Similarly the trans
:
cis conformer ratio of 1-OMe is also in DMSO-d6 (Ktrans/cis = 3.6) higher compared to that of 1-NMe2 (Ktrans/cis = 2.0, Fig. 3, blue). The lower trans
:
cis ratios of the dimethyl amide in polar solvents are indicative of weaker n → π* interactions between the adjacent carbonyls and in agreement with the lower electrophilicity of amide compared to ester moieties.
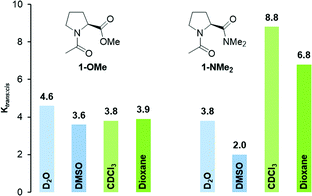 |
| Fig. 3
K
trans:cis values of Ac-Pro-OMe (1-OMe) and Ac-Pro-NMe2 (1-NMe2) determined by NMR-spectroscopy of 80 mM solutions in D2O and CDCl3, DMSO-d6, dioxane-d8. | |
Surprisingly, in the less polar solvents CDCl3 and dioxane-d8 the opposite trend was observed. In both solvents, the trans
:
cis conformer ratio of the amide 1-NMe2 is significantly higher compared to that of the ester 1-OMe (Fig. 3, green and light green). For example, in CDCl3 the equilibrium constant Ktrans/cis of 1-NMe2 is more than twice as high as that of 1-OMe (Ktrans/cis = 8.8 and 3.8, respectively).
These findings can neither be explained by interactions between adjacent carbonyl groups nor steric effects and show that an additional factor is contributing to the trans
:
cis conformer ratio of Xaa–Pro bonds. They are also unexpected with regard to the conformational properties of oligoprolines with more than six residues that adopt PPII helices with all-trans amide bonds in water and PPI helices with all-cis amide bonds in less polar solvents (e.g.iPrOH).24,25 These conformational preferences are due to hydration of the amides in PPII helices where they are oriented perpendicular to the axis and therefore solvent exposed, whereas the amides in PPI helices are aligned along the axis and not solvent exposed.25 The preference of the trans-conformer of 1-NMe2 in apolar solvents observed herein suggests that the helicity of oligoprolines precludes conformation-directing effects that are important within small peptides and segments of proteins without defined secondary structures.
Since the polarity of the solvent led to the observed differences between the prolyl ester and amide, we suspected that differences in the polarity of the trans and cis conformers are key for the observed equilibrium constants Ktrans/cis. To evaluate this hypothesis, we determined the overall dipole moments of the trans and cis conformers of the ester 1-OMe and the amide 1-NMe2 by quantum chemical calculations.
Computational studies.
We started by determining the geometries of the lowest energy conformations of the cis and trans isomers at the PBE0-D3/def2-TZVP level of theory using the Turbomole program package (versions 6.3.1 and 6.6).26,27 Thermal corrections for obtaining the Gibbs free energies at 298.15 K were calculated for all minima from unscaled vibrational frequencies obtained at the same level and were combined with single point energies calculated at the RI-MP2(ref. 28)/def2-QZVP//PBE0-D3/def2-TZVP level to yield Gibbs free energies at 298.15 K. Solvation effects were considered by the implicit solvation model COSMO.29 We employed this continuum solvation model rather than explicit solvent molecules that would require difficult sampling over the conformational space of solvent molecule arrangements and, in particular, would make the definition of the dipole moment difficult, since it is not well defined how many of the solvent molecules would need to be included in the dipole moment calculation. For both proline derivatives C(4)-endo puckered pyrrolidine rings were predicted to be energetically slightly more favored (by <1 kcal mol−1) compared to C(4)-exo puckers in case of the cis and the trans conformers. This is in good agreement with crystal structures and previously calculated structures of proline and proline derivatives.30,31 In the lowest energy structures of the trans conformers of both 1-OMe and 1-NMe2 (Fig. 4, Grel = 0.0 kcal mol−1) the Ψ-angles are ∼155° and the typical indicators of n → π* interactions between the adjacent carbonyl groups are present:9,10,32 The Oi−1⋯Ci distances are within the van-der-Waals radii of the interaction partners (<3.2 Å), the Bürgi–Dunitz trajectory angles Oi−1⋯Ci–Oi (ΘBD) are around 95°, and Ci is not planar but pyramidalized (see ESI for details†). The values are less pronounced in case of the amide compared to the ester (e.g., Oi−1⋯Ci 3.10 Å 1-NMe2versus 3.02 Å 1-OMe). This is indicative of weaker n → π* interactions as expected for the less electrophilic amide.
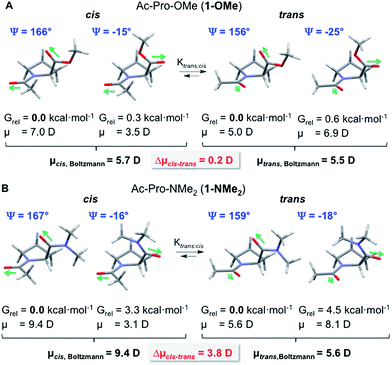 |
| Fig. 4 Lowest energy structures and their relative Gibbs free energies at 298 K (Grel) and Boltzmann-averaged dipole moments μ [D] of trans and cis conformers of Ac-Pro-OMe (1-OMe) and Ac-Pro-NMe2 (1-NMe2) calculated with CHCl3 as solvent at the PBE0-D3-COSMO/def2-TZVP level of theory. For clarity, only the values of the C(4)-endo conformers are listed. The values hardly change when also the C(4)-exo conformers are taken into account, see the ESI.† | |
For the trans and cis conformers of the ester 1-OMe, additional low energy structures (Grel < 1 kcal mol−1) with Ψ-angles of approximately −20° were found (Fig. 4A). Conformations with similar Ψ-angles are also in case of the dimethyl amide 1-NMe2 the next lowest energy structures. Yet, they have significantly higher energies (Grel > 3 kcal mol−1) due to steric repulsion between the dimethyl amide moiety and the pyrrolidine ring (Fig. 4B).
Rotation around the Ψ-angle affects the relative orientation of the carbonyl moieties significantly and was expected to affect the overall dipole moments of the cis and trans conformers. We therefore systematically changed the Ψ-angle within the identified lowest energy structures by steps of 30° and performed a constrained geometry optimization of each of these conformers to ensure that the conformations with Ψ = ∼160° and −20° are the global and local energy minimum structures. The dipole moments of these global and local energy minima structures were calculated on the same level of theory (PBE0-D3/def2-TZVP) as the Gibbs free energies. Overall dipole moments were then calculated as Boltzmann-averaged values over all available cis and trans conformers, respectively, of 1-OMe and 1-NMe2 based on their Gibbs free energies (Fig. 4, see the ESI for details†).
The overall dipole moments of the trans and cis conformers of ester 1-OMe are almost identical (Δμcis–trans = 0.2 D) since polarity differences are leveled out by the almost equal population of conformers with Ψ-angles of 160° and −20°. In contrast, the cis conformer of amide 1-NMe2 has a significantly higher dipole moment compared to the respective trans conformer (Δμcis–trans = 3.8 D). This is due to the higher population of the conformers with Ψ-angles around 160° within which the adjacent carbonyl moieties point into similar directions in the cis conformer but into different directions in the trans conformer (Fig. 4B).
These differences in the dipole moments corroborate the experimental findings: the less polar trans conformer is favored over the cis conformer significantly more in apolar than polar solvents. This is reflected in the observed higher trans
:
cis conformer ratio of the amide 1-NMe2 in apolar compared to polar solvents (e.g., Ktrans/cis = 8.8 in CDCl3 and Ktrans/cis = 3.8 in D2O, Fig. 3). Since the n → π* interaction between the adjacent amide groups is weak the trans
:
cis conformer ratios of amide 1-NMe2 is mainly controlled by the polarity of the environment. The situation is different in case of the ester 1-OMe. Here, the polarity of the environment has a minor effect and the strength of the n → π* interaction between the adjacent carbonyl groups controls Ktrans/cis.‡
Conformational properties of proline derivatives bearing electron withdrawing substituents
To probe the generality of these findings, we analyzed the solvent dependence of the trans
:
cis conformer ratios of (4S)- and (4R)-configured fluoroproline (Flp) and azidoproline (Azp) methyl esters (Ac-Xaa-OMe) and dimethyl amides (Ac-Xaa-NMe2). Fluoroprolines are among the most often used proline derivatives for tuning the trans
:
cis conformer ratio within peptides and proteins since they can be incorporated by chemical synthesis and protein expression into peptides and proteins.12,16 Azidoprolines are attractive since they allow for further derivatisation by, e.g., “click chemistry”.14,17,33 The electron-withdrawing fluoro and azido substituents are known to control the ring puckering of these derivatives by a stereoelectronic gauche effect.16,17 This leads in case of (4R)Flp and (4R)Azp to a preference of C(4)-exo puckering whereas (4S)Flp and (4S)Azp adopt C(4)-endo puckers preferentially (Fig. 5 and 6, top middle). Thus, these derivatives allow for probing whether the polarity effect is affected by the ring pucker and substituents at C(4).
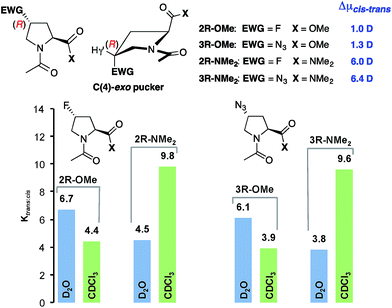 |
| Fig. 5
K
trans:cis values of (4R)-configured Ac-Xaa-OMe and Ac-Xaa-NMe2 of Flp and Azp derivatives determined by NMR-spectroscopy of 80 mM solutions in D2O (blue) and CDCl3 (green) and difference in the dipole moments (Δμcis–trans) of trans and cis conformers calculated with CHCl3 as solvent at the PBE0-D3-COSMO/def2-TZVP level of theory. | |
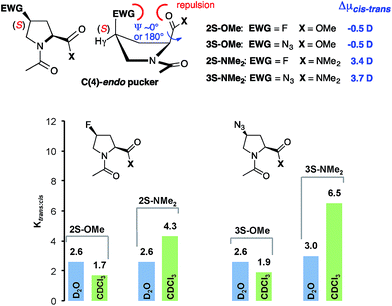 |
| Fig. 6
K
trans:cis values of (4S)-configured Ac-Xaa-OMe and Ac-Xaa-NMe2 of Flp and Azp derivatives determined by NMR-spectroscopy of 80 mM solutions in D2O (blue) and CDCl3 (green) and difference in the dipole moments (Δμcis–trans) of trans and cis conformers calculated with CHCl3 as solvent at the PBE0-D3-COSMO/def2-TZVP level of theory. | |
Calculations of the difference in the dipole moments (Δμcis–trans) of the cis and trans conformers with the lowest energies of all four proline derivatives by the methods described above showed a similar trend as for the unsubstituted prolines 1-OMe and 1-NMe2 (Fig. 5 and 6, see the ESI for the lowest energy structures†): regardless of the absolute configuration at C(4) and the ring pucker, the overall dipole moments of the trans and cis conformers of the methyl esters (2R/2S/3R/3S–OMe) are almost identical (Δμcis–trans = ±1 D) whereas those of the trans conformers of the amides (2R/2S/3R/3S–NMe2) are significantly higher compared to those of the respective cis conformers (Δμcis–trans = 2.5–6.3 D).
Thus, a similar trend is expected for the trans
:
cis conformer ratios in polar versus less polar solvents for these substituted proline derivatives as for 1-OMe and 1-NMe2. Indeed, in D2O the trans
:
cis conformer ratios of the (4R)-configured dimethyl amides 2R-NMe2 and 3R-NMe2 are lower than those of the methyl esters Ac-(4R)Flp-OMe 2R-OMe and Ac-(4R)Azp-OMe 3R-OMe (Fig. 5, blue).16,17 This reflects the lower electrophilicity of the amide compared to the ester and the thereby weakened n → π* interaction. In CDCl3 this trend is reversed and the trans
:
cis conformer ratios of the amides are significantly higher than those of the esters (Fig. 5, green), which underscores that the polarity controls their conformational properties.
In the diastereoisomeric (4S)-configured esters Ac-(4S)Flp-OMe 2S–OMe and Ac-(4S)Azp-OMe 3S–OMe the trans conformer is generally less favored (Ktrans/cis = 2.6) since a transannular electronic repulsion between the electron-rich F or N3 substituent and the carbonyl oxygen of the methyl ester or amide moiety places the adjacent carbonyl groups in an unfavorable position for an n → π* interaction (Fig. 6, top middle).18 Thus, the contribution of the n → π* interaction is less compared to that in unsubstituted proline.16,17 As a result, the esters and respective dimethyl amides have comparable trans
:
cis amide ratios in D2O (Fig. 6, blue). In CDCl3 the trans
:
cis conformer ratios of the amides are higher by a factor of 2–3 compared to those of the respective esters (Fig. 6, green). Thus, the trans
:
cis ratio is also within these substituted proline-amide derivatives predominantly controlled by the polarity of the solvent and the difference in the dipole moments of the trans and cis conformers.§
Trans
:
cis isomer ratio of short peptides with the Pro–Pro motive
Finally we probed whether the observed polarity effects also occur in short-chain peptides. Towards this goal we examined the trans
:
cis isomer ratio of the tripeptide H-Pro-Pro-Asp-NH2 (4) that is a known catalyst for aldol and conjugate addition reactions between aldehydes and nitroolefins (Fig. 7).34–36 Reassuringly, NMR spectroscopic analyses of the trifluoroacetic acid (TFA) salt of 4 in DMSO-d6 showed a trans
:
cis ratio of 3.4
:
1, whereas the trans
:
cis ratio is 6.0
:
1 in a mixture of CDCl3
:
MeOH-d4 9
:
1 (the peptide is not soluble in pure CDCl3). Thus, the trans isomer is also within this tripeptide more favored in apolar compared to polar environments, which shows how important the choice of the solvent is for the conformational properties of short chain peptides with the Pro–Pro motive.
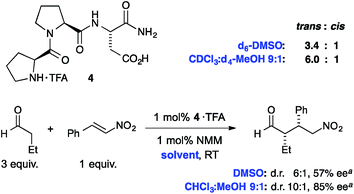 |
| Fig. 7
Trans : cis ratios of the tripeptidic catalyst H-Pro-Pro-Asp-NH2 (4) and stereoselectivities of 1,4-addition reactions between butanal and nitrostyrene. aData taken from ref. 35. | |
Interestingly, the diastereoselectivity (d.r.) and enantioselectivity (ee) of this peptidic catalyst in C–C bond forming reactions correlates with the trans
:
cis amide ratios and are significantly higher in a mixture of CHCl3
:
MeOH 9
:
1 than DMSO (Fig. 7, bottom). Whereas the polarity of the solvent is likely also affecting other factors, e.g., differences in the interaction strength of a putative imminium–nitronate intermediate with the carboxylic acid moiety of the catalyst,36 the data indicates that the trans
:
cis conformer ratio correlates with the stereochemical outcome of the reaction.
Conclusions
In conclusion, we have shown how important the polarity of the environment is for the trans
:
cis conformer equilibrium of proline derivatives that are connected by tertiary amide groups. Polar solvents favor the cis conformer, apolar environments the less polar trans conformer. The results also show that the trans
:
cis ratio of Xaa–Pro bonds is controlled by a fine balance of n → π* interactions between adjacent amides and the polarity of the environment. Since both are comparatively weak interactions, subtle structural or environmental changes can affect their contributions significantly. The results are particularly relevant for peptides and proteins containing Pro–Pro moieties, which are common motives in, e.g., collagen and catalytically active peptides. The presented findings provide a guide for influencing the trans
:
cis conformer ratio via the environment and thereby control the conformational and folding properties of peptides and proteins.
Notes and references
- For reviews see:
(a) K. P. Lu, G. Finn, T. H. Lee and L. K. Nicholson, Nat. Chem. Biol., 2007, 3, 619 CrossRef CAS PubMed
;
(b) A. H. Andreotti, Biochemistry, 2003, 42, 9515 CrossRef CAS PubMed
.
- M. D. Shoulders and R. T. Raines, Annu. Rev. Biochem., 2009, 78, 929 CrossRef CAS PubMed
.
- B. K. Kay, M. P. Williamson and M. Sudol, FASEB J., 2000, 14, 231 CAS
.
-
(a)
L. Moroder, C. Renner, J. J. Lopez, M. Mutter and G. Tuchscherer, in cis–trans Isomerization in Biochemistry, ed. E. Dugave, Wiley-VCH Verlag GmbH & Co. KGaA, 2006, DOI:10.1002/9783527609338.ch11, p. 225
;
(b) G. Fischer, Chem. Soc. Rev., 2000, 29, 119 RSC
.
- S. C. Lummis, D. L. Beene, L. W. Lee, H. A. Lester, R. W. Broadhurst and D. A. Dougherty, Nature, 2005, 438, 248 Search PubMed
.
-
(a) C. J. Brandl and C. M. Deber, Proc. Natl. Acad. Sci. U. S. A., 1986, 83, 917 Search PubMed
;
(b) A. Perálvarez-Marín, J.-L. Bourdelande, E. Querol and E. Padrós, Mol. Membr. Biol., 2006, 23, 127 Search PubMed
;
(c) D. N. Woolfson, R. J. Mortishire-Smith and D. H. Williams, Biochem. Biophys. Res. Commun., 1991, 175, 733 Search PubMed
.
-
(a) B. Lewandowski and H. Wennemers, Curr. Opin. Chem. Biol., 2014, 22, 40 Search PubMed
;
(b) H. Wennemers, Chem. Commun., 2011, 47, 12036 Search PubMed
;
(c) E. A. C. Davie, S. M. Mennen, Y. Xu and S. J. Miller, Chem. Rev., 2007, 107, 5759 Search PubMed
.
- For examples, see:
(a) J. Duschmale, S. Kohrt and H. Wennemers, Chem. Commun., 2014, 50, 8109 Search PubMed
;
(b) K. T. Barrett, A. J. Metrano, P. R. Rablen and S. J. Miller, Nature, 2014, 509, 7 Search PubMed
;
(c) R. Kastl and H. Wennemers, Angew. Chem., Int. Ed., 2013, 52, 7228–7232 Search PubMed
;
(d) J. Duschmale and H. Wennemers, Chem.–Eur. J., 2012, 18, 1111–1120 Search PubMed
;
(e) K. Akagawa and K. Kudo, Angew. Chem., Int. Ed., 2012, 51, 12786 Search PubMed
.
- R. W. Newberry, B. vanVeller, I. A. Guzei and R. T. Raines, J. Am. Chem. Soc., 2013, 135, 7843 Search PubMed
.
- M. P. Hinderaker and R. T. Raines, Protein Sci., 2003, 12, 1188 Search PubMed
.
-
(a) E. Beausoleil, R. Sharma, S. W. Michnick and W. D. Lubell, J. Org. Chem., 1998, 63, 6572 Search PubMed
;
(b) L. Halab, L. Bélec and W. D. Lubell, Tetrahedron, 2001, 57, 6439 Search PubMed
;
(c) N. G. Delaney and V. J. Madison, J. Am. Chem. Soc., 1982, 104, 6635 Search PubMed
.
-
(a) S. K. Holmgren, K. M. Taylor, L. E. Bretscher and R. T. Raines, Nature, 1998, 392, 666 Search PubMed
;
(b) B. Holzberger and A. Marx, J. Am. Chem. Soc., 2010, 132, 15708 Search PubMed
;
(c) W. Kim, R. A. McMillan, J. P. Snyder and V. P. Conticello, J. Am. Chem. Soc., 2005, 127, 18121 Search PubMed
;
(d) D. Naduthambi and N. J. Zondlo, J. Am. Chem. Soc., 2006, 128, 12430 Search PubMed
;
(e) T. Y. Zheng, Y. J. Lin and J. C. Horng, Biochemistry, 2010, 49, 4255 Search PubMed
;
(f) R. Golbik, C. Yu, E. Weyher-Stingl, R. Huber, L. Moroder, N. Budisa and C. Schiene-Fischer, Biochemistry, 2005, 44, 16026 Search PubMed
.
-
(a) F. W. Kotch, I. A. Guzei and R. T. Raines, J. Am. Chem. Soc., 2008, 130, 2952 Search PubMed
;
(b) S. A. Cadamuro, R. Reichold, U. Kusebauch, H.-J. Musiol, C. Renner, P. Tavan and L. Moroder, Angew. Chem., Int. Ed., 2008, 47, 2143 Search PubMed
;
(c) M. D. Shoulders, I. A. Guzei and R. T. Raines, Biopolymers, 2008, 89, 443 Search PubMed
;
(d) C. L. Jenkins, A. I. McCloskey, I. A. Guzei, E. S. Eberhardt and R. T. Raines, Biopolymers, 2005, 80, 1 Search PubMed
;
(e) M. D. Shoulders, J. A. Hodges and R. T. Raines, J. Am. Chem. Soc., 2006, 128, 8112 Search PubMed
.
-
(a) R. S. Erdmann and H. Wennemers, J. Am. Chem. Soc., 2010, 132, 13957 Search PubMed
;
(b) R. S. Erdmann and H. Wennemers, Angew. Chem., Int. Ed., 2011, 50, 6835 Search PubMed
;
(c) R. S. Erdmann and H. Wennemers, J. Am. Chem. Soc., 2012, 134, 17117 Search PubMed
;
(d) R. S. Erdmann and H. Wennemers, Org. Biomol. Chem., 2012, 10, 1982 Search PubMed
.
- C. Siebler, R. S. Erdmann and H. Wennemers, Angew. Chem., Int. Ed., 2014, 53, 10340 Search PubMed
.
-
(a) L. E. Bretscher, C. L. Jenkins, K. M. Taylor, M. L. DeRider and R. T. Raines, J. Am. Chem. Soc., 2001, 123, 777 Search PubMed
;
(b) M. L. DeRider, S. J. Wilkens, M. J. Waddell, L. E. Bretscher, F. Weinhold, R. T. Raines and J. L. Markley, J. Am. Chem. Soc., 2002, 124, 2497 Search PubMed
;
(c) C. Renner, S. Alefelder, J. H. Bae, N. Budisa, R. Huber and L. Moroder, Angew. Chem., Int. Ed., 2001, 40, 923 Search PubMed
.
- L. S. Sonntag, S. Schweizer, C. Ochsenfeld and H. Wennemers, J. Am. Chem. Soc., 2006, 128, 14697 Search PubMed
.
- M. Kuemin, Y. A. Nagel, S. Schweizer, F. W. Monnard, C. Ochsenfeld and H. Wennemers, Angew. Chem., Int. Ed., 2010, 49, 6324 Search PubMed
.
- A. M. P. Koskinen, J. Helaja, E. T. T. Kumpulainen, J. Koivisto, H. Mansikkamäki and K. Rissanen, J. Org. Chem., 2005, 70, 6447 Search PubMed
.
- C. Martin, B. Legrand, A. Lebrun, D. Berthomieu, J. Martinez and F. Cavelier, Chem.–Eur. J., 2014, 20, 14240 Search PubMed
.
- A. K. Pandey, D. Naduthambi, K. M. Thomas and N. J. Zondlo, J. Am. Chem. Soc., 2013, 135, 4333–4363 Search PubMed
.
-
(a) T. Higashijima, M. Tasumi and T. Miyazawa, Biopolymers, 1977, 16, 1259 Search PubMed
;
(b) G.-B. Liang, C. J. Rito and S. H. Gellman, Biopolymers, 1992, 32, 293 Search PubMed
;
(c) R. Improta, C. Benzi and V. Barone, J. Am. Chem. Soc., 2001, 123, 12568 Search PubMed
.
- The cis and trans conformers were assigned by NOESY,
for details, see the ESI†.
-
(a) M. De Zotti, F. Formaggio, M. Crisma, C. Peggion, A. Moretto and C. Toniolo, J. Pept. Sci., 2014, 20, 307 Search PubMed
;
(b) F. Rabanal, M. D. Ludevid, M. Pons and E. Giralt, Biopolymers, 1993, 33, 1019 Search PubMed
.
- M. Kuemin, S. Schweizer, C. Ochsenfeld and H. Wennemers, J. Am. Chem. Soc., 2009, 131, 15474 Search PubMed
.
-
(a) C. Adamo, G. E. Scuseria and V. J. Barone, Chem. Phys., 1999, 111, 2889 Search PubMed
;
(b) S. Grimme, J. Antony, S. Ehrlich and H. Krieg, J. Chem. Phys., 2010, 132, 154104 Search PubMed
;
(c) F. Weigend and R. Ahlrichs, Phys. Chem. Chem. Phys., 2005, 7, 3297 Search PubMed
.
- TURBOMOLE V6.6, 2014, V6.3 2011, a development of University of Karlsruhe and Forschungszentrum Karlsruhe GmbH, 1989–2007, TURBOMOLE GmbH, since 2007, available from http://www.turbomole.com.
-
(a) F. Weigend and M. Häser, Theor. Chem. Accounts Theor. Comput. Model. Theor. Chim. Acta., 1997, 97, 331 Search PubMed
;
(b) F. Weigend, M. Häser, H. Patzelt and R. Ahlrichs, Chem. Phys. Lett., 1998, 294, 143 Search PubMed
.
- A. Klamt and G. Schüürmann, J. Chem. Soc., Perkin Trans. 2, 1993, 799 Search PubMed
.
- N. Panasik, E. S. Eberhardt, A. S. Edison, D. R. Powell and R. T. Raines, Int. J. Pept. Protein Res., 1994, 44, 262 Search PubMed
.
-
(a) Y. Kee Kang and H. Sook Park, Biophys. Chem., 2005, 113, 93–101 Search PubMed
;
(b) C. Benzi, R. Improta, G. Scalmani and V. Barone, J. Comput. Chem., 2002, 23, 341 Search PubMed
.
- P. Wilhelm, B. Lewandowski, N. Trapp and H. Wennemers, J. Am. Chem. Soc., 2014, 136, 15829 Search PubMed
.
-
(a) B. Amgarten, R. Rajan, N. Martinez-Saez, B. L. Oliveira, I. S. Albuquerque, R. A. Brooks, D. G. Reid, M. J. Duer and G. J. L. Bernardes, Chem. Commun., 2015, 51, 5250 Search PubMed
;
(b) C. Kroll, R. Mansi, F. Braun, S. Dobitz, H. R. Maecke and H. Wennemers, J. Am. Chem. Soc., 2013, 135, 16793 Search PubMed
;
(c) D. M. Ryan, M. K. Coggins, J. J. Concepcion, D. L. Ashford, Z. Fang, L. Alibabaei, D. Ma, T. J. Meyer and M. L. Waters, Inorg. Chem., 2014, 53, 8120 Search PubMed
;
(d) K. M. Bonger, V. V. Kapoerchan, G. M. Grotenbreg, C. J. van Koppen, C. M. Timmers, G. A. van der Marel and H. S. Overkleeft, Org. Biomol. Chem., 2010, 8, 1881 Search PubMed
;
(e) S. T. Le Quement, M. Ishoey, M. T. Petersen, J. Thastrup, G. Hagel and T. E. Nielsen, ACS Comb. Sci., 2011, 13, 667 Search PubMed
;
(f) M. Kümin, L.-S. Sonntag and H. Wennemers, J. Am. Chem. Soc., 2007, 129, 466 Search PubMed
;
(g) U. Lewandowska, W. Zajaczkowski, L. Chen, F. Bouillière, D. Wang, K. Koynov, W. Pisula, K. Müllen and H. Wennemers, Angew. Chem., 2014, 53, 12537 Search PubMed
.
- P. Krattiger, R. Kovasy, J. D. Revell, S. Ivan and H. Wennemers, Org. Lett., 2005, 7, 1101 Search PubMed
.
- M. Wiesner, J. D. Revell and H. Wennemers, Angew. Chem., Int. Ed., 2008, 47, 1871 Search PubMed
.
- J. Duschmale, J. Wiest, M. Wiesner and H. Wennemers, Chem. Sci., 2013, 4, 1312 Search PubMed
.
Footnotes |
† Electronic supplementary information (ESI) available: Synthesis and analytical details of all compounds, details on the computational analysis. See DOI: 10.1039/c5sc02211h |
‡ It is noteworthy that the trans : cis ratios of the esters are generally higher in D2O than CDCl3. This could be due to a better solvation and thereby stabilization of the increased charge separation induced by the n → π* interaction between the adjacent carbonyl groups within the trans conformer.
|
§ For a detailed listing of the calculated dipole moments and the structures, see the ESI.† |
|
This journal is © The Royal Society of Chemistry 2015 |
Click here to see how this site uses Cookies. View our privacy policy here.