DOI:
10.1039/D3NR01755A
(Minireview)
Nanoscale, 2023,
15, 13546-13560
Heterostructures of MXenes and transition metal oxides for supercapacitors: an overview
Received
15th April 2023
, Accepted 17th July 2023
First published on 8th August 2023
Abstract
MXenes are a large family of two dimensional (2D) materials with high conductivity, redox activity and compositional diversity that have become front-runners in the materials world for a diverse range of energy storage applications. High-performing supercapacitors require electrode materials with high charge storage capabilities, excellent electrical conductivity for fast electron transfer, and the ability of fast charging/discharging with good cyclability. While MXenes show many of these properties, their energy storage capability is limited by a narrow electrochemically stable potential window due to irreversible oxidation under anodic potentials. Although transition metal oxides (TMOs) are often high-capacity materials with high redox activity, their cyclability and poor rate performance are persistent challenges because of their dissolution in aqueous electrolytes and mediocre conductivity. Forming heterostructures of MXenes with TMOs and using hybrid electrodes is a feasible approach to simultaneously increase the charge storage capacity of MXenes and improve the cyclability and rate performance of oxides. MXenes could also act as conductive substrates for the growth of oxides, which could perform as spacers to stop the aggregation of MXene sheets during charging/discharging and help in improving the supercapacitor performance. Moreover, TMOs could increase the interfacial contact between MXene sheets and help in providing short-diffusion ion channels. Hence, MXene/TMO heterostructures are promising for energy storage. This review summarizes the most recent developments in MXene/oxide heterostructures for supercapacitors and highlights the roles of individual components.
1. Introduction
Due to the exhaustion of fossil fuels and subsequent environmental issues, high efficiency energy storage technologies are urgently needed.1–3 Rechargeable batteries and electrochemical capacitors are considered to be the future of storage technologies.4–10 Although rechargeable batteries show higher energy densities, they are limited by a low power density and a small cycle life.11–19 Widespread interest has been developed in supercapacitors due to their superior power density, high cyclability, and high rate charge storage.20–25
Supercapacitors, also referred to as ultra-capacitors, are generally categorized into double-layer capacitors and pseudocapacitors on the basis of their elementary charge storage mechanism (Fig. 1).26 Double-layer capacitors store charge electrostatically by physically adsorbing ions, whereas reversible faradic redox reactions proceed at the surface of electrode materials in pseudocapacitors to store the charge. Hence, a larger amount of charge can be stored in pseudocapacitors, and their energy density is comparatively high.26,27
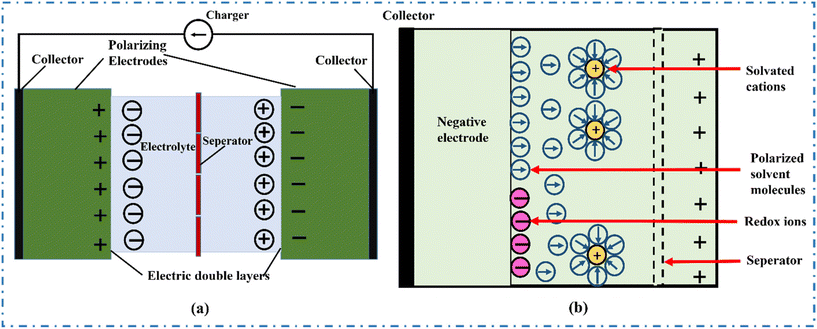 |
| Fig. 1 Mechanism of charge storage in (a) an EDLC (electric double layer capacitor) and (b) pseudocapacitors.26 | |
Supercapacitors are constructed with two electrodes, current collectors, an electrolyte, a separator, and other packaging materials.20–25 The electrochemical performance of supercapacitors primarily relies on the employed materials, electrolytes, and electrode architecture. Recently, 2D nanomaterials, such as graphene,28 transition metal oxides/hydroxides,29–31 transition metal dichalcogenides,32,33 and MXenes,34,35 have been massively investigated as active materials in energy storage applications. Among them, MXenes have drawn immense attention due to their distinctive characters such as larger interlayer spacing, redox activity, metallic conductivity, and long cyclability.34,36–44 MXenes are 2D transition metal carbides, nitrides, and/or carbonitrides.45–47 MXenes have a standard formula of Mn+1XnTx, where M represents an early transition metal, X signifies C and/or N, T signifies surface functional groups (e.g., –OH, –F, and –O), and n = 1, 2, 3, or 4.34,36–45 They show outstanding electrochemical properties, due to their inherently conductive carbide core and the possibility of charge transfer due to the presence of transition metals with flexible oxidation numbers.48–51 Since the discovery of Ti3C2Tx MXenes in 2011, several MXenes with unique properties have been synthesized and used as electrode materials for energy storage applications.34,36–45,52–57
Despite several suitable properties, MXene electrodes face certain issues during electrochemical testing, such as restacking of MXene sheets and oxidation. Several studies have been reported to address these issues, such as the synthesis of nanostructured MXenes,58 few-layer MXene sheets,59 porous MXene architectures,60 MXene/transition metal chalcogenides (TMCs)61 and MXene/transition metal oxide (TMO) composites.62 Particularly, TMOs have gained wide attention thanks to their high redox activity, ease of preparation, controlled shape, and functionality.63–65 Therefore, hybrid electrodes consisting of MXenes and TMOs can help in achieving high redox reactivity at higher rates with improved cycling efficiency. Furthermore, the volume expansion of TMOs can be minimized and the tendency of MXene sheets to restack can be reduced in these hybrid electrodes.66–68 Several heterostructures of MXenes and TMOs have been documented through hydrothermal reactions,69–71 electrostatic self-assembly,35,72 and in situ progression,73,74 showing unique arrangements and morphologies. Moreover, MXene surface terminations could be systematically directed to reduce the irreversible capacity since the electrochemical properties of MXenes greatly depend on the surface chemistry.48–51,75–80 This minireview systematically focuses on the formation strategies of such heterostructures and highlights the aspects governing their improved energy storage capability.
2. Synthesis methodologies of MXenes and their heterostructures with TMOs
TMOs can be easily integrated with MXenes using various approaches. The physical and chemical characteristics of the formed heterostructures are directly influenced by their synthesis method. Below, some common methods have been discussed.
2.1 Hydrothermal method
The advantages of the hydrothermal method include high product crystallinity, low operating temperatures, and high diffusion rates. Here, MXenes are dispersed in a liquid phase with a different material under extreme conditions of pressure and heat. Moreover, the functional groups can be controlled on the surface of MXene-based heterostructures, further advancing their activity. In 2018, Yu and coworkers obtained a novel 2D/2D heterojunction of ultrathin Ti3C2/Bi2WO6 nanosheets through the in situ growth of Bi2WO6 on the surface of Ti3C2 (Fig. 2a–c).69 For this process, few-layer ultrathin Ti3C2 nanosheets were acquired through the ultrasonic exfoliation of multilayered MXenes (Fig. 2a). The negative potential of Ti3C2 makes Bi3+ ions easily adsorbed on their surface, which results in a close interface between Ti3C2 and Bi2WO6. The growth of Bi2WO6 on the Ti3C2 surface is ensured by the electrostatic attraction between Bi3+ cations and Ti3C2, which leads to strong contact between Ti3C2 and Bi2WO6. The constructed heterostructure composite is fashioned with abundant atomic layers, a large interface contact area, and a quite small charge transport distance, which potentially improved the photocatalytic efficiency of the heterostructure by 6 times as compared to pristine Bi2WO6 nanosheets. In 2019, an innovative ultrafast kinetics net electrode was constructed through a MoSe2/MXene heterojunction by Jiang and coworkers using a straightforward hydrothermal process and the thermal annealing method.70 The prepared heterojunction moderated the volume expansion, improved the low electronic conductivity and polyselenide shuttle effect of MoSe2. In addition to dramatically enhancing the reaction kinetics, the force of van der Waals contact among MoSe2 and MXenes successfully capped the volume shift throughout sodium ion insertion/extraction cycles. The MoSe2/Ti3C2 heterojunctions acquired a 3D network with rich porous structure, demonstrating a potential anode with a reversible capacity of 434 mA h g−1 for 200 cycles at 1 A g−1. However, MXenes can undergo oxidation by dissolved oxygen in aqueous solutions under hydrothermal conditions.
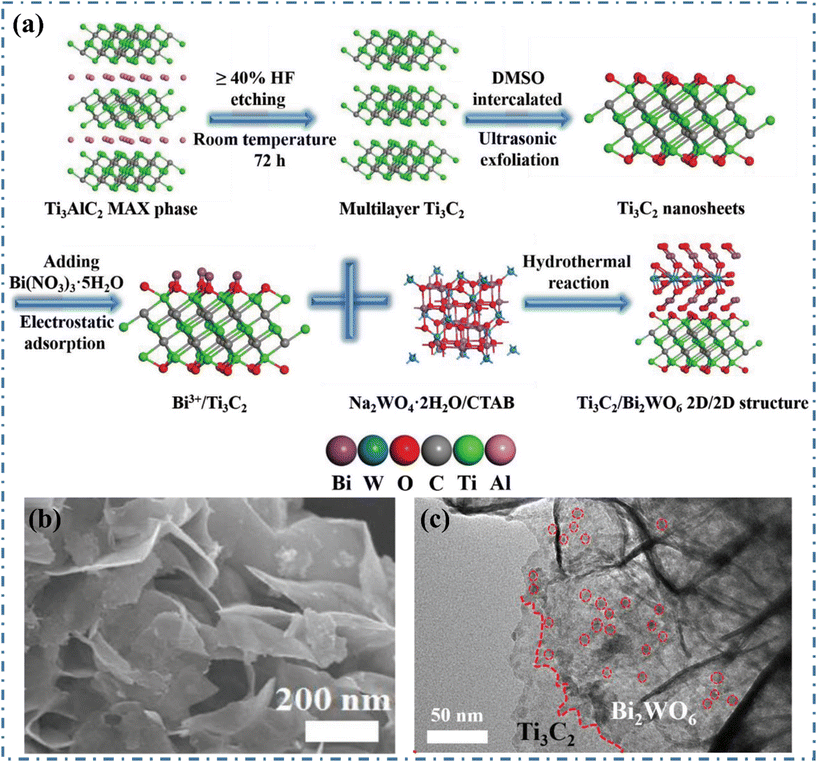 |
| Fig. 2 (a) Schematic demonstration of the synthesis of ultrathin Ti3C2/Bi2WO6 nanosheets (2D/2D heterojunction). (b and c) FESEM and TEM images of Ti3C2/Bi2WO6.69 Reproduced from ref. 69 with permission from Elsevier, copyright 2020. | |
2.2 Self-assembly strategy
Self-assembly has grown to be the most advanced bottom-up strategy for building nanomaterials in terms of their size and morphology. This strategy is easy to implement, economical, extremely effective, and outcomes in metal-oxide nanostructures packed tightly on MXene nanosheets. Here, metal oxides (MOs) self-assemble on MXenes (Ti3C2) through electrostatic interactions, or van der Waals interactions, consequently reducing the surface energy and stabilizing the structure. The potential of individual components is adequately assimilated in the subsequent MO/MXene heterostructures. Correspondingly, MXene nanosheets serve as a substrate for circumventing the aggregation of TMO nanostructures. Generally, their structures and electrochemical properties are significantly influenced by regulating the proportions of MXenes and the complementary materials. In 2018, Xu and coworkers reported van der Waals interaction-based facile self-assembled nanorods and nanowires of TiO2 and SnO2 on MXenes (Ti3C2) (Fig. 3a–e).35 As a substrate, MXene nanosheets were utilized, allowing reversible transportation of electrons and ions at the interface and inhibiting the TMO nanostructures from clumping together. Consequently, TMO nanostructures act as a spacer to block MXene nanosheets from stacking, thus retaining the active sites. MXene nanosheets with outstanding mechanical flexibility improve the pulverization of SnO2 nanowires, which are known for experiencing extreme volume expansion. In 2021, the same group proposed another ideal and compact interfacial arrangement of Fe3O4 and MXenes as a result of the self-assembly process facilitated by van der Waals interactions.66 The route permits a uniform dispersion of Fe3O4 nanodots over MXene nanosheets. In 2023, Guang-Sheng Wang reported a versatile method for the synthesis of Co-based bimetallic oxide heterostructures with a flower shaped morphology, which can be used to build a 3D crossing network structure via the electrostatic self-assembly of MXene nanosheets.72 The built network is practicable for constructing conductive pathways, facilitating the unrestricted movement of electrons. The obtained bimetallic MXene composite demonstrated excellent microwave absorption capacity with a minimum reflection loss. Unlike hydrothermal synthesis, this self-assembly approach does not result in structural deterioration of MXene nanosheets.
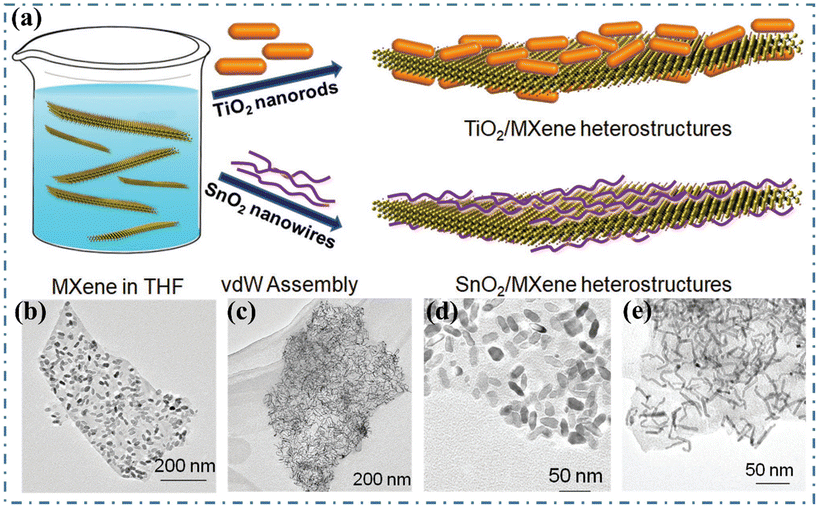 |
| Fig. 3 (a) Schematic illustration for the self-assembly of SnO2 nanowires and TiO2 nanorods on the nanosheets of MXenes in THF via van der Waals contacts. (b and c) Pictorial representation of the TEM images of TiO2/MXene heterostructures at various magnification scales. (d and e) TEM images of SnO2/MXene heterostructures at various magnification scales.35 Reproduced from ref. 35 with permission from Wiley-VCH, copyright 2018. | |
2.3 Chemical deposition strategy
With chemical liquid phase deposition, specific coatings can be deposited on the target material via controlled chemical reactions. This technique can be used to peel away the inner layers of multilayered MXenes or to coat MXene nanosheets with nanoparticles to avoid restacking. Unnecessary oxidation of MXenes could be avoided here as this method is performed under mild reaction conditions (relatively low pressure and temperature). In 2022, a porous hydrogel with a 3D hierarchical Ti3C2TX@NiO–rGO heterostructure was prepared by Haijun Xu, and it demonstrated excellent mechanical properties and conductivity.81 To synthesize the heterostructure, chemical bath deposition and thermal annealing methods were used, followed by a simple GO-assisted self-convergence hydrothermal process. The heterostructure displayed a 2D arrangement inherently consisting of a thin, wrinkled, and paper-like composition. A large electroactive surface and rapid ion/electron transport channels were achieved by preparing a 3D mesoporous architecture that efficiently constrained the aggregation of the Ti3C2Tx@NiO heterostructure. Li et al. synthesized RuO2 nanoparticles that are evenly attached to MXene nanosheets.47 During the reaction, negatively charged MXene surfaces were electrostatically self-attracted onto positively charged Ru3+ surfaces prior to the oxidation of Ru cations, which efficiently hampers the restacking of MXene nanosheets. The increased specific surface area of the mesoporous structure of RuO2·xH2O@MXenes might have hastened the electrolyte ion migration and diffusion, further enhancing the electrochemical performance.
2.4 High temperature in situ derivation strategy
By heating MXenes with or without additional phases, high-temperature in situ derivation is a strategy for creating a second phase on the surface of MXenes.73 For example, Yang et al. established an in situ growth of an accordion-like TiO2/Ti3C2 composite and employed it as an anode material for LIBs (lithium-ion batteries) and SIBs (sodium-ion batteries).73 MXene heterostructures are formed as a result of oxidation, sulfidation, or a phase transition that occurs during the heat treatment. One example is the single-step oxidation of 2D Ti3C2 or Nb2C powders at 1150 °C for 30 s to produce thin sheets of disordered graphitic carbon that are embellished with oxide nanocrystals of anatase or niobia, Nb2O5 (Fig. 4a–c).74 Analogous structures were acquired by oxidizing Ti3C2 in CO2 between 150 and 300 °C. Controlling the process can be challenging with flash oxidation, especially if the particles get on fire. The layers of the as-synthesized Ti3C2Tx were analogous to those of exfoliated graphite. During flash oxidation, oxide nanocrystals were shown to form at the edges and between the layers. In another example, a humidity sensor was developed by growing in situ TiO2 nanowires on a 2D Ti3C2 MXene using the alkali oxidation process, showing an urchin-like morphology.82 As compared to pure Ti3C2 or pure TiO2, the sensor's remarkable sensitivity is due to its extraordinarily high specific surface area. OH-terminated Ti3C2 sheets are regarded as metallic due to their extremely low band gap and excellent carrier mobility (Fig. 4d–f).82
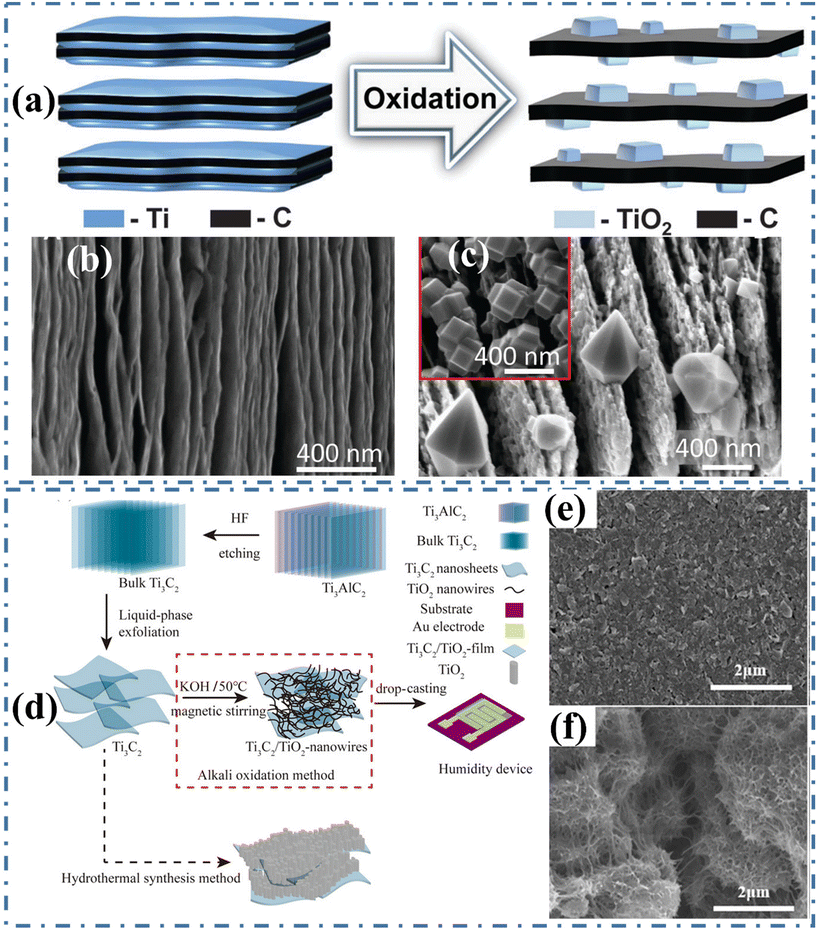 |
| Fig. 4 (a) Diagram showing the oxidation of MXenes, and the creation of a hybrid structure made of graphitic and disordered carbon. (b and c) TEM pictures of Ti3C2Tx powders before and after 30 s of flash oxidation in air at 1150 °C.74 (d) Processes used to prepare the materials including HF etching, LPE, alkali oxidation, and others. SEM images of the (e) initially synthesized 2D-Ti3C2 MXene nanosheets and (f) Ti3C2/TiO2 composites that resemble sea urchins.82 Reproduced from ref. 74 with permission from RSC, copyright 2014; ref. 82 with permission from ACS, copyright 2019. | |
3. Heterostructures of MXenes/TMOs for supercapacitors
Heterostructures of MXenes/TMOs are promising electrode materials in energy storage devices, particularly supercapacitors, which are gaining much attention due to their high power density and high cyclability.83 In MXene/TMO heterostructures, TMOs prevent the restacking of MXene sheets, while the MXene layers might prevent the accumulation of TMOs and disseminate charge and ion transport thanks to their high electrical conductivity. TMO nanostructures may also serve as spacers to separate the MXene layer, improving interfacial connections and enriching the active sites, leading to a high cyclability of the heterostructures. MXene nanosheets can also serve as conducting substrates for the growth of TMO nanoarchitectures, which helps in enhancing electron transportation.84 It should be noted that MXene/TMO interfacial bonding can be significantly influenced by the morphological, structural, and surface-controlled functional groups of MXenes and TMOs together.35,74,81 Thus, MXene/TMO heterostructures are promising electrode materials for supercapacitors due to the synergistic interaction between conductive MXenes and high capacity TMOs.85,86
TMOs such as non-layered metal oxides, binary transition metal oxides (BMOs), e.g., MnO2, SnO2, NiMoO4, ZnCo2O4, NiCoO4, etc., are the most suitable choice with MXenes for their high redox capacity.87–89 When combined with MXenes, manganese oxides (MnO2) are one of the most extensively researched among all oxides because of their high theoretical specific capacitance value, low toxicity, low cost, and ease of fabrication.62,90–93 In addition, MnO2 has a fast redox reaction for improved charge storage ability compared to other materials due to its varying oxidation states from Mn3+ to Mn4+. It exists in six crystal phases, which produce a wide range of distinct crystal shapes.76 In addition to having a high theoretical capacitance of 1370 F g−1 and a wide electrochemical window of 0–0.9 V, MnO2 can also function in mild aqueous electrolytes, leading to less chemical corrosion of the current collector and structural breakdown.35,74,81 Thus, MnO2 and MXene hybrids with various morphologies have been extensively studied to produce supercapacitors demonstrating high performance. For example, in 2022 Meng et al. explored a heterojunction of MnO2 nano lamellas (NL) on Ti3C2Tx MXene nanosheets (MnO2 NL/MX) by maintaining the reaction conditions in terms of morphological and structural compositions. The associated mechanisms are as follows: (i) the heterostructure of MnO2 NL/MX prevents aggregation and restacking that lead to an increase in the active sites and (ii) direct face-to-face contact guarantees robust electrical interactions between MnO2 and Ti3C2Tx, which could improve their inherent electrochemical properties and achieve a specific capacitance of 334.3 F g−1 at 0.5 A g−1 with a cycling retention of 90% after 5000 successive cycles.62 In addition, various other heterostructures of MnO2 with MXenes have also been explored for supercapacitors, e.g., ε-MnO2 nanowhiskers with Ti3C2Txvia direct chemical synthesis methods that lead to enhanced supercapacitor performance. MnO2 nanowhiskers in the MXene framework improve the overall cycling performance by 88% after 10
000 cycles by increasing the electrolyte-accessible surface area and contributing pseudocapacitance.94 While in 2022, Pan et al. constructed MXenes with a MoO3-based hybrid free standing film as the negative electrode and A-CNT/Kx–MnO2 as the positive electrode via introducing a strategy of K+ ion intercalation that increases ion/electron transport during the galvanostatic charge–discharge process and helps to operate up to 2 V of a wide operating potential window having an energy density of 36.5 W h kg−1.95 Another strategy of cation and anion intercalation/deintercalation ion mechanisms in LnMnO3(LMO)/Ti3C2Tx was observed by Tomar et al. Here, multilayer MXenes show cations, and oxygen-defective LMOs show anion intercalation. In ex situ, XPS analysis found not only K+ ions but also OH− ions intercalating into the LMO/Ti3C2Tx heterostructures, which increases the charge storage. Furthermore, achieved a specific capacitance of 442.8 F g−1 at 3 A g−1 with an energy density of 34.1 W h kg−1.96
In general, the morphologies and binding mechanisms of MXenes and TMOs are vital for enhancing the supercapacitor performance. It is crucial to ascertain how MXenes and TMOs interact, bind, store energy, transport ions, and interact with one another in order to optimize the performance of supercapacitors.27,68,97 For surface functionalization and morphological control, Mustafa et al. prepared SnO2 nanoflowers (NFs) treated with ascorbic acid, which activated the surface and served as a linkage for MXene nanosheets in the MXene/SnO2 heterostructure. SnO2 NFs served as interlayer spacers and nanopillars to stop restacking, which helped in improving the performance of supercapacitors with 643 F g−1 and only 2% degradation loss in cycling stability.98 In another report, Zhang et al. synthesized a heterostructure of Ti3C2Tx-supported Fe3O4 nanoplates (denoted as MXene–Fe) (Fig. 5a–c) via a one-step solvothermal strategy as a pseudocapacitive material. The increasing concentration of Fe ions causes hexagonal plates to form and cover the surface of Ti3C2Tx uniformly. It acts as a spacer to prevent the Ti3C2Tx nanosheets from being stacked again, ensuring there are enough electrochemically active sites. The CV profiles of the MXene–Fe-3 electrode were examined at various scan rates, showing redox peaks indicating the pseudocapacitive feature that leads to determining the electrochemical kinetics and storage mechanism. It has smaller b values that lead to more diffusion-controlled behavior (Fig. 6d). At a lower scan rate of 5 mV s−1, the capacitance contribution is only 23.6% (Fig. 6e). With a decrease in diffusive contribution, the capacitive contribution increases with the scan rate (Fig. 6f).99 Another strategy was employed by Ashraf et al. where an ionic liquid (IL)-incorporated delaminated heterostructure, D-Ti3C2/MoO3, was constructed by the integration of imidazolium-based ILs to MoO3 nanorods that are anchored on the surface of the MXene surface. D-Ti3C2 provides structural stability and increases the hydrophilicity of heterostructures, whereas IL@MoO3 helps in enhancing the electrical conductivity and achieves a capacitance of 1680 F g−1 at 1 A g−1.100
 |
| Fig. 5 (a) Schematic illustration of the synthesis of the Ti3C2Tx/Fe3O4 heterostructure. (b and c) SEM images of the heterostructure. (d) Representation of the calculated b values of various peak currents, the inset shows the associated CV curves for the MXene-Fe-3 electrode. (e) Capacitive contribution at 5 mV s−1. (f) Capacitive–diffusive contribution at different scan rates.99 Reproduced from ref. 99 with permission from RSC, copyright 2021. | |
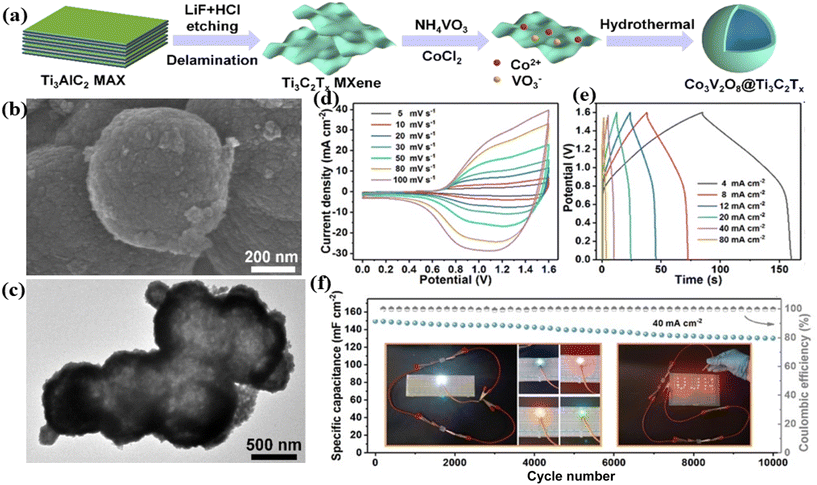 |
| Fig. 6 (a) Representation of the synthesis of a BMO/MXene heterostructure. (b and c) Corresponding SEM and TEM images. (d) CV at various scan rates. (e) GCD at various current densities. (f) Cycling stability, and the insets show photographs of the LED.104 Reproduced from ref. 104 with permission from RSC, copyright 2022. | |
Liu et al. recently prepared a novel class of W18O49/Ti3C2Tx pseudocapacitive materials. While both the electrode materials have a negative charge, the electrostatic repulsive force aids in preventing W18O49 aggregation and MXene nanosheet's restacking. The heterostructure exhibited a higher supercapacitor performance of 696.2 F g−1 at 1 A g−1 with a higher cyclic retention of 99.7% after 6000 cycles.101
3.1 Binary transition metal oxide (BMO)/MXene heterostructures
Apart from the above-mentioned transition metal oxides, some binary transition metal oxides with MXenes were also explored. Thanks to their multiple oxidation states and the ability to provide abundant active sites for faradic redox reactions, BMOs are able to deliver improved charge storage performance than their single counterparts.102,103 Zhou et al. prepared a Ti3C2Tx MXene-wrapped hollow Co3V2O8 nanosphere for a solid-state supercapacitor (Fig. 6a). Hollow nanostructures of Co3V2O8 nanospheres with an empty interior show in their corresponding SEM and TEM images that they may have enough room to support volume expansion and reduce the mechanical strain brought on by volume change during the repeated charge–discharge process (Fig. 6b and c). This leads to an increase in the areal capacitance of the asymmetric supercapacitor device, which has a higher CV area with different scan rates and a specific capacity varying from 4 to 80 mA cm−2, and at 4 mA cm−2 the specific capacity is 203.8 mA cm−2 (Fig. 6d and e). The ASC device shows a high cyclic retention of 94.5% after 10
000 successive cycles with the lighting of 25 LED bulbs (Fig. 6f).104
Another strategy of electrophoretic deposition was employed by Asen et al. to construct a RuCo2O4/Ti3C2Tx MXene@NF heterostructure. The hierarchical urchin porous structure of RuCo2O4 grown on Ti3C2Tx MXene@NF was confirmed via density functional theory studies and the heterostructure shows a remarkable capacitance of 450 F g−1 at a current density of 3 A g−1 with a high cyclic retention of 94%.105 In addition to the Ru-based BMO, NiCo, NiMo, and ZnCo, etc. were also explored with MXenes for supercapacitors. BMO heterostructure generally follows the same strategy as bare bimetallic oxides for preparing electrode materials for supercapacitors. However, in 2019, Javed et al. prepared an all-solid-state flexible ASC device based on a battery-type BMO heterostructure by incorporating ZnCo2O4 polyhedra on Ti3C2Tx, which achieved a specific capacitance of 2643.66 F g−1 at 2 A g−1 with an ultrahigh energy density of 99.94 W h kg−1.106 Furthermore, the heterostructure was able to achieve higher cycling retention. In 2019, Wang et al. reported a facile pathway to prepare hydrophilic NiMoO4/Ti3C2Tx heterostructure nanosheets having interconnected porous construction, enabling the diffusion of electrolyte ions throughout reversible insertion/deinsertion progressions. The selected MXene was made from ultrathin Ti3C2Tx flakes that were synthesized by removing Al layers from bulk Ti3AlC2, followed by LiF/HCl etching. Subsequently, the negatively charged Ti3C2Tx flake's surfaces were electrostatically attached with nickel and molybdenum ions using the hydrothermal process and post-calcination, generating the NiMoO4/Ti3C2Tx heterostructure. The greater hydrophilicity and electrical conductivity of Ti3C2Tx, as well as the synergistic interactions between NiMoO4 and Ti3C2Tx, resulted in a high capacitance of 1364 F g−1 at 0.5 A g−1. Additionally, an asymmetric supercapacitor with a large 1.6 V potential window and an energy density of 33.76 W h kg−1 was achieved by combining a reduced graphene oxide hydrogel as the negative electrode with a NiMoO4/Ti3C2Tx as the positive electrode.107
The elasticity and flexibility of MXenes can be reflected in their in-plane stiffness (C) and out-of-plane rigidity (D). The C and D of MXenes are strongly influenced by the M element type, surface functional groups, and MX thickness.17 MXenes are a type of strong yet flexible material, as evidenced by the Foppl–von Karman numbers per area (C/D), which are equivalent to those of the MoS2 monolayer.67 MXenes have D values ranging from 4 to 156 eV, which are quite higher than that of monolayer graphene (1.2 eV), logically concluding that monolayer MXenes have a much higher bending rigidity than monolayer graphene. Interestingly, the bending rigidity of three-atom-thick Ti2C and Nb2C MXenes was more flexible than that of three-atom-thick MoS2 (approximately 9.14 eV). Surface termination in Tin+1Cn (n = 1, 2, or 3) might prevent the collapse of surface atomic layers and withstand significant stresses under tensile loading, increasing MXenes’ mechanical adaptability makes them prime materials for fabricating flexible electrodes and other electronic devices.17,67 With an increase in layer thickness, MXenes become less flexible. Even though the thinnest MXenes are the most flexible, their in-plane stiffness decreases as their thickness decreases. Moreover, MSC electrodes printed with MXene structures have emerged as a promising option for the development of superior electrochemical performance.108 According to a study published in 2019, Li et al. uniformly anchored hydrous ruthenium oxide (RuO2) nanoparticles on the as-synthesized Ti3C2Tx MXene nanosheets via a screen printing process (Fig. 7). The fabricated MSCs had a better volumetric capacitance of 864.2 F cm−3 at 1 mV s−1, remarkable cycling stability (90% retention after 10
000 cycles), a virtuous rate capacity of 2000 mV s−1, and wonderful flexibility showing 87.3% retention of the initial capacitance after 2000 bending cycles.108
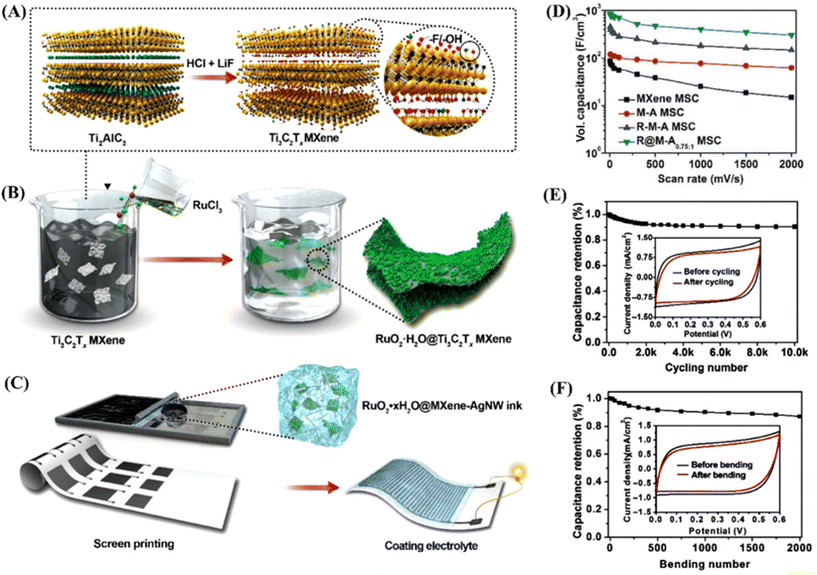 |
| Fig. 7 Illustration of (a) the preparation of Ti3C2Tx MXenes; (b) procedure for the synthesis of the RuO2·xH2O@MXene nanocomposite; (c) development of flexible MSC devices through screen printing; a graphical image showing (d) the volumetric capacitance of various MXene MSCs at diverse scan rates; (e) plot of cycling stability at 100 mV s−1 (inset: CV curves recorded before and after cycling); and (f) plot of cycling stability with a bending strain of 5.0% at various bending cycles (the inset shows CV curves recorded before and after 2000 bending cycles at 100 mV s−1).108 Reproduced from ref. 108 with permission from Wiley-VCH, copyright 2019. | |
Lithium-ion capacitors (LICs), which fully combine the advantages of LIBs and SCs, often have high power/energy densities and a long lifespan in a single device.109,110 With much greater theoretical capacities and a minimum rate of volume expansion (∼5%) during electrochemical lithiation/delithiation sequences, niobium oxide-based anodes are gaining increasing interest among all TMOs and BMOs. In 2021, for the first time, Qin et al. fabricated single-crystalline perovskite NaNbO3 nanocubes (S-P-NNO NCs) by a straightforward hydrothermal alkalization method.111 Furthermore, the resultant nanocubes were assembled with f-Nb2CTx MXenes to construct S-P-NNO/f-Nb2CTx heterostructures using a freeze-drying route. The heterostructures were given strong electronic conductivity by the conductive f-Nb2CTx MXenes, which also created a three-dimensional (3D) porous network that allowed for quick Li+ transfer inside the hybrids. Additionally, S-P-NNO NCs distributed equally on and across the nanostructures (NSs) of MXenes, might lessen their tendency to aggregate and self-stack. When used as an anode material for LICs, the produced heterostructures presented excellent pseudocapacitance-dominated capacities and high-rate characteristics because of the synergistic contributions from the S-P-NNO NCs and f-Nb2CTx NSs. The assembled asymmetric capacitor from the prepared hybrid demonstrated a high energy density of ∼241 W h kg−1 at 56 W h kg−1 with a superior power density of ∼13 kW kg−1. A noteworthy level of cycling stability was also achieved after 4000 charge–discharge cycles.
3.2 Role of electrolytes in TMO/MXene heterostructures
In the past few decades, most studies have focused on H2SO4 and less concentrated salt electrolytes for MXene-based heterostructures. In contrast to neutral aqueous electrolytes, H2SO4 is neither safe nor environmentally friendly. Further issues with acidic electrolytes are as follows: (i) because of its acidic nature, there is a possibility that the cell would fail due to corrosion and the generation of by-products, (ii) the generation of the hydrogen evolution reaction leads to a short operation potential window, and (iii) because of the usage of H2SO4 and low concentration salt electrolytes, the self-discharge rates of the cells are high. Electrolytes, for instance, gels or “water in salts” (WIS) are therefore being taken into consideration. WIS are extremely concentrated electrolytes (salt/water ratio >1) that have drawn a lot of interest because of their steady, high electrochemical stability window (>2 V), slow self-discharge rates, and the high concentration of salt.112–115
By taking these aspects into consideration recently, Saraf et al. prepared α-MoO3/Ti3C2 heterostructure-based free-standing films with different compositions via simple mixing at room temperature without using any binders. Ti3C2 MXenes provide mechanical stability and easy synthesis of free-standing films with α-MoO3. The electrochemical performance shows multiple redox peaks in a water-in-salt electrolyte (19.8 m LiCl), and a wide operating potential of 1.8 V (vs. Ag wire).116 Zheng et al. prepared Mn3O4 (+ve electrode)/Ti3C2Tz (−ve electrode) with high mass loading (10 mg cm−2) and low self-discharge rates in a 14 M LiCl electrolyte, respectively. Subsequent ASC devices showed ultrahigh energy and power density with excellent rate capability and a wide operating potential window of 1.5 V due to the usage of WIS electrolytes.117 In addition to the LiCl-based electrolytes, Zheng et al. utilized a 21 M lithium bis(trifluoromethanesulphonyl)imide (LiTFSI) electrolyte in a Mo1.3CTz MXene/MnO2 system. The tertbutyl ammonium intercalated MnO2/MXene in a very intense electrolyte shows an ultrahigh potential window of 2.5 V and high-rate capability. Another benefit of this electrolyte is slower SD rates than less concentrated electrolytes.91 Similarly, such MXene/oxide heterostructures should be explored in other electrolytes such as ionic liquids118 and organic electrolytes119 to find new electrochemical insights. Table 1 summarizes the electrochemical performance of some state-of-the-art MXene/TMO heterostructure-based supercapacitors.
Table 1 Reported state-of-the-art MXene/TMO heterostructures and their supercapacitor performance
S. no |
Electrode materials |
Specific capacitance/areal capacitance (F g−1/mF cm−2) |
Current density (A g−1/mV s−1) |
Cycling efficiency |
Ref. |
1 |
Fe2O3/Ti3C2Tx aerogel |
182 F g−1 |
1 |
81.74% after 10 000 cycles |
120
|
2 |
Fe2O3/Ti3C2Tx |
486.3 F g−1 |
1 |
95.7% after 5000 cycles |
121
|
3 |
MXene/V2O5 film |
319.1 F g−1 |
0.5 |
70.4%, after 5000 cycles |
122
|
4 |
Fe2O3/Ti3C2Tx |
584 F g−1 |
1 |
121% after 13 000 cycles |
123
|
5 |
CoOx–NiO/Ti3C2Tx |
1960 F g−1 |
1 |
90.2% after 8000 cycles |
124
|
6 |
MoO3/D-Ti3C2Tx |
545 F g−1 |
3 |
90% after 5000 cycles |
125
|
7 |
Ti3C2Tx/MoO3−x/PEDOT:PSS |
523.0 F g−1 |
1 |
95.5% after 5000 cycles |
126
|
8 |
Ti3C2Tx/NiCo-LDHs |
1207 F g−1 |
1 |
93% after 5000 cycles |
127
|
9 |
NiCo2O4/Ti3C2Tx/rGO |
1633 F g−1 |
1 |
86.6% after 10 000 cycles |
126
|
10 |
V4C3TX@NiO/rGO |
1009.5 F g−1 |
1 |
97.45% after 10 000 cycles |
128
|
11 |
MnO2/Ti3C2Tx |
130.5 F g−1 |
1 |
100% after 1000 cycles |
92
|
12 |
N–Ti3C2Tx/TiO2 |
918.69 F g−1 |
5 |
74.39% after 10 000 cycles |
129
|
13 |
Ti3C2Tx/rGO/Fe3O4 |
1250.5 mF cm−2 |
1 |
85.8% after 5000 cycles |
130
|
14 |
Ti3C2Tx/NiO/rGO |
979 F g−1 |
0.5 |
95.6% after 10 000 cycles |
131
|
15 |
MXene–MnO2–CoNi-LDHs |
922 F g−1 |
1 |
79% after 4000 cycles |
90
|
4. Theoretical aspects of MXene properties
DFT simulations have favored MXene-based materials having pronounced energy storage density, low diffusion barriers, and excellent stability for energy storage applications.71,132 Modelling and simulations have been used to determine the surface terminating groups in MXenes.71,133,134 Although the complication of assemblies and formation makes it exceedingly difficult to experimentally determine surface terminal arrangements at the atomic scale, there is still no clear correlation between MXene termination and the synthesis strategy. An effective method for determining the optimum surface structure of MXenes in this situation is a first-principles simulation, e.g., based on DFT.134,135 Ashton et al. used DFT methods to examine how the chemical configuration and hydrogen chemical potential of MXenes with various terminal groups affected their thermodynamic stability, which shows that the majority of possibilities for MXenes are theoretically synthesizable.136 Several theoretical research studies have conducted to verify the formation of terminations on the surfaces of numerous MXenes. However, the results are far from an accurate representation of the actual situation as dictated by the etching procedures since the whole intricacy of the surface only considers one or two types of T-groups in a symmetric way and more sophisticated simulation methods are still necessary for an accurate match.137
The literature has revealed that the most stable MXenes were those with
O and/or –OH terminations because the –F terminal group was easily exchanged by –OH groups, and –OH terminations were changed to –O terminals at high temperatures, and/or metal adsorption operation, which might occur when they were washed and/or kept in water.138,139 Sang et al. used STEM-EELS in a similar way to characterize single layer Ti3C2Tx (with a 7.5
:
1 LiF to Ti3AlC2 molar ratio in 6 M HCl at 35 °C for 24 hours).140 The frequency of various point defects and the significance of etchant concentration in defect production were clarified in the monolayer flakes. The effect of defects on the surface chemistry and electrical characteristics of Ti3C2Tx was theoretically investigated using ab initio molecular dynamics (AIMD) simulations, and it was shown that the conductivity of Ti3C2Tx was not affected by the defect concentration.133,137
The identification of numerous metal atoms in MXenes significantly increases the variety of potential chemical compositions and the inherent characteristics of MXenes.138 An organized Cr2TiAlC2 MAX phase structure with a Ti atom layer enclosed between outer Cr layers was first identified by Liu et al.141 Later, Anasori et al. applied DFT calculations, and it was predicted that the early transition metals M′ and M′′ would exist as an immense family of ordered carbides, M′2M′′C2 and M′2M′′2C3. When compared to the typical single-metal Ti–C-based MXenes, multiple Mo2TiC2Tx, Mo2Ti2C3Tx, and Cr2TiC2Tx exhibit different electrochemical behaviors. Due to their reduced symmetry and flexibility in selecting metal atom combinations to customize their electrical characteristics, multiple metal atom MXenes have a wider spectrum of possible features.142,143
4.1 Electronic characteristics of MXenes and their heterostructures with metal oxides
By applying DFT calculations, the band structure of terminated MXenes was found to be metallic in nature.34,45 Ti3C2Tx has a greater electrical conductance than graphene (2500 S m−1) according to experimental measurements, reaching 3250 S m−1.144,145 Khazaei et al. further demonstrated through DFT calculations with a few notable exceptions that the MXenes of metal nitrides M2N (M = Cr, Zr, Ti) and metal carbides M2C (M = V, Sc, Ti, Cr, Zr, Nb, Ta) with F, O, or OH termination are frequently metallic and non-magnetic. DFT analysis established that only Ti2C and Zr2C exhibit instinctive magnetism for the bare M2C MXene, while the other bare MXenes lack magnetic properties.146,147 In comparison with a pure metal oxide electrode, the MXene/metal oxide heterostructure offers a larger specific capacitance, greater rate capability, and more stable cycling performance due to its higher electrical conductivity and quicker electron transfer ability according to DFT calculations.48 The electronic state and electronic structure can be tuned effectively by the hybrid structure, which supports the enhancement of electrochemical performance. For example, in 2023, Zhang et al. confirmed the mechanism behind the better electrochemical performance of the NCO/MGA-300 electrode through DFT calculations48 In this research an assembly of NiCo2O4 (NCO) microtubes was laid on/into a Ti3C2Tx MXene/reduced graphene oxide aerogel (MGA). The NCO/MGA-300 electrode therefore displayed exceptional gravimetric capacitance equivalent to 1633 F g−1 at 1 A g−1, and rate performance of 1492 F g−1 at 10 A g−1. Additionally, an asymmetric supercapacitor prepared from this heterostructure exhibited consistent electrochemical performance at various compressive pressures of 20%, 40% and 60% following 100 compression–release cycles. The impact of the heterostructure on the kinetics of redox reactions was computed by the adsorption properties of OH− on the NCO (220)/MXene heterojunction and NCO (220) surfaces. Given that the heterostructure can hold more electrolyte ions due to its reduced adsorption energy (−2.314 eV), the heterostructure favors the kinetics of redox reactions on electrode surfaces. A considerable charge transfer phenomenon was confirmed by the charge density at the NCO (220)/MXene heterointerface, which showed an accumulating tendency near the surface of NCO (220). The same year, Pathak et al. established the importance of theoretical perception gained from DFT calculations for a deeper understanding of the charge-storage mechanism including the assessment of the electronic properties and quantum capacitance of the MXene heterostructure (MnCo2O4/Ti3C2Tx).71 It also provided the details on the interactions between orbitals, the bonding procedure, and the charge transfer capabilities of each electrode material. DFT simulations revealed higher electronic states around the Fermi level in the MnCo2O4/Ti3C2Tx hybrid structure and improved quantum capacitance, supporting the superior energy storage capability. The MnCo2O4/Ti3C2Tx hybrid shows a remarkable specific capacitance of 860.22 F g−1 at a current density of 2 A g−1.
5. Conclusions and outlook
MXenes are effective choices to overcome the limitations of currently available energy storage technologies, which not only offer great possibilities for supercapacitors and batteries with enhanced storage capabilities, improved cyclability, and fast charging–discharging, but also advance the continuously expanding materials field. Forming heterostructures of MXenes and oxides and using them as single electrodes is perhaps the most feasible way to improve the energy storage capacity of storage devices. The high conductivity of MXenes helps in retaining the redox capacity of oxides at higher rates and improves the cycling efficiency of oxides. Moreover, several studies have reported a suppressed dissolution of oxides in combination with MXenes.116 Therefore, MXene/oxide heterostructures are certainly effective choices and deserve sincere attention. Below, we highlight some opportunities that can be taken into consideration for future studies.
• The electrochemistry of MXenes is still in its infancy, which needs to be explored in different electrolytes such as organic, ionic liquid, WIS and mixed-ion electrolytes.
• It is important to establish the key electrochemical properties such as electron transfer, conductivity, ionic and charge transport, and capacitance, and different effective ways to exploit the electrochemistry of MXenes with a particular emphasis on mechanistic aspects.
• Combining MXenes and oxides under the optimized conditions is important for improved performance. When compared to single oxides, the electrical conductivity of a binary oxide is twice as high.102,103 For this reason, preparing hybrid electrodes of MXenes and binary TMOs could be an effective approach.
• One of the major challenges is the identification of the most suitable combinations of MXenes and TMOs for different energy storage devices. Herein, the role of computational prediction and theoretical investigations in pre-selecting the best possible materials is important. These studies are also important to reveal the dynamics of ions confined between the formed heterostructures.148
• 3D MXene/TMO heterostructures could be important for energy storage devices. Such heterostructures of various compositions and terminal groups may improve the ion diffusion and reactive sites.123
• The scalable production of MXenes and their heterostructures is another challenge and should be taken as an opportunity.
• The moderate energy density of supercapacitors has been a persistent challenge. While MXenes offer high capacitance, their energy density is limited by the electrochemically stable voltage window due to irreversible anodic oxidation. To address this issue, both structural and electrochemical modifications could be helpful in increasing the energy density. Partial oxidation of MXenes to form oxide nanostructures could be a possible strategy to increase the overall capacity of MXene electrodes. Similarly, asymmetric supercapacitors can be designed where MXenes can be employed as negative electrode materials and oxides as positive electrode materials. In this direction, suitable anodes could be selected based on the behaviour of the materials in the chosen electrolytes to pair up with MXene cathodes. Electrolytes play an important role in governing the energy density. Redesigned electrolytes such as WiS and ionic electrolytes usually show a high electrochemically stable voltage window, leading to a high energy density. Hence, there are numerous possibilities to increase the energy density of MXene and oxide based supercapacitors.
There is no denying to the fact that if sincere efforts are made to address the existing issues in the field of 2D materials for energy storage applications, a new era of tuneable and flexible electrochemical devices based on MXene-based technologies is not far away with a better and brighter future. The application of MXenes and their endless heterostructures in flexible and wearable supercapacitors is among hot research areas. It should be noted that the size, composition, shape, texture, thickness and electrode design and architectures play a vital role in energy storage. Therefore, rational design of MXene/oxide heterostructures with improved chemical and electrochemical stability in different electrolyte systems is crucial to obtain a wider electrochemically stable operational working window and enhanced supercapacitor performance.
Conflicts of interest
The authors declare no conflicts of interest to declare.
Acknowledgements
SMM thanks SERB-DST, New Delhi, India (Project CRG/2020/001769), BRNS, Mumbai, India (Project 58/14/17/2020-BRNS/37215), and IIT Indore for the financial support.
References
- Y. Feng, L. Tao, Z. Zheng, H. Huang and F. Lin, Energy Storage Mater., 2020, 31, 274–309 CrossRef.
- J. Liu, H. Mooney, V. Hull, S. J. Davis, J. Gaskell, T. Hertel, J. Lubchenco, K. C. Seto, P. Gleick, C. Kremen and S. Li, Science, 2015, 347, 1258832 CrossRef PubMed.
- M. Elimelech and W. A. Phillip, Science, 2011, 333, 712–717 CrossRef CAS PubMed.
- X. Yuan, F. Ma, L. Zuo, J. Wang, N. Yu, Y. Chen, Y. Zhu, Q. Huang, R. Holze, Y. Wu and T. Van Ree, Electrochem. Energy Rev., 2021, 4, 1–34 CrossRef CAS.
- Y. Zhai, Y. Dou, D. Zhao, P. F. Fulvio, R. T. Mayes and S. Dai, Adv. Mater., 2011, 23, 4828–4850 CrossRef CAS PubMed.
- Z. Zhang, X. Huang, H. Li, Y. Zhao and T. Ma, Appl. Surf. Sci., 2017, 400, 238–244 CrossRef CAS.
- H. Wang, C. Zhu, D. Chao, Q. Yan and H. J. Fan, Adv. Mater., 2017, 29, 1702093 CrossRef PubMed.
- Z. Mao, R. Wang, B. He, J. Jin, Y. Gong and H. Wang, Small, 2023, 19, 2207224 CrossRef CAS PubMed.
- F. Zhang, X. Guo, P. Xiong, J. Zhang, J. Song, K. Yan, X. Gao, H. Liu and G. Wang, Adv. Energy Mater., 2020, 10, 2000446 CrossRef CAS.
- S. Wang, S. Zhao, X. Guo and G. Wang, Adv. Energy Mater., 2022, 12, 2100864 CrossRef CAS.
- S. Niu, Z. Wang, M. Yu, M. Yu, L. Xiu, S. Wang, X. Wu and J. Qiu, ACS Nano, 2018, 12, 3928–3937 CrossRef CAS PubMed.
- N. Sun, Z. Guan, Y. Liu, Y. Cao, Q. Zhu, H. Liu, Z. Wang, P. Zhang and B. Xu, Adv. Energy Mater., 2019, 9, 1901351 CrossRef.
- Y. Zhong, B. Li, S. Li, S. Xu, Z. Pan, Q. Huang, L. Xing, C. Wang and W. Li, Nano-Micro Lett., 2018, 10, 56 CrossRef PubMed.
- N. Nitta, F. Wu, J. T. Lee and G. Yushin, Mater. Today, 2015, 18, 252–264 CrossRef CAS.
- A. E. Baumann, X. Han, M. M. Butala and V. S. Thoi, J. Am. Chem. Soc., 2019, 141, 17891–17899 CrossRef CAS PubMed.
- J. Duan, X. Tang, H. Dai, Y. Yang, W. Wu, X. Wei and Y. Huang, Electrochem. Energy Rev., 2020, 3, 1–42 CrossRef CAS.
- F. Wu, J. Maier and Y. Yu, Chem. Soc. Rev., 2020, 49, 1569–1614 RSC.
- X. Guo, H. Gao, S. Wang, G. Yang, X. Zhang, J. Zhang, H. Liu and G. Wang, Nano Lett., 2022, 22, 1225–1232 CrossRef CAS PubMed.
- X. Zhu, K. Wang, Y. Xu, G. Zhang, S. Li, C. Li, X. Zhang, X. Sun, X. Ge and Y. Ma, Energy Storage Mater., 2021, 36, 291–308 CrossRef.
- A. S. Aricò, P. Bruce, B. Scrosati, J.-M. Tarascon and W. Van Schalkwijk, Nat. Mater., 2005, 4, 366–377 CrossRef PubMed.
- S. N. Ansari, M. Saraf, A. K. Gupta and S. M. Mobin, Chem. – Asian J., 2019, 14, 3566–3571 CrossRef CAS PubMed.
- M. Saraf, R. Rajak and S. M. Mobin, J. Mater. Chem. A, 2016, 4, 16432–16445 RSC.
- M. Saraf, K. Natarajan and S. M. Mobin, ACS Sustainable Chem. Eng., 2018, 6, 10489–10504 CrossRef CAS.
- K. Natarajan, A. K. Gupta, S. N. Ansari, M. Saraf and S. M. Mobin, ACS Appl. Mater. Interfaces, 2019, 11, 13295–13303 CrossRef CAS PubMed.
- A. Halder, M. Ghosh, A. Khayum M, S. Bera, M. Addicoat, H. S. Sasmal, S. Karak, S. Kurungot and R. Banerjee, J. Am. Chem. Soc., 2018, 140, 10941–10945 CrossRef CAS PubMed.
- M. R. Lukatskaya, S. Kota, Z. Lin, M.-Q. Zhao, N. Shpigel, M. D. Levi, J. Halim, P.-L. Taberna, M. W. Barsoum, P. Simon and Y. Gogotsi, Nat. Energy, 2017, 2, 17105 CrossRef CAS.
- Y. Zhou, K. Maleski, B. Anasori, J. O. Thostenson, Y. Pang, Y. Feng, K. Zeng, C. B. Parker, S. Zauscher, Y. Gogotsi, J. T. Glass and C. Cao, ACS Nano, 2020, 14, 3576–3586 CrossRef CAS PubMed.
- M. D. Stoller, S. Park, Y. Zhu, J. An and R. S. Ruoff, Nano Lett., 2008, 8, 3498–3502 CrossRef CAS PubMed.
- P. He, H. Yu, D. Li and H. Zhou, J. Mater. Chem., 2012, 22, 3680 RSC.
- K. Kubota, N. Yabuuchi, H. Yoshida, M. Dahbi and S. Komaba, MRS Bull., 2014, 39, 416–422 CrossRef CAS.
- D. Kim, S.-H. Kang, M. Slater, S. Rood, J. T. Vaughey, N. Karan, M. Balasubramanian and C. S. Johnson, Adv. Energy Mater., 2011, 1, 333–336 CrossRef CAS.
- M. Chhowalla, H. S. Shin, G. Eda, L.-J. Li, K. P. Loh and H. Zhang, Nat. Chem., 2013, 5, 263–275 CrossRef PubMed.
- M. Pumera, Z. Sofer and A. Ambrosi, J. Mater. Chem. A, 2014, 2, 8981–8987 RSC.
- M. Naguib, V. N. Mochalin, M. W. Barsoum and Y. Gogotsi, Adv. Mater., 2014, 26, 992–1005 CrossRef CAS PubMed.
- Y.-T. Liu, P. Zhang, N. Sun, B. Anasori, Q.-Z. Zhu, H. Liu, Y. Gogotsi and B. Xu, Adv. Mater., 2018, 30, 1707334 CrossRef PubMed.
- M. R. Lukatskaya, O. Mashtalir, C. E. Ren, Y. Dall'Agnese, P. Rozier, P. L. Taberna, M. Naguib, P. Simon, M. W. Barsoum and Y. Gogotsi, Science, 2013, 341, 1502–1505 CrossRef CAS PubMed.
- Y. Xia, T. S. Mathis, M.-Q. Zhao, B. Anasori, A. Dang, Z. Zhou, H. Cho, Y. Gogotsi and S. Yang, Nature, 2018, 557, 409–412 CrossRef CAS PubMed.
- M.-Q. Zhao, C. E. Ren, Z. Ling, M. R. Lukatskaya, C. Zhang, K. L. Van Aken, M. W. Barsoum and Y. Gogotsi, Adv. Mater., 2015, 27, 339–345 CrossRef CAS PubMed.
- O. Mashtalir, M. R. Lukatskaya, M.-Q. Zhao, M. W. Barsoum and Y. Gogotsi, Adv. Mater., 2015, 27, 3501–3506 CrossRef CAS PubMed.
- M. Naguib, J. Halim, J. Lu, K. M. Cook, L. Hultman, Y. Gogotsi and M. W. Barsoum, J. Am. Chem. Soc., 2013, 135, 15966–15969 CrossRef CAS PubMed.
- X. Liang, Y. Rangom, C. Y. Kwok, Q. Pang and L. F. Nazar, Adv. Mater., 2017, 29, 1603040 CrossRef PubMed.
- X. Liang, A. Garsuch and L. F. Nazar, Angew. Chem., Int. Ed., 2015, 54, 3907–3911 CrossRef CAS PubMed.
- D. Er, J. Li, M. Naguib, Y. Gogotsi and V. B. Shenoy, ACS Appl. Mater. Interfaces, 2014, 6, 11173–11179 CrossRef CAS PubMed.
- M. Zhao, X. Xie, C. E. Ren, T. Makaryan, B. Anasori, G. Wang and Y. Gogotsi, Adv. Mater., 2017, 29, 1702410 CrossRef PubMed.
- M. Naguib, M. Kurtoglu, V. Presser, J. Lu, J. Niu, M. Heon, L. Hultman, Y. Gogotsi and M. W. Barsoum, Adv. Mater., 2011, 23, 4248–4253 CrossRef CAS PubMed.
- M. Saraf, T. Zhang, T. Averianov, C. E. Shuck, R. W. Lord, E. Pomerantseva and Y. Gogotsi, Small Methods, 2023, 2201551 CrossRef PubMed.
- X. Li, Z. Huang, C. E. Shuck, G. Liang, Y. Gogotsi and C. Zhi, Nat. Rev. Chem., 2022, 6, 389–404 CrossRef PubMed.
- M. Zhang, D. Jiang, F. Jin, Y. Sun, J. Wang, M. Jiang, J. Cao, B. Zhang and J. Liu, J. Colloid Interface Sci., 2023, 636, 204–215 CrossRef CAS PubMed.
- R. A. Chavan, D. M. Ulisso, A. S. Rasal, J. Y. Chang and A. V. Ghule, Mater. Adv., 2023, 4, 2659–2666 RSC.
- S. De, S. Acharya, C. K. Maity and G. C. Nayak, ACS Appl. Energy Mater., 2023, 6, 969–980 CrossRef CAS.
- R. A. Chavan, G. P. Kamble, S. B. Dhavale, A. S. Rasal, S. S. Kolekar, J.-Y. Chang and A. V. Ghule, Energy Fuels, 2023, 37, 4658–4670 CrossRef CAS.
- Y. Zhong, X. Xia, F. Shi, J. Zhan, J. Tu and H. J. Fan, Adv. Sci., 2016, 3, 1500286 CrossRef PubMed.
- B. Anasori, M. R. Lukatskaya and Y. Gogotsi, Nat. Rev. Mater., 2017, 2, 16098 CrossRef CAS.
- B. Mendoza-Sánchez and Y. Gogotsi, Adv. Mater., 2016, 28, 6104–6135 CrossRef PubMed.
- X. Xie, Z. Ao, D. Su, J. Zhang and G. Wang, Adv. Funct. Mater., 2015, 25, 1393–1403 CrossRef CAS.
- E. G. Da Silveira Firmiano, A. C. Rabelo, C. J. Dalmaschio, A. N. Pinheiro, E. C. Pereira, W. H. Schreiner and E. R. Leite, Adv. Energy Mater., 2014, 4, 1301380 CrossRef.
- C. Zhao, X. Wang, J. Kong, J. M. Ang, P. S. Lee, Z. Liu and X. Lu, ACS Appl. Mater. Interfaces, 2016, 8, 2372–2379 CrossRef CAS PubMed.
- Y. Wang, X. Wang, X. Li, Y. Bai, H. Xiao, Y. Liu, R. Liu and G. Yuan, Adv. Funct. Mater., 2019, 29, 1900326 CrossRef.
- C. Yu, Y. Gong, R. Chen, M. Zhang, J. Zhou, J. An, F. Lv, S. Guo and G. Sun, Small, 2018, 14, 1801203 CrossRef PubMed.
- S. Abdolhosseinzadeh, J. Heier and C. (John) Zhang, ChemElectroChem, 2021, 8, 1911–1917 CrossRef CAS.
- X. Chen, S. Wang, J. Shi, X. Du, Q. Cheng, R. Xue, Q. Wang, M. Wang, L. Ruan and W. Zeng, Adv. Mater. Interfaces, 2019, 6, 1901160 CrossRef CAS.
- W. Meng, J. Zhou, G. Wang, J. Qin, L. Yang, H. Huang, Y. Zhao and H. He, J. Energy Storage, 2022, 56, 106105 CrossRef.
- S. Fang, D. Bresser and S. Passerini, Adv. Energy Mater., 2020, 10, 1902485 CrossRef CAS.
- S. Yuan, X. Duan, J. Liu, Y. Ye, F. Lv, T. Liu, Q. Wang and X. Zhang, Energy Storage Mater., 2021, 42, 317–369 CrossRef.
- W.-B. Yu, Z.-Y. Hu, J. Jin, M. Yi, M. Yan, Y. Li, H.-E. Wang, H.-X. Gao, L.-Q. Mai, T. Hasan, B.-X. Xu, D.-L. Peng, G. Van Tendeloo and B.-L. Su, Natl. Sci. Rev., 2020, 7, 1046–1058 CrossRef PubMed.
- P. Zhang, N. Sun, R. A. Soomro, S. Yue, Q. Zhu and B. Xu, ACS Appl. Energy Mater., 2021, 4, 11844–11853 CrossRef CAS.
- R. Li, X. Ma, J. Li, J. Cao, H. Gao, T. Li, X. Zhang, L. Wang, Q. Zhang, G. Wang, C. Hou, Y. Li, T. Palacios, Y. Lin, H. Wang and X. Ling, Nat. Commun., 2021, 12, 1587 CrossRef CAS PubMed.
- X. Liu, F. Xu, Z. Li, Z. Liu, W. Yang, Y. Zhang, H. Fan and H. Y. Yang, Coord. Chem. Rev., 2022, 464, 214544 CrossRef CAS.
- S. Cao, B. Shen, T. Tong, J. Fu and J. Yu, Adv. Funct. Mater., 2018, 28, 1800136 CrossRef.
- E. Xu, Y. Zhang, H. Wang, Z. Zhu, J. Quan, Y. Chang, P. Li, D. Yu and Y. Jiang, Chem. Eng. J., 2020, 385, 123839 CrossRef CAS.
- M. Pathak, P. Mane, B. Chakraborty and C. S. Rout, J. Energy Storage, 2023, 66, 107475 CrossRef.
- H.-Y. Wang, X.-B. Sun, Y. Xin, S.-H. Yang, P.-F. Hu and G.-S. Wang, J. Mater. Sci. Technol., 2023, 134, 132–141 CrossRef.
- C. Yang, Y. Liu, X. Sun, Y. Zhang, L. Hou, Q. Zhang and C. Yuan, Electrochim. Acta, 2018, 271, 165–172 CrossRef CAS.
- M. Naguib, O. Mashtalir, M. R. Lukatskaya, B. Dyatkin, C. Zhang, V. Presser, Y. Gogotsi and M. W. Barsoum, Chem. Commun., 2014, 50, 7420–7423 RSC.
- N. M. Caffrey, Nanoscale, 2018, 10, 13520–13530 RSC.
- H.-W. Wang, M. Naguib, K. Page, D. J. Wesolowski and Y. Gogotsi, Chem. Mater., 2016, 28, 349–359 CrossRef CAS.
- L. H. Karlsson, J. Birch, J. Halim, M. W. Barsoum and P. O. Å. Persson, Nano Lett., 2015, 15, 4955–4960 CrossRef CAS PubMed.
- F. Liu, J. Zhou, S. Wang, B. Wang, C. Shen, L. Wang, Q. Hu, Q. Huang and A. Zhou, J. Electrochem. Soc., 2017, 164, A709–A713 CrossRef CAS.
- B. B. Sahoo, V. S. Pandey, A. S. Dogonchi, P. K. Mohapatra, D. N. Thatoi, N. Nayak and M. K. Nayak, J. Energy Storage, 2023, 65, 107335 CrossRef.
- R. S. Karmur, D. Gogoi, A. Biswas, C. Prathibha, M. R. Das and N. N. Ghosh, Appl. Surf. Sci., 2023, 623, 157042 CrossRef CAS.
- W. Chen, Y. Peng, Z. Qiu, X. Zhang and H. Xu, J. Alloys Compd., 2022, 901, 163614 CrossRef CAS.
- N. Li, Y. Jiang, C. Zhou, Y. Xiao, B. Meng, Z. Wang, D. Huang, C. Xing and Z. Peng, ACS Appl. Mater. Interfaces, 2019, 11, 38116–38125 CrossRef CAS PubMed.
- M. S. Javed, A. Mateen, I. Hussain, A. Ahmad, M. Mubashir, S. Khan, M. A. Assiri, S. M. Eldin, S. S. A. Shah and W. Han, Energy Storage Mater., 2022, 53, 827–872 CrossRef.
- W. Xi, J. Jin, Y. Zhang, R. Wang, Y. Gong, B. He and H. Wang, Nanoscale, 2022, 14, 11923–11944 RSC.
- X. Hui, X. Ge, R. Zhao, Z. Li and L. Yin, Adv. Funct. Mater., 2020, 30, 2005190 CrossRef CAS.
- K. Nasrin, V. Sudharshan, K. Subramani and M. Sathish, Adv. Funct. Mater., 2022, 32, 2110267 CrossRef CAS.
- X. Dong, Y. Zhang, B. Ding, X. Hao, H. Dou and X. Zhang, J. Power Sources, 2018, 390, 208–214 CrossRef CAS.
- N. Mahmood, I. A. De Castro, K. Pramoda, K. Khoshmanesh, S. K. Bhargava and K. Kalantar-Zadeh, Energy Storage Mater., 2019, 16, 455–480 CrossRef.
- S. T. Mahmud, M. M. Hasan, S. Bain, S. T. Rahman, M. Rhaman, M. M. Hossain and M. Ordu, ACS Mater. Lett., 2022, 4, 1174–1206 CrossRef CAS.
- X. Li, Z. Lin, Y. Wei, W. Luo, J. Ding, T. Li and Y. Ma, J. Energy Storage, 2022, 55, 105668 CrossRef.
- W. Zheng, J. Halim, L. Yang, H. O. Badr, Z. Sun, P. O. Å. Persson, J. Rosen and M. W. Barsoum, Batteries Supercaps, 2022, 5(10), e202200151 CAS.
- H. Jiang, Z. Wang, Q. Yang, M. Hanif, Z. Wang, L. Dong and M. Dong, Electrochim. Acta, 2018, 290, 695–703 CrossRef CAS.
- Y. Wei, M. Zheng, W. Luo, B. Dai, J. Ren, M. Ma, T. Li and Y. Ma, J. Energy Storage, 2022, 45, 103715 CrossRef.
- R. B. Rakhi, B. Ahmed, D. Anjum and H. N. Alshareef, ACS Appl. Mater. Interfaces, 2016, 8, 18806–18814 CrossRef CAS PubMed.
- Z. Pan, C. Yang, Y. Li, X. Hu and X. Ji, Chem. Eng. J., 2022, 428, 131138 CrossRef CAS.
- A. K. Tomar, T. Kshetri, N. H. Kim and J. H. Lee, Energy Storage Mater., 2022, 50, 86–95 CrossRef.
- S. Feng, X. Wang, M. Wang, C. Bai, S. Cao and D. Kong, Nano Lett., 2021, 21, 7561–7568 CrossRef CAS PubMed.
- H. Mustafa, A. Rasheed, S. Ajmal, N. Sarwar, U. Falak, S. G. Lee and A. Sattar, Energy Fuels, 2023, 37, 2410–2419 CrossRef CAS.
- L. Zhang, Z. Wang, W. Chen, R. Yuan, K. Zhan, M. Zhu, J. Yang and B. Zhao, Nanoscale, 2021, 13, 15343–15351 RSC.
- I. Ashraf, S. Ahmad, D. Dastan, C. Wang, H. Garmestani and M. Iqbal, Electrochim. Acta, 2022, 429, 141036 CrossRef CAS.
- S. Liu, T. Zeng, Y. Zhang, Q. Wan and N. Yang, Small, 2022, 18, 2204829 CrossRef CAS PubMed.
- A. M. Patil, N. Kitiphatpiboon, X. An, X. Hao, S. Li, X. Hao, A. Abudula and G. Guan, ACS Appl. Mater. Interfaces, 2020, 12, 52749–52762 CrossRef CAS PubMed.
- J. Yu, Z. Cui, X. Li, D. Chen, J. Ji, Q. Zhang, J. Sui, L. Yu and L. Dong, J. Energy Storage, 2020, 27, 101165 CrossRef.
- J. Zhou, B. Liu, L. Zhang, Q. Li, C. Xu and H. Liu, J. Mater. Chem. A, 2022, 10, 24896–24904 RSC.
- P. Asen, A. Esfandiar and H. Mehdipour, Nanoscale, 2022, 14, 1347–1362 RSC.
- M. S. Javed, H. Lei, H. U. Shah, S. Asim, R. Raza and W. Mai, J. Mater. Chem. A, 2019, 7, 24543–24556 RSC.
- Y. Wang, J. Sun, X. Qian, Y. Zhang, L. Yu, R. Niu, H. Zhao and J. Zhu, J. Power Sources, 2019, 414, 540–546 CrossRef CAS.
- H. Li, X. Li, J. Liang and Y. Chen, Adv. Energy Mater., 2019, 9, 1970050 CrossRef.
- H. Huang, X. Wang, E. Tervoort, G. Zeng, T. Liu, X. Chen, A. Sologubenko and M. Niederberger, ACS Nano, 2018, 12, 2753–2763 CrossRef CAS PubMed.
- L. Qin, S. Zhu, C. Cheng, D. Wu, G. Wang, L. Hou and C. Yuan, Small, 2022, 18, 2107987 CrossRef CAS PubMed.
- L. Qin, Y. Liu, S. Zhu, D. Wu, G. Wang, J. Zhang, Y. Wang, L. Hou and C. Yuan, J. Mater. Chem. A, 2021, 9, 20405–20416 RSC.
- Q. Zhang, J. Rong, D. Ma and B. Wei, Energy Environ. Sci., 2011, 4, 2152 RSC.
- M. Xia, J. Nie, Z. Zhang, X. Lu and Z. L. Wang, Nano Energy, 2018, 47, 43–50 CrossRef CAS.
- L. Xiao, Z. Hu, Y. He, L. Jiao, L. Lv, Q. Yin, Q. Wei, Z. Li and Y. Yang, ACS Appl. Energy Mater., 2023, 6, 68–78 CrossRef CAS.
- Z. Wang, Z. Xu, H. Huang, X. Chu, Y. Xie, D. Xiong, C. Yan, H. Zhao, H. Zhang and W. Yang, ACS Nano, 2020, 14, 4916–4924 CrossRef CAS PubMed.
- M. Saraf, C. E. Shuck, N. Norouzi, K. Matthews, A. Inman, T. Zhang, E. Pomerantseva and Y. Gogotsi, Energy Environ. Mater., 2023, e12516 CrossRef.
- W. Zheng, J. Halim, Z. Sun, J. Rosen and M. W. Barsoum, Energy Storage Mater., 2021, 38, 438–446 CrossRef.
- G. Valurouthu, R. Panigrahi, M. Saraf, C. E. Shuck, B. S. Mallik, N. Kurra and Y. Gogotsi, Batteries Supercaps, 2023, 6, e202300009 CrossRef CAS.
- J. Li, X. Wang, W. Sun, K. Maleski, C. E. Shuck, K. Li, P. Urbankowski, K. Hantanasirisakul, X. Wang, P. Kent, H. Wang and Y. Gogotsi, ChemElectroChem, 2021, 8, 151–156 CrossRef CAS.
- Y. Luo, Y. Tang, X. Bin, C. Xia and W. Que, Small, 2022, 18, 2204917 CrossRef CAS PubMed.
- T.-Z. Shi, Y.-L. Feng, T. Peng and B.-G. Yuan, Electrochim. Acta, 2021, 381, 138245 CrossRef CAS.
- W. Luo, Y. Sun, Z. Lin, X. Li, Y. Han, J. Ding, T. Li, C. Hou and Y. Ma, J. Energy Storage, 2023, 62, 106807 CrossRef.
- Y. Ma, H. Sheng, W. Dou, Q. Su, J. Zhou, E. Xie and W. Lan, ACS Appl. Mater. Interfaces, 2020, 12, 41410–41418 CrossRef CAS PubMed.
- X. Zhang, B. Shao, A. Guo, Z. Gao, Y. Qin, C. Zhang, F. Cui and X. Yang, J. Alloys Compd., 2021, 862, 158546 CrossRef CAS.
- Y. Wang, X. Wang, X. Li, R. Liu, Y. Bai, H. Xiao, Y. Liu and G. Yuan, Nano-Micro Lett., 2020, 12, 115 CrossRef CAS PubMed.
- P. Zhang, Y. Sui, W. Ma, N. Duan, Q. Liu, B. Zhang, H. Niu and C. Qin, Dalton Trans., 2023, 52, 710–720 RSC.
- X. Wu, B. Huang, Q. Wang and Y. Wang, Chem. Eng. J., 2020, 380, 122456 CrossRef CAS.
- W. Chen, Z. Geng, S. Zhu, Z. Qiu, X. Zhang and H. Xu, Sustainable Energy Fuels, 2022, 6, 4938–4947 RSC.
- J. Yu, M. Zeng, J. Zhou, H. Chen, G. Cong, H. Liu, M. Ji, C. Zhu and J. Xu, Chem. Eng. J., 2021, 426, 130765 CrossRef CAS.
- L. Zhang, K. Yu, Y. Li, Z. Wang, K. Zhan, J. Yang and B. Zhao, ACS Appl. Nano Mater., 2023, 6, 482–491 CrossRef CAS.
- W. Chen, C. Hao, Z. Qiu, X. Zhang, H. Xu, B. Yu and S. Chen, ACS Appl. Mater. Interfaces, 2022, 14, 19534–19546 CrossRef CAS PubMed.
- H. Zhu, Z. Liang, S. Xue, X. Ren, X. Liang, W. Xiong, L. Gao and A. Liu, Ceram. Int., 2022, 48, 27217–27239 CrossRef CAS.
- V. M. Hong Ng, H. Huang, K. Zhou, P. S. Lee, W. Que, J. Z. Xu and L. B. Kong, J. Mater. Chem. A, 2017, 5, 3039–3068 RSC.
- Y. Bai, K. Zhou, N. Srikanth, J. H. L. Pang, X. He and R. Wang, RSC Adv., 2016, 6, 35731–35739 RSC.
- C. Zhan, W. Sun, Y. Xie, D. Jiang and P. R. C. Kent, ACS Appl. Mater. Interfaces, 2019, 11, 24885–24905 CrossRef CAS PubMed.
- M. Ashton, N. Trometer, K. Mathew, J. Suntivich, C. Freysoldt, S. B. Sinnott and R. G. Hennig, J. Phys. Chem. C, 2019, 123, 3180–3187 CrossRef CAS.
- X. Sang, Y. Xie, M.-W. Lin, M. Alhabeb, K. L. Van Aken, Y. Gogotsi, P. R. C. Kent, K. Xiao and R. R. Unocic, ACS Nano, 2016, 10, 9193–9200 CrossRef CAS PubMed.
- J. L. Hart, K. Hantanasirisakul, A. C. Lang, B. Anasori, D. Pinto, Y. Pivak, J. T. Van Omme, S. J. May, Y. Gogotsi and M. L. Taheri, Nat. Commun., 2019, 10, 522 CrossRef CAS PubMed.
- T. Bashir, S. A. Ismail, J. Wang, W. Zhu, J. Zhao and L. Gao, J. Energy Chem., 2023, 76, 90–104 CrossRef CAS.
- X. Sang, Y. Xie, D. E. Yilmaz, R. Lotfi, M. Alhabeb, A. Ostadhossein, B. Anasori, W. Sun, X. Li, K. Xiao, P. R. C. Kent, A. C. T. Van Duin, Y. Gogotsi and R. R. Unocic, Nat. Commun., 2018, 9, 2266 CrossRef PubMed.
- Z. Liu, L. Zheng, L. Sun, Y. Qian, J. Wang and M. Li, J. Am. Ceram. Soc., 2014, 97, 67–69 CrossRef CAS.
- Y. Gogotsi and B. Anasori, ACS Nano, 2019, 13, 8491–8494 CrossRef CAS PubMed.
- J. Wang, W. Liu, G. Luo, Z. Li, C. Zhao, H. Zhang, M. Zhu, Q. Xu, X. Wang, C. Zhao, Y. Qu, Z. Yang, T. Yao, Y. Li, Y. Lin, Y. Wu and Y. Li, Energy Environ. Sci., 2018, 11, 3375–3379 RSC.
- S. J. Kim, H.-J. Koh, C. E. Ren, O. Kwon, K. Maleski, S.-Y. Cho, B. Anasori, C.-K. Kim, Y.-K. Choi, J. Kim, Y. Gogotsi and H.-T. Jung, ACS Nano, 2018, 12, 986–993 CrossRef CAS PubMed.
- S. Stankovich, D. A. Dikin, R. D. Piner, K. A. Kohlhaas, A. Kleinhammes, Y. Jia, Y. Wu, S. T. Nguyen and R. S. Ruoff, Carbon, 2007, 45, 1558–1565 CrossRef CAS.
- M. Khazaei, M. Arai, T. Sasaki, C.-Y. Chung, N. S. Venkataramanan, M. Estili, Y. Sakka and Y. Kawazoe, Adv. Funct. Mater., 2013, 23, 2185–2192 CrossRef CAS.
- M. Khazaei, A. Ranjbar, M. Arai, T. Sasaki and S. Yunoki, J. Mater. Chem. C, 2017, 5, 2488–2503 RSC.
- E. Pomerantseva and Y. Gogotsi, Nat. Energy, 2017, 2, 17089 CrossRef CAS.
|
This journal is © The Royal Society of Chemistry 2023 |
Click here to see how this site uses Cookies. View our privacy policy here.