DOI:
10.1039/C5RA26520G
(Paper)
RSC Adv., 2016,
6, 21293-21301
HPLC enantioseparation on a homochiral MOF–silica composite as a novel chiral stationary phase†
Received
12th December 2015
, Accepted 15th February 2016
First published on 16th February 2016
Abstract
The last frontier in the development of chiral stationary phases for chromatographic enantioseparation involves homochiral metal–organic frameworks (MOFs). Using enantiopure (R)-2,2′-dihydroxy-1,1′-binaphthalene-6,6′-dicarboxylic acid as a starting material, we prepared three homochiral MOFs that were further used as chiral stationary phases for high-performance liquid chromatography to separate the enantiomers of various kinds of racemic sulfoxides, sec-alcohols, β-lactams, benzoins, flavanones and epoxides. The experimental results showed excellent performances for enantioseparation, and highlighted that enantioseparation on homochiral MOF columns is practical.
Introduction
Metal–organic frameworks (MOFs) are a novel class of porous materials that are built using metal ions and organic linkers.1 They are promising materials for many applications, such as gas storage and separation,2 catalysis,3 and sensors.4 This is because of their advantageous characteristics, such as high surface area, large pore size, high thermal and chemical stability, uniformly structured cavities and availability for modification. Recently, MOFs have attracted increased attention as new stationary phases for chromatography.5 In particular, homochiral MOFs have attracted considerable attention because of their applications in enantioselective separations and catalysis. Microporous MOFs with good solvent stability are attractive stationary phases for high-performance liquid chromatography (HPLC) and some achiral MOFs have been successfully used as stationary phases for HPLC separation of small achiral molecules. However, examples of chromatographic separations of enantiomers using homochiral MOFs are rare and only a few chiral MOFs have been studied as chiral stationary phases (CSPs).6
The first attempt to use chiral MOFs as CSPs in preparative column chromatography was reported by Fedin et al.6a In their work, a column was prepared by loading a glass tube with a suspension of 3D porous chiral MOF [Zn2-(bdc)(l-lac)(dmf)]·DMF. Resulting chromatograms showed that PhSOMe enantiomers were completely separated using the column, while other sulfoxide enantiomers with electron-withdrawing substituents showed reduced enantioselectivity. Kaskel et al. then synthesized and packed two new chiral-UMCM-1 analogues into HPLC columns.6b The chiral-UMCM-1 was selective for 1-phenyl-1-ethanol enantiomers in n-heptane, but the resolution was too low and strong tailing occurred. Yuan and coworkers reported another chiral MOF ([(CH3)2NH2][Cd(bpdc)1.5]·2DMA) as a CSP.6d This MOF successfully separated some different enantiomers: 1,1-bi-2-naphthol, 1,2-diphenyl-1,2-ethanediol, 1-(4-chlorophenyl)ethanol, furoin, benzoin, flavanone, Troger's base, 3-benzyloxy-1,2-propanediol, 3,5-dinitro-N-(1-phenylethyl)-benzamide and warfarin sodium. Hydrogen bonding interactions played a vital role in the chiral separation because of the poorer resolution obtained using hexane–isopropanol than hexane–dichloromethane as the mobile phase. Tang and coworkers reported separation for racemic ibuprofen, phenylethylamine and benzoin using MOFs ({[ZnLBr]·H2O}n) synthesized using the chiral organic linker N-(4-pyridylmethyl)-L-leucine·HBr.6e Recently, Cui et al. reported a 1,1′-biphenol-based chiral MOF containing one-dimensional nanosized channels decorated with chiral dihydroxy group for the separation of racemic aromatic amines and amides.6f It should be noted that almost all of the MOFs reported previously featured narrow ranges of chiral enantioselectivity.
Our laboratory has reported chiral HPLC enantiomer separation using (R)-CuMOF-1–silica composite as a CSP that was synthesized from a mixture of (R)-2,2′-dihydroxy-1,1′-binaphthalene-6,6′-dicarboxylic acid 1 and Cu(NO3)2 in DMF.6c The packed column (10 cm length, 4.6 mm id) successfully separated 15 sulfoxide enantiomers with hexane–EtOH (50/50) and 16 enantiomers with hexane–i-PrOH (90/10) as mobile phases. Exploration of chiral MOFs as stationary phases for diverse chiral compound separation is still required because chiral separations are extremely important. Here we report the investigation of some homochiral MOF–silica composites prepared from (R)-2,2′-dihydroxy-1,1′-binaphthalene-6,6′-dicarboxylic acid 1 and (R)-2,2′-dimethoxy-1,1′-binaphthalene-6,6′-dicarboxylic acid 2 as CSPs for enantiomeric HPLC separation of a wide range of racemates, such as sulfoxides, sec-alcohols, β-lactams, benzoins, flavanones and epoxides.
Results and discussion
Synthesis and characterization of chiral MOFs
Crystalline (R)-CuMOF-1 was prepared using a slightly modified version of the method previously reported by Lin et al.7 by heating a DMF solution containing (R)-2,2′-dihydroxy-1,1′-binaphthalene-6,6′-dicarboxylic acid 1 and Cu(NO3)2·3H2O at 80 °C for 18 h. Crystals of (R)-ZnMOF-1 were obtained by heating a mixture of (R)-2,2′-dihydroxy-1,1′-binaphthalene-6,6′-dicarboxylic acid 1 and Zn(NO3)2·6H2O in a mixture of N-methylformamide (NMF) and EtOH at 80 °C for 24 h. (R)-CuMOF-2 was prepared by heating a mixture of (R)-2,2′-dimethoxy-1,1′-binaphthalene-6,6′-dicarboxylic acid 2 and Cu(NO3)2·3H2O in a solvent mixture of N,N-dimethylacetamide (DMA) and H2O at 60 °C for 48 h.
Single crystal X-ray analysis revealed that (R)-ZnMOF-1 existed as [Zn[(R)-1]2(NMF)2]·NMF, which crystallized in the space group C2221. The asymmetric unit contains one Zn(II), NMF and half of a molecule of (R)-1, and a guest NMF molecule in which the methyl group was disordered over two positions with an occupancy factor of 0.68/0.32. The Zn atom in mononuclear (R)-ZnMOF-1 is six-coordinated in a geometry best described as a skewed-trapezoidal bipyramid, in which four oxygen atoms are from the asymmetric bidentate carboxylate groups (Zn1–O2, 2.093 Å; Zn1–O3, 2.243 Å) of (R)-1 and two from NMF molecules (Zn1–O4, 2.020 Å). A zigzag 1D chain is formed by –[Zn–(R)-1]– coordination and extends along the c-axis (Fig. 1).
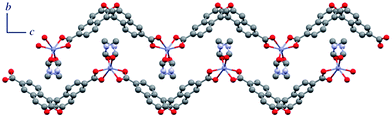 |
| Fig. 1 1D chain structure of (R)-ZnMOF-1 composed of Zn[(R)-1]2(NMF)2 along the c axis. Guest NMF molecules and H-atoms are omitted for clarity. | |
Single crystal X-ray analysis revealed that (R)-CuMOF-2 was formulated as [Cu2[(R)-2]4(H2O)2]·DMA, which crystallized in the space group C2. The asymmetric unit contains one Cu(II), half of a (R)-2 moiety, one coordinated water molecule and a DMA molecule as a guest. The structure consists of dinuclear Cu units and is typical of dinuclear [M2(carboxylate)4L2] complexes, with (R)-2 ligands providing the four metal-bridging bidendate caboxylate groups (Cu1–Cu1*, 2.625 Å; Cu1–O3RCOO, 1.976 Å; Cu1*–O4RCOO, 2.016 Å; symmetry code*, 1 − x, y, −z) and two oxygen atoms (Cu–O8w, 2.217 Å). A 1D chain structure is constructed from (R)-2-bridged –[Cu2–[(R)-2]2]– along the a-axis (Fig. 2).
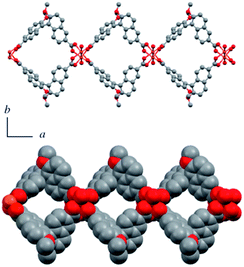 |
| Fig. 2 1D chain structure of (R)-CuMOF-2 composed of Cu2[(R)-2]4(H2O)2 along the a axis (top). A space-filling model of the 1D chain (bottom). DMA molecules and H-atoms are omitted for clarity. | |
In the crystal of (R)-CuMOF-1,7 1D chain structures stack indirectly on each other (red and blue molecules in Fig. 3, bottom) to form hourglass-shaped channels with diameters of approximately 6.2 Å along the a-axis. For the (R)-CuMOF-2 crystal, the chain structures lie directly on top of each other (red and blue molecules in Fig. 3, top) and the chain structure is tilted at 69.2° relative to the stacking direction (c-axis, Fig. 3) so that the channels adopt almost cylindrical shapes. On this basis we speculate that chiral guest molecules are able to enter and readily pass through the chiral channels of (R)-CuMOF-1 in Fig. 3 (bottom). (R)-CuMOF-2 crystals have channels running along the c-axis in Fig. 3 (top). The volume and diameter of the channel in the crystal lattice were 197 Å3 and 4.4 Å, respectively. There were no guest molecules in the channels of the (R)-CuMOF-2 crystal. The poor enantioselectivity on (R)-CuMOF-2 may be because of the small entrance to the channel of (R)-CuMOF-2. In the crystal of (R)-ZnMOF-1, twelve discrete voids of 18–25 Å3 are present in the unit cell (Fig. 4).
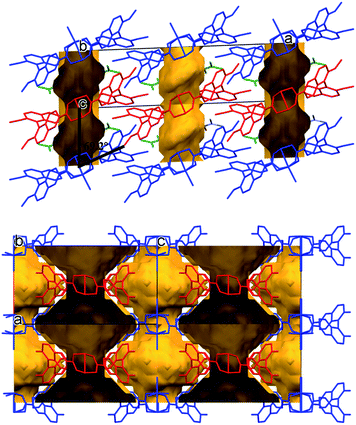 |
| Fig. 3 The channel structures in (R)-CuMOF-2 crystal (top) and (R)-CuMOF-1 crystal (bottom, from ref. 7) viewed along the b-axis. The lattice channels in both crystals are depicted as yellow Connolly surface visualized with mercury. H-atoms are omitted for clarity. | |
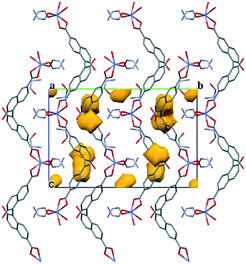 |
| Fig. 4 Crystal structure of (R)-ZnMOF-1. The lattice voids are depicted as yellow Connolly surface visualized with mercury. Guest NMF molecules and H-atoms are omitted for clarity. | |
(R)-CuMOF-1–silica composite was prepared according to the previously reported method.6c (R)-ZnMOF-1–silica composite was prepared by heating a mixture of (R)-1, Zn(NO3)2·6H2O and Daisogel (SP-120-7P) in a mixture of NMF and EtOH at 80 °C for 24 h. The resulting green precipitate was filtered and washed with MeOH, and dried in vacuo at 80 °C. (R)-CuMOF-2–silica composite was also prepared by heating a mixture of (R)-2, Cu(NO3)2·3H2O and Daisogel (SP-120-7P) in a mixture of DMA and H2O at 80 °C for 18 h. The resulting green precipitate was filtered and washed with MeOH, and dried in vacuo at 80 °C. The successful preparation of a series of (R)-CuMOF-1–silica composite, (R)-ZnMOF-1–silica composite and (R)-CuMOF-2–silica composite was confirmed by scanning electron microscopy (SEM) (Fig. S7–S9†), powder X-ray diffraction (PXRD) (Fig. S10†), and N2 physisorption data (Fig. S11) in ESI.† The packed columns for HPLC were prepared by loading the suspension of MOF–silica composite in hexane/i-PrOH (90
:
10) into a stainless steel column (10 cm length × 4.6 mm i.d.).
Chromatographic enantiomer separation on (R)-CuMOF-1
To investigate the chiral recognition ability of the (R)-CuMOF-1–silica composite packed column, various sulfoxide racemates (Fig. 5) were first selected as test solutes. Table 1 shows the separation (α) and retention (Rs) factors for the resolution efficiency. As can be seen from the Table 1, fourteen (3, 6, 7, 8, 9, 10, 15, 19, 20, 22 and 25) out of 23 sulfoxides were resolved with good-to-excellent selectivity using hexane/EtOH (50/50) as the mobile phase. For the resolution of sulfoxides 4, 5, 11, 16, 17, 18, 21, 23 and 24, separation factors were improved when the mobile phase was changed from hexane/EtOH (50/50) to hexane/i-PrOH (90/10). The chromatograms showed clear baseline separation.
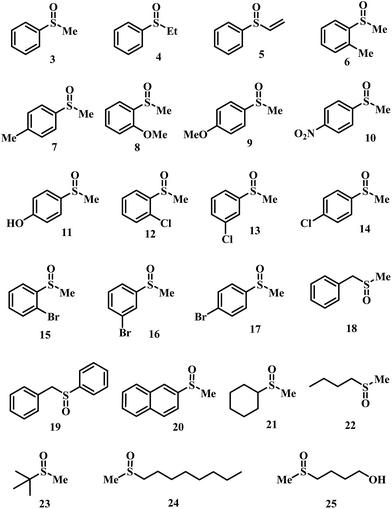 |
| Fig. 5 Chemical structures of selected sulfoxides. | |
Table 1 Chromatographic data for the resolution of sulfoxides6c
Compound |
Eluent Ia |
Eluent IIb |
αc |
Rsd |
α |
Rs |
Hexane/EtOH (50/50). Hexane/i-PrOH (90/10). Calculated from k′2/k′1, where k′1 = (t1 − t0)/t0 and k′2 = (t2 − t0)/t0. The retention times for the faster and slower moving enantiomers are designated as t1 and t2, respectively, and that for 1,3,5-tri-tert-butylbenzene used as a void volume marker is designated as t0. Calculated from 2(t2 − t1)/ω1 − ω2, where ω is peak width. |
3 |
4.40 |
1.50 |
3.99 |
1.33 |
4 |
1.92 |
1.03 |
2.80 |
0.84 |
5 |
1.63 |
0.92 |
2.39 |
0.87 |
6 |
1.93 |
0.75 |
1.47 |
0.65 |
7 |
5.71 |
1.53 |
2.16 |
1.01 |
8 |
1.33 |
0.35 |
1.31 |
0.36 |
9 |
2.59 |
1.08 |
1.43 |
0.78 |
10 |
2.31 |
1.20 |
1.63 |
1.07 |
11 |
1.32 |
0.15 |
1.40 |
0.33 |
12 |
1.24 |
0.33 |
1.09 |
0.06 |
13 |
1.99 |
0.93 |
1.95 |
1.04 |
14 |
2.11 |
1.06 |
1.90 |
0.79 |
15 |
1.90 |
0.52 |
1.00 |
— |
16 |
1.67 |
0.57 |
2.49 |
0.68 |
17 |
2.94 |
1.07 |
3.03 |
0.94 |
18 |
1.00 |
— |
2.01 |
0.84 |
19 |
2.38 |
1.14 |
1.77 |
0.93 |
20 |
1.24 |
0.17 |
1.00 |
— |
21 |
1.00 |
— |
2.23 |
1.36 |
22 |
1.25 |
0.49 |
1.20 |
0.39 |
23 |
1.00 |
— |
1.05 |
0.18 |
24 |
1.00 |
— |
1.27 |
0.31 |
25 |
1.28 |
0.71 |
1.00 |
— |
Sulfoxides 18 and 21, for example, which were not resolved when hexane/EtOH (50/50) was used, had excellent resolution using less polar hexane/i-PrOH (90/10) as the mobile phase. It is interesting to note that the chiral recognition of substituted sulfoxides occurred more efficiently for the para-substituted derivatives than the meta- and ortho-substituted derivatives may be due to the steric reasons. For example, α values for o-chlorophenyl methyl sulfoxide 12, m-chlorophenyl methyl sulfoxide 13 and p-chlorophenyl methyl sulfoxide 14 were 1.24, 1.99 and 2.11, respectively, as shown in Fig. 6. Interestingly, the (S)-isomer of the sulfoxides eluted before the (R)-isomer in all cases tested, suggesting stronger retention of the (R)-enantiomer in the cavity of (R)-CuMOF-1. This was likely because of intermolecular hydrogen bond interactions between the phenolic OH group of (R)-CuMOF-1 and the O
S group of the sulfoxide.
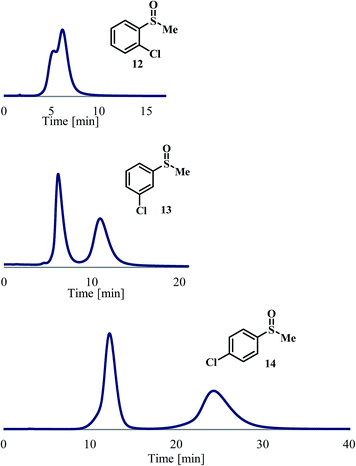 |
| Fig. 6 HPLC chromatogram for the resolution of methyl chlorophenyl sulfoxide isomers (12–14) using eluent I as the mobile phase at a flow rate of 1.0 mL min−1 with a UV detector at 254 nm. | |
In the HPLC resolution of sec-alcohols 26–47, the composition of the mobile phase played a significant role in retention and resolution. Table 2 presents α and Rs values for the resolution of sec-alcohols (26–47) (Fig. 7) using different mobile phases (I: hexane/EtOH = 50/50, III: hexane/i-PrOH = 99/1 and IV: hexane/CH2Cl2 = 10/90). In contrast to the binary phase hexane/EtOH (50/50), the less polar hexane/i-PrOH (99/1) mobile phase produced excellent resolution of sec-alcohols 26, 27, 28, 34, 37, 40, and 42 (Fig. 8 and Table 2). When the mobile phase was changed from hexane/i-PrOH (99/1) to hexane/CH2Cl2 (10/90), the resolution efficiencies for sec-alcohols 29, 30, 31, 33, 35, 36, 38, 39, 41, and 43–47 were substantially improved (Table 2). The observed mobile phase influence on enantioseparation most likely occurred because of competition from solvent adsorption via hydrogen bonding. Since the MOF has hydrogen bonding forces with EtOH, enantioselectivity decreased compared to when CH2Cl2 was used as the mobile phase. It should also be noted that the enantiomeric separation of substituted benzhydrols (42–44) occurred more efficiently for the para-substituted derivatives than for the meta- and ortho-substituted derivatives.
Table 2 Chromatographic data for the resolution of sec-alcohols
Compound |
Eluent Ia |
Eluent IIIb |
Eluent IVc |
α |
Rs |
α |
Rs |
α |
Rs |
Hexane/EtOH = 50/50. Hexane/i-PrOH = 99/1. Hexane/CH2Cl2 = 10/90. |
26 |
1.00 |
— |
1.31 |
0.72 |
1.00 |
— |
27 |
1.00 |
— |
1.95 |
1.38 |
1.28 |
0.37 |
28 |
1.00 |
— |
1.44 |
0.72 |
1.30 |
0.32 |
29 |
1.00 |
— |
1.17 |
0.31 |
1.92 |
0.52 |
30 |
1.00 |
— |
1.00 |
— |
1.83 |
0.75 |
31 |
1.00 |
— |
1.36 |
0.48 |
1.57 |
0.53 |
32 |
1.00 |
— |
1.00 |
— |
1.00 |
— |
33 |
1.00 |
— |
1.00 |
— |
2.17 |
0.93 |
34 |
1.00 |
— |
1.15 |
0.31 |
1.00 |
— |
35 |
1.00 |
— |
1.00 |
— |
1.14 |
0.26 |
36 |
1.00 |
— |
1.00 |
— |
1.34 |
0.36 |
37 |
1.00 |
— |
1.27 |
0.56 |
1.00 |
— |
38 |
1.00 |
— |
1.00 |
— |
1.86 |
0.93 |
39 |
1.00 |
— |
1.60 |
0.59 |
1.89 |
0.98 |
40 |
1.00 |
— |
2.09 |
0.70 |
1.00 |
— |
41 |
1.00 |
— |
1.28 |
0.55 |
1.81 |
0.47 |
42 |
1.00 |
— |
1.22 |
0.33 |
1.00 |
— |
43 |
1.00 |
— |
1.29 |
0.44 |
1.37 |
0.28 |
44 |
1.00 |
— |
1.42 |
0.78 |
2.44 |
0.62 |
45 |
1.00 |
— |
1.00 |
— |
2.44 |
0.70 |
46 |
1.00 |
— |
1.00 |
— |
1.74 |
0.83 |
47 |
1.21 |
0.17 |
1.00 |
— |
1.77 |
0.44 |
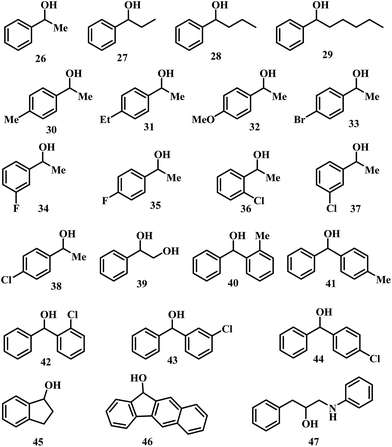 |
| Fig. 7 Chemical structures of selected sec-alcohols. | |
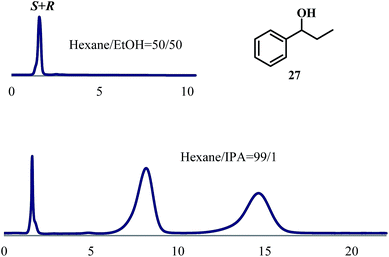 |
| Fig. 8 Chromatogram for the resolution of 1-phenyl-1-propanol 27. | |
Table 3 presents the α and Rs values for the resolution of some pharmaceutically important lactams (48–54) (Fig. 9) and amides (55 and 56) (Fig. 9) using different mobile phases (I: hexane/EtOH = 50/50 and II: hexane/i-PrOH = 90/10). As can be seen from Table 3, almost all lactams and amides, except 48 and 54, were resolved very efficiently using hexane/EtOH (50/50) as the mobile phase. In this case, enantiomeric separation of substituted β-lactams (50–52) occurred more efficiently for the para-substituted derivatives than for the meta- and ortho-substituted derivatives. For example, the α values for o-chloro-β-lactam (50), m-chloro-β-lactam (51) and p-chloro-β-lactam (52) were 1.42, 2.03 and 5.01, respectively, as shown in Fig. 10.
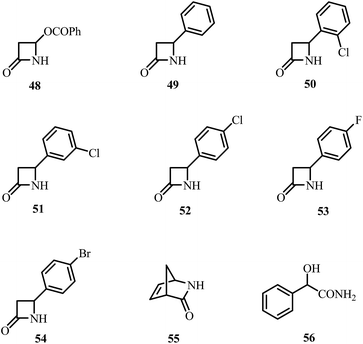 |
| Fig. 9 Chemical structures of selected amide compounds. | |
Table 3 Chromatographic data for the resolution of amide and lactams
Compound |
Eluent Ia |
Eluent IIb |
α |
Rs |
α |
Rs |
Hexane/EtOH = 50/50. Hexane/i-PrOH = 90/10. |
48 |
2.18 |
1.33 |
2.96 |
1.64 |
49 |
1.50 |
0.88 |
1.11 |
0.33 |
50 |
1.42 |
0.33 |
1.00 |
— |
51 |
2.03 |
0.61 |
1.00 |
— |
52 |
5.01 |
1.39 |
3.54 |
1.18 |
53 |
1.51 |
0.87 |
1.27 |
0.28 |
54 |
5.37 |
1.38 |
5.56 |
1.28 |
55 |
1.86 |
0.99 |
1.00 |
— |
56 |
3.38 |
0.73 |
1.74 |
0.71 |
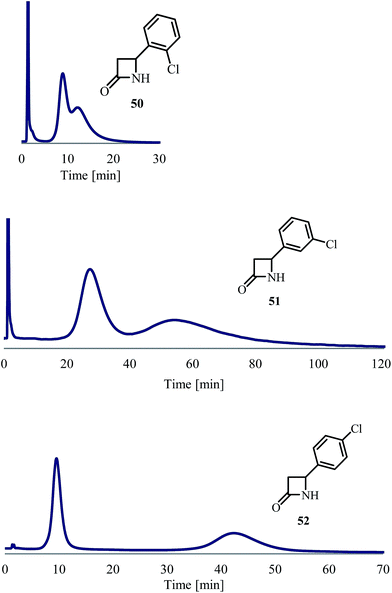 |
| Fig. 10 HPLC chromatogram for the resolution of β-lactam isomers (50–52) using eluent I as the mobile phase at a flow rate of 1.0 mL min−1 with a UV detector at 254 nm. | |
Some benzoin derivatives (57–62) (Fig. 11) were resolved using mobile phases I (hexane/EtOH = 50/50), III (hexane/i-PrOH = 99/1) and IV (hexane/CH2Cl2 = 10/90) (Table 4). For example, compounds 57–59 were separated using hexane/EtOH (50/50) as the mobile phase, while hexane/i-PrOH (99/1) was considered to be more suitable for compounds 61 and 62. The hydroxyl group of benzoin (57) may be important for the chiral recognition because compounds 60 and 61 could not be resolved under these conditions.
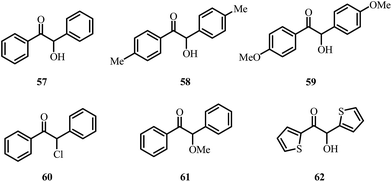 |
| Fig. 11 Chemical structures of selected benzoin. | |
Table 4 Chromatographic data for the resolution of benzoin derivatives
Compound |
Eluent Ia |
Eluent IIIb |
Eluent IVc |
α |
Rs |
α |
Rs |
α |
Rs |
Hexane/EtOH = 50/50. Hexane/i-PrOH = 99/1. Hexane/CH2Cl2 = 10/90. |
57 |
2.06 |
0.67 |
1.97 |
0.62 |
1.32 |
0.25 |
58 |
2.23 |
0.34 |
2.00 |
0.41 |
1.00 |
— |
59 |
2.10 |
0.32 |
1.00 |
— |
1.00 |
— |
60 |
1.00 |
— |
1.00 |
— |
1.00 |
— |
61 |
1.00 |
— |
1.60 |
0.32 |
1.00 |
— |
62 |
1.00 |
— |
1.36 |
0.73 |
1.00 |
— |
Some remarkable substituent effects were observed in the resolution of flavanone derivatives using hexane/CH2Cl2 = 10/90 as the mobile phase (Table 5). Interestingly, introduction of electron-withdrawing substituents on the aromatic ring (65 and 67) improved enantioselectivity, while the introduction of electron-donating substituents (64 and 66) reduced enantioselectivity, as shown in Fig. 12.
Table 5 Chromatographic data for the resolution of flavanonea
Compound |
α |
Rs |
Eluent: hexane/CH2Cl2 = 10/90. |
63 |
1.78 |
0.70 |
64 |
1.39 |
0.36 |
65 |
2.77 |
0.88 |
66 |
1.14 |
0.11 |
67 |
2.38 |
0.86 |
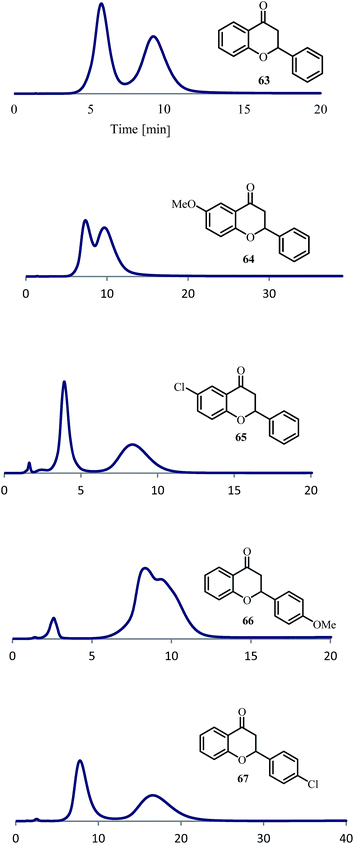 |
| Fig. 12 HPLC chromatogram for the resolution of flavanone derivatives (63–67) using eluent IV as the mobile phase at a flow rate of 1.0 mL min−1 with a UV detector at 254 nm. | |
The polarity of the mobile phase was found to play a significant role in the resolution of trans-stilbene oxide 68, as shown in Table 6. The hexane/EtOH (50
:
50) mobile phase gave poor resolution. Increased EtOH content in the mobile phase however, allowed for improved separation of the enantiomer. The results showed that the use of EtOH (100%) as the mobile phase produced excellent resolution of 68, with α value of 2.76 (Fig. 13).
Table 6 Chromatographic data for the resolution of trans-stilbene oxide
Eluent |
α |
Rs |
Hexane/EtOH = 50/50 |
1.32 |
0.19 |
Hexane/EtOH = 30/70 |
1.63 |
0.31 |
Hexane/EtOH = 10/90 |
1.85 |
0.58 |
Hexane/EtOH = 0/100 |
2.76 |
1.03 |
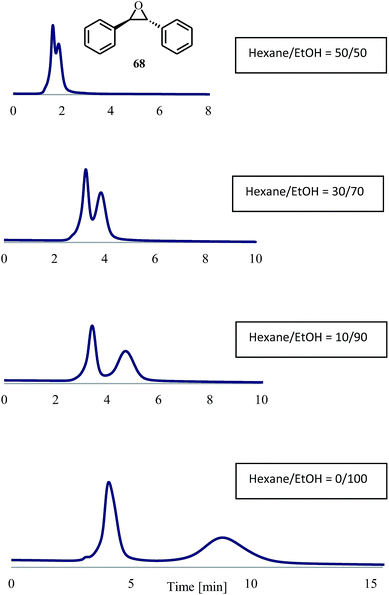 |
| Fig. 13 HPLC chromatogram for the resolution of trans-stilbene oxide 68 with different solvent systems as eluent at a flow rate of 1.0 mL min−1 with a UV detector at 254 nm. | |
Chromatographic enantiomeric separation on (R)-ZnMOF-1 and (R)-CuMOF-2
Eleven sulfoxides (3–7, 9–11, 14, 17 and 18) were separated using a (R)-ZnMOF-1 column (hexane/i-PrOH = 90/10 as eluent) and the α values for sulfoxides 6, 7, 9, 11 and 14 were higher than those obtained using the (R)-CuMOF-1 column, as shown in Table 7. Conversely, the (R)-CuMOF-2 column did not show any resolution, except for sulfoxide 4. This may be from the lack of hydrogen bonding interactions between guest molecules and (R)-CuMOF-2, which has no hydroxyl group. For the enantiomeric separation of sec-alcohols, unsuccessful results were obtained for the (R)-ZnMOF-1 and (R)-CuMOF-2 columns (Table 8). For the resolution of lactams (48–54), (R)-ZnMOF-1 had higher α values for compounds 49 and 51 than those for (R)-CuMOF-1. (R)-CuMOF-2 showed poor enantioselectivity, except for the β-lactam 48 (Table 9).
Table 7 Chromatographic data for the resolution of sulfoxidesa
Compound |
(R)-CuMOF-1 |
(R)-ZnMOF-1 |
(R)-CuMOF-2 |
α |
Rs |
α |
Rs |
α |
Rs |
Hexane/i-PrOH = 90/10. |
3 |
3.99 |
1.33 |
1.93 |
0.54 |
1.00 |
— |
4 |
2.80 |
0.84 |
1.90 |
0.64 |
1.45 |
0.11 |
5 |
2.39 |
0.87 |
2.19 |
0.46 |
1.00 |
— |
6 |
1.47 |
0.65 |
3.17 |
0.75 |
1.00 |
— |
7 |
2.16 |
1.01 |
2.45 |
0.77 |
1.00 |
— |
8 |
1.31 |
0.36 |
1.00 |
— |
1.00 |
— |
9 |
1.43 |
0.78 |
2.15 |
0.59 |
1.00 |
— |
10 |
1.63 |
1.07 |
1.47 |
0.43 |
1.00 |
— |
11 |
1.40 |
0.33 |
4.13 |
0.54 |
1.00 |
— |
12 |
1.09 |
0.06 |
1.00 |
— |
1.00 |
— |
13 |
1.95 |
1.04 |
1.00 |
— |
1.00 |
— |
14 |
1.90 |
0.79 |
2.48 |
0.55 |
1.00 |
— |
15 |
1.00 |
— |
1.00 |
— |
1.00 |
— |
16 |
2.49 |
0.68 |
1.00 |
— |
1.00 |
— |
17 |
3.03 |
0.94 |
1.91 |
0.36 |
1.00 |
— |
18 |
2.01 |
0.84 |
1.63 |
0.59 |
1.00 |
— |
19 |
1.77 |
0.93 |
1.00 |
— |
1.00 |
— |
20 |
1.00 |
— |
1.00 |
— |
1.00 |
— |
21 |
2.23 |
1.36 |
1.00 |
— |
1.00 |
— |
22 |
1.20 |
0.39 |
1.00 |
— |
1.00 |
— |
23 |
1.05 |
0.18 |
1.00 |
— |
1.00 |
— |
24 |
1.27 |
0.31 |
1.00 |
— |
1.00 |
— |
Table 8 Chromatographic data for the resolution of sec-alcoholsa
Compound |
(R)-CuMOF-1 |
(R)-ZnMOF-1 |
(R)-CuMOF-2 |
α |
Rs |
α |
Rs |
α |
Rs |
Hexane/i-PrOH = 99/1. |
26 |
1.31 |
0.72 |
1.00 |
— |
1.00 |
— |
27 |
1.95 |
1.38 |
1.00 |
— |
1.00 |
— |
28 |
1.44 |
0.72 |
1.00 |
— |
1.00 |
— |
29 |
1.17 |
0.31 |
1.00 |
— |
1.00 |
— |
30 |
1.00 |
— |
1.00 |
— |
1.00 |
— |
31 |
1.36 |
0.48 |
1.00 |
— |
1.00 |
— |
32 |
1.00 |
— |
1.00 |
— |
1.00 |
— |
33 |
1.00 |
— |
1.00 |
— |
1.00 |
— |
34 |
1.15 |
0.31 |
1.00 |
— |
1.00 |
— |
35 |
1.00 |
— |
1.00 |
— |
1.00 |
— |
36 |
1.00 |
— |
1.00 |
— |
1.00 |
— |
37 |
1.27 |
0.56 |
1.00 |
— |
1.00 |
— |
38 |
1.00 |
— |
1.00 |
— |
1.00 |
— |
39 |
1.60 |
0.59 |
1.00 |
— |
1.00 |
— |
Table 9 Chromatographic data for the resolution of lactamsa
Compound |
(R)-CuMOF-1 |
(R)-ZnMOF-1 |
(R)-CuMOF-2 |
α |
Rs |
α |
Rs |
α |
Rs |
Hexane/i-PrOH = 90/10. |
48 |
2.96 |
1.64 |
1.00 |
— |
1.74 |
0.14 |
49 |
1.11 |
0.33 |
1.24 |
0.15 |
1.00 |
— |
50 |
1.00 |
— |
1.00 |
— |
1.00 |
— |
51 |
1.00 |
— |
1.23 |
0.25 |
1.00 |
— |
52 |
3.54 |
1.18 |
1.00 |
— |
1.00 |
— |
53 |
1.27 |
0.28 |
1.00 |
— |
1.00 |
— |
54 |
5.56 |
1.28 |
1.00 |
— |
1.00 |
— |
Finally, we tested the reproducibility of the results and the reuse of the chiral MOF columns. Fig. 14 shows the HPLC chromatograms of sulfoxide 3 measured on Dec. 2011 (a) and that measured on Jan. 2016 (b) on the same (R)-CuMOF-1 column, showing both excellent reproducibility and good reusability of (R)-CuMOF-1 for the HPLC chiral stationary phase. On the other hand, (R)-ZnMOF-1 column is less stable than (R)-CuMOF-1 column since the structural integrity of (R)-ZnMOF-1 has been lost in the last 1 and a half year (Fig. 15).
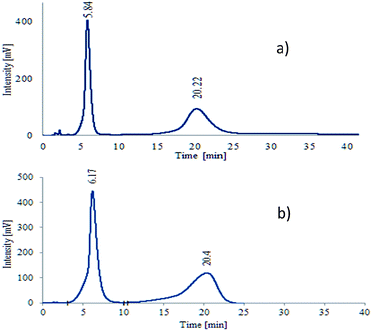 |
| Fig. 14 (a) HPLC chromatogram of sulfoxide 3 measured on Dec. 2011 and (b) that measured on Jan. 2016 on the same (R)-CuMOF-1 column. | |
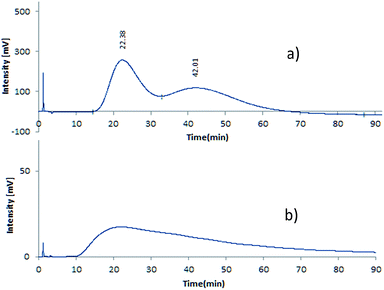 |
| Fig. 15 (a) HPLC chromatogram of sulfoxide 3 measured on Sep. 2014 and (b) that measured on Jan. 2016 on the same (R)-ZnMOF-1 column. | |
Conclusions
We have developed homochiral MOFs as a promising chiral stationary phase for HPLC enantioseparation. Columns packed with (R)-CuMOF-1 and (R)-ZnMOF-1 showed excellent chiral recognition towards many kinds of enantiomers, such as sulfoxides, sec-alcohols, β-lactams, benzoins, flavanones and epoxides. The results indicated that convenient and selective enantioseparations using homochiral MOF columns is practical for HPLC. The results are promising for exploring the potential of homochiral MOFs as novel chiral stationary phases for HPLC enantiomeric separation in the future. These species may also open a gateway to a new area in chiral separation science.
Acknowledgements
This study was supported by a Grant-in-Aid for Scientific Research (C) (No. 26410126) from The Ministry of Education, Culture, Sports, Science and Technology (MEXT). The authors are grateful to Prof. H. Tsue at Kyoto University for the BET measurement.
Notes and references
-
(a) M. Eddaoudi, D. B. Moler, H. Li, B. Chen, T. M. Reineke, M. O'Keeffe and O. M. Yaghi, Acc. Chem. Res., 2001, 34, 319–330 CrossRef CAS PubMed;
(b) G. Ferey, C. Mellot-Draznieks, C. Serre and F. Millange, Acc. Chem. Res., 2005, 38, 217–225 CrossRef CAS PubMed;
(c) R. J. Hill, D. L. Long, N. R. Champness, P. Hubberstey and M. Schroder, Acc. Chem. Res., 2005, 38, 335–348 CrossRef CAS PubMed;
(d) Z. Wang, G. Chen and K. Ding, Chem. Rev., 2009, 109, 322–359 CrossRef CAS PubMed;
(e) J. P. John, J. Perman and M. J. Zaworotko, Chem. Soc. Rev., 2009, 38, 1400–1417 RSC;
(f) W. L. Leong and J. J. Vittal, Chem. Rev., 2011, 111, 688–764 CrossRef CAS PubMed;
(g) D. Zhao, D. J. Timmons, D. Yuan and H. Zhou, Acc. Chem. Res., 2011, 44, 123–133 CrossRef CAS PubMed;
(h) T. R. Cook, Y. Zheng and P. J. Stang, Chem. Rev., 2013, 113, 734–777 CrossRef CAS PubMed.
-
(a) K. S. Suslick, P. Bhyrappa, J. H. Chou, M. E. Kosal, S. Nakagaki, D. W. Smithenry and S. R. Wilson, Acc. Chem. Res., 2005, 38, 283–291 CrossRef CAS PubMed;
(b) L. J. Murray, M. Dinca and J. R. Long, Chem. Soc. Rev., 2009, 38, 1294–1314 RSC;
(c) A. Phan, C. J. Doonan, F. J. Uribe-Romo, C. B. Knobler, M. O'Keeffe and O. M. Yaghi, Acc. Chem. Res., 2010, 43, 58–67 CrossRef CAS PubMed;
(d) K. Sumida, D. L. Rogow, J. A. Mason, T. M. McDonald, E. D. Bloch, Z. R. Herm, T. Bae and J. R. Long, Chem. Rev., 2012, 112, 724–781 CrossRef CAS PubMed;
(e) B. Van de Voorde, B. Bueken, J. Denayer and D. De Vos, Chem. Soc. Rev., 2014, 43, 5766–5788 RSC.
-
(a) J. Y. Lee, O. K. Farha, J. Roberts, K. A. Scheidt, B. T. Nguyen and J. T. Hupp, Chem. Soc. Rev., 2009, 38, 1450–1459 RSC;
(b) L. Ma and W. Lin, Top. Curr. Chem., 2010, 293, 175–205 CrossRef CAS PubMed;
(c) M. Yoon, R. Srirambalaji and K. Kim, Chem. Rev., 2012, 112, 1196–1231 CrossRef CAS PubMed;
(d) J. M. Falkowski, S. Liu and W. Lin, RSC Catal. Ser., 2013, 12, 344–364 CAS;
(e) A. Dhakshinamoorthy, M. Opanasenko, J. Cejka and H. Garcia, Catal.: Sci. Technol., 2013, 3, 2509–2540 RSC;
(f) J. Liu, L. Chen, H. Cui, J. Zhang, L. Zhang and C. Su, Chem. Soc. Rev., 2014, 43, 6011–6061 RSC.
-
(a) L. E. Kreno, K. Leong, O. K. Farha, M. Allendorf, R. P. Van Duyne and J. T. Hupp, Chem. Rev., 2012, 112, 1105–1125 CrossRef CAS PubMed;
(b) D. Liu, K. Lu, C. Poon and W. Lin, Inorg. Chem., 2014, 53, 1916–1924 CrossRef CAS PubMed;
(c) L. Heinke, M. Tu, S. Wannapaiboon, R. A. Fischer and C. Woell, Microporous Mesoporous Mater., 2015, 216, 200–215 CrossRef CAS.
-
(a) Z. Gu, C. Yang, N. Chang and X. Yan, Acc. Chem. Res., 2012, 45, 734–745 CrossRef CAS PubMed;
(b) P. Peluso, V. Mamane and S. Cossu, J. Chromatogr. A, 2014, 1363, 11–26 CrossRef CAS PubMed.
-
(a) A. L. Nuzhdin, D. N. Dybtsev, K. P. Bryliakov, E. P. Talsi and V. P. Fedin, J. Am. Chem. Soc., 2007, 129, 12958–12959 CrossRef CAS PubMed;
(b) M. Padmanaban, P. Mueller, C. Lieder, K. Gedrich, R. Gruenker, V. Bon, I. Senkovska, S. Baumgaertner, S. Opelt, S. Paasch, E. Brunner, F. Glorius, E. Klemm and S. Kaskel, Chem. Commun., 2011, 47, 12089–12091 RSC;
(c) K. Tanaka, T. Muraoka, D. Hirayama and A. Ohnishi, Chem. Commun., 2012, 48, 8577–8579 RSC;
(d) M. Zhang, Z. Pu, X. Chen, X. Gong, A. Zhu and L. Yuan, Chem. Commun., 2013, 49, 5201–5203 RSC;
(e) X. Kuang, Y. Ma, H. Su, J. Zhang, Y. Dong and B. Tang, Anal. Chem., 2014, 86, 1277–1281 CrossRef CAS PubMed;
(f) Y. Peng, T. Gong, K. Zhang, X. Lin, Y. Liu, J. Jiang and Y. Cui, Nat. Commun., 2014, 5, 4406 CAS.
- Y. Cui, H. L. Ngo, P. S. White and W. Lin, Chem. Commun., 2003, 994–998 RSC.
Footnote |
† Electronic supplementary information (ESI) available: General experimental, synthesis and characterization of ligands and homochiral MOFs, details for single crystal XRD structure refinement. CCDC 1427405 and 1427406. For ESI and crystallographic data in CIF or other electronic format see DOI: 10.1039/c5ra26520g |
|
This journal is © The Royal Society of Chemistry 2016 |
Click here to see how this site uses Cookies. View our privacy policy here.