Polyethylene microplastics affect behavioural, oxidative stress, and molecular responses in the Drosophila model
Received
7th September 2024
, Accepted 24th October 2024
First published on 24th October 2024
Abstract
The escalating presence of microplastic pollution poses a significant environmental threat, with far-reaching implications for both ecosystems and human health. This study investigated the toxicological impact of polyethylene microplastics (PE MPs) using Drosophila melanogaster, fruit flies, as a model organism. Drosophila were exposed to PE MPs orally at concentrations of 1 mg ml−1 and 10 mg ml−1 agar food. The study assessed behavioural parameters and biochemical markers including reactive oxygen species (ROS), superoxide dismutase (SOD), and glutathione-S-transferase (GST) activity. The expression levels of key genes (Hsp70Bc, rpr, and p53) were also analysed using the RT-qPCR technique. Results indicated a significant decline in climbing activity among adult flies and crawling behaviour in larvae, indicating potential disruption of motor function. Biochemical analysis revealed elevated ROS levels, indicative of oxidative stress, in both larval and fly stages. Moreover, the antioxidant defence system exhibited decreased SOD activity and a concentration-dependent increase in GST activity indicating the functioning of a quick xenobiotic clearance mechanism. Gene expression analysis demonstrated upregulation of rpr, p53, and Hsp70Bc genes, suggesting activation of cell death pathways and stress response mechanisms. Overall, these findings underline the adverse effects of PE MPs on Drosophila, including behavioural impairment, oxidative stress, and activation of stress response pathways.
Environmental significance
Despite recycling efforts, 80% of plastic waste remains untreated, polluting land, water, and air, and breaking down into harmful microplastics permeating all ecosystems, from oceans to soil and air. Their presence has profound environmental implications. In aquatic environments, fish, molluscs, and plankton ingest microplastics, leading to inflammation, organ damage, and altered behaviours. These particles also accumulate in terrestrial ecosystems, affecting organisms and entering the human food chain through contaminated water, food, and air. This research emphasises the need for further investigation, particularly using Drosophila melanogaster, to understand the impact of polyethylene microplastics on terrestrial organisms and human health.
|
1 Introduction
Plastic, an incredibly versatile material, has become ubiquitous in modern society due to its durability, low cost, and ease of manufacturing, resulting in today's production of more than 400 million tonnes of plastic every year and a forecasted global production of 1.1 billion tonnes by 2050.1 Despite efforts toward recycling, nearly 80% of plastic waste remains unprocessed, infiltrating landfills, water bodies, and natural habitats.2 Once subjected to environmental forces such as sunlight, wind, and water, plastic undergoes fragmentation, ultimately giving rise to microplastics.3 These tiny particles, measuring less than 5 mm in size, pervade virtually all types of ecosystems, from oceans to freshwater bodies, soil, and even the air we breathe.4 Studies have shown that these minute plastic particles, despite their size, can have profound effects on diverse organisms.5
Microplastics have been found to accumulate in tissues, alter feeding behaviors, interfere with reproduction, and induce physiological stress in a range of species.6 In aquatic ecosystems, organisms such as fish, molluscs, crustaceans, and plankton are particularly susceptible to ingesting microplastics either directly or indirectly through the food chain.7 Research indicates that the ingestion of polystyrene (PS) microplastics leads to adverse effects across various organisms. For instance, in zebrafish, it triggers inflammation and lipid accumulation in the liver.8 Similarly, silver carp experience significant damage to their gills and intestines upon exposure to these microplastics.9 Whereas in Tigriopus japonicus (marine copepod), microplastics accumulated in the intestinal tract and induced reactive oxygen species (ROS) generation. Furthermore, there was an alteration in the expression of antioxidant-related genes and changes in antioxidant enzyme activity were observed.10
Ingestion of polyvinyl chloride (PVC) microplastic caused weight loss in Carassius auratus, (goldfish) and damage to organs and tissue of the gastrointestinal system.11 Polyethylene (PE) microplastics (MPs) cause developmental toxicity in Danio rerio (zebrafish) revealing adverse effects on hatching rate and post-hatching larval survival.12 Similarly, it affects Daphnia magna (water fleas) metabolism, showing significant changes in metabolites and a reduction in survival and heart rate.13 They also impact human cell lines, demonstrating cell-specific effects and increased production of nitric oxide and ROS.14 Research has found that the smaller the plastic particles, the more substantial the negative effects they cause, in terms of ultrastructural alterations, ROS generation, and antioxidant responses.15 Smaller microplastics are more likely to penetrate tissues and cells, potentially causing physical damage or interfering with cellular processes.16
While studies have extensively investigated the effects of microplastics on aquatic species, research on their impacts on terrestrial ecosystems is comparatively scarce. Moreover, existing studies have predominantly focused on certain types of microplastics, such as PS and polyethylene terephthalate (PET), with limited attention given to other polymers like PE. PE is primarily used in packaging, from bags to bottles, due to its durability and moisture resistance. Additionally, it serves in construction for pipes and insulation, while also appearing in consumer goods, medical devices, automotive components, and various industrial applications. PE MPs have been found in soil,17 water bodies and a range of personal care products18 and pose a threat to humans and wildlife.
Humans can be exposed to microplastics through various pathways. One common route is through the consumption of contaminated food and water.19 Seafood, such as fish and shellfish, can contain microplastics that have been ingested by marine organisms, thereby entering the human food chain.20 Additionally, microplastics have been found in drinking water, both bottled and tap, as well as in other beverages.21 Inhalation of microplastics is another potential route of exposure, as these particles have been detected in the air, particularly in indoor environments.22 Furthermore, microplastics can leach from consumer products, such as food packaging, utensils, and cosmetics, exposing individuals through direct contact.23 Microscopic plastic fragments have been observed in human excrement,24 and scientists have found minuscule plastic particles in the placentas of women.25 So, it is important to study the toxicity of microplastic in terrestrial organisms.
Drosophila melanogaster (fruit fly) presents a unique opportunity for studying the toxicity of polyethylene microplastics on a representative terrestrial organism. This model offers numerous advantages for experimentation, including a short life cycle, easy and low cost of maintenance, and ethical approval. Furthermore, Drosophila possesses functional homologs for over 75% of the genetic material responsible for human diseases.26 Past research has successfully utilized Drosophila to assess potential dangers associated with exposure to polyethylene terephthalate27 and polystyrene microplastics.28
In this study, we administered polyethylene microplastics (PE MPs) orally at concentrations of 1 mg ml−1 and 10 mg ml−1 to investigate their effects on various biochemical parameters, including ROS, superoxide dismutase (SOD), and glutathione S-transferase (GST) levels, as well as on the locomotor activity of both flies and larvae. Additionally, we examined the mRNA expression levels of key stress response genes, such as rpr, Hsp70Bc, and p53.
Leveraging the benefits of the Drosophila model, we conducted comprehensive research to investigate the impacts of polyethylene microplastics. Examining reactive oxygen species (ROS), superoxide dismutase (SOD), and glutathione S-transferase (GST) levels in Drosophila is critical for assessing the toxic nature of microplastics. ROS are markers of oxidative stress, indicating cellular damage, while SOD and GST are antioxidant enzymes that help mitigate this damage. Locomotor effects serve as behavioural indicators of neurotoxicity and overall health, providing insights into how microplastics impact physiological functions. Together, these measures form a comprehensive assessment of microplastic toxicity, aiding in understanding environmental and health risks.
2 Materials and methods
2.1 Chemicals
Propionic acid, copper sulphate, phenazonium methosulphate (PMS), sodium potassium tartrate, nicotinamide adenine dinucleotide (NADH), D-glucose, sodium hydroxide, yeast extract powder, sodium carbonate, chloroform, nitro blue tetrazolium (NBT), tetrasodium pyrophosphate (TSPP), orthophosphoric acid, glacial acetic acid, 4′,6-diamidino-2-phenylindole (DAPI) were acquired from Sisco Research Laboratories (SRL) Chemicals, Mumbai. Methyl para-hydroxy benzoate was obtained from Rankem, New Delhi. From HiMedia Mumbai (India), ethanol, sodium phosphate dibasic, agar–agar type I, and sodium phosphate monobasic were procured. 2′,7′-Dichlorofluorescein diacetate (DCHF-DA) and Polyethylene Ultra-high molecular weight, particle size 34–50 μm were obtained from Sigma Aldrich, India.
2.2 Transmission electron microscopy (TEM) imaging of PE MPs
The size and morphology of commercial PE MPs were examined using JEM-2100 Plus Hi-Resolution Transmission Electron Microscope (HRTEM), JEOL Japan.
2.3
Drosophila rearing
The study utilized the Oregon K wild-type strain, which was maintained under specific conditions: a temperature of 26 °C, 60–65% humidity, and a 12 hours light and dark cycle. The flies were raised on cornmeal-agar food comprising glucose, sucrose, agar–agar, yeast extract powder and corn flour. To inhibit fungal growth, antifungal agents including propionic acid, orthophosphoric acid, and Tego (methylparaben dissolved in ethanol) were added to the autoclaved cornmeal agar at 58–60 °C.
2.4 Experimental setup
The experimental group consisted of control, 1 mg ml−1, and 10 mg ml−1 of PE MPs. A stock solution of PE MP at a concentration of 100 mg ml−1 was prepared, from which two treatment groups were established: one at 1 mg ml−1 and the other at 10 mg ml−1. The PE MPs were administered orally to the flies by mixing them with food and they were exposed to the treatments for 14 days under controlled laboratory conditions. All assays were performed in triplicates to attain statistical significance.
2.5 Crawling activity
The experiment with third-instar larvae was conducted using a previously established methodology.29 The larvae were acquired by placing flies in a 2
:
1 ratio of females to males in both control and PE MP-containing food vials. After 3 days, adult flies were removed, and the larvae were allowed to mature until the 3rd instar stage. Subsequently, these larvae were utilized for conducting the crawling assay. Larvae were collected from the food vial and rinsed with distilled water to remove excess media from the body. Using a brush, the larvae were transferred onto an agar Petri dish and allowed to crawl over the agar plate on a graph sheet. The entire procedure was recorded on camera. The number of grid lines crossed by the larvae in one minute was counted, and the mean distance travelled by the larvae from each group was used to calculate the distance traversed by each treatment group in one minute.
2.6 Climbing activity
The climbing experiment was carried out following previously documented research.30 Twenty male flies were added to media vials with two concentrations of PE MPs (1 and 10 mg ml−1). The flies were kept in these treatment vials for 14 days, and the food was replaced every three days. After 14 days of exposure, the flies were transferred to 10 cm vials with 3 cm markings. The vial aperture was closed with a plug, which was tapped 3–4 times to confirm that all flies were at the bottom. Following that, the flies were permitted to ascend for 10 seconds, and the whole procedure was videotaped. The video recording was used to count the number of flies that successfully ascended beyond 3 cm within 10 seconds. This assay was performed in triplicate for each control and treatment group, and the percentage of flies that crossed the marked distance was calculated based on the average number of flies that crossed the labelled distance.
2.7 Homogenate preparation
One millilitre of 0.1 M sodium phosphate buffer was used to homogenize twenty newborn male flies31 third instar larvae,32 from each treatment group. The homogenate was centrifuged at 6000 rpm for 10 minutes in a cooling centrifuge at 4 °C. Following centrifugation, the supernatant was carefully collected without disrupting the pellet and used for the estimation of proteins, and superoxide dismutase activity and to determine the levels of glutathione S-transferase. Similarly, tris buffer was used to prepare fly/larvae homogenate for the estimation of the total reactive oxygen species. The homogenate was centrifuged at 5000 rpm for 10 minutes at 4 °C to obtain the supernatant which was used for the assay.
2.8 Protein estimation
Protein content in larvae and the fly homogenate was determined by the Lowry method,33 using BSA as standard. 0.1 ml of tissue homogenate was used and following incubations with Lowry reagents, produced a blue colour solution. The intensity of this colour, measured by absorbance at 660 nm, correlates directly with the protein concentration.
2.9 Estimation of reactive oxygen species
2′,7′-Dichlorodihydrofluorescein diacetate (DCFDA) was used to measure the intracellular ROS levels following a previously established method.34 Briefly, 0.1 ml of homogenate was mixed with 10 μM DCFDA solution in a 96-well microplate. The plate was then kept in the dark at room temperature for 60 minutes. A fluorescence microplate reader was used to measure the fluorescence intensity; the excitation and emission wavelengths were set at 488 nm and 525 nm, respectively.
2.10 Estimation of superoxide dismutase activity
The activity of superoxide dismutase (SOD) was determined at room temperature using the previously described method.35 The reaction mixture consisted of 0.1 ml of 186 μM phenazine methosulfonate, 0.2 ml of fly/larval homogenate, 0.2 ml of H2O, 1 ml of 0.052 M TSPP (pH 8), and 0.3 ml of NBT. 780 μM NADH was added to the reaction mixture to start the reaction. A spectrophotometer was used to measure the change in absorbance at 560 nm when the reaction was stopped with acetic acid after one minute. One unit of enzyme activity typically refers to the amount of enzyme that causes a 50% inhibition of the reduction of NBT under specified assay conditions.
2.11 Estimation of glutathione-S-transferase activity
Glutathione-S-transferase (GST) activity is determined using 1-chloro-2,4-dinitrobenzene (CNDB) and reduced glutathione (GSH) according to the method described by Umamaheswari et al.36 The protocol involves incubating the fly/larvae homogenate with a reaction mixture containing 10 mM GSH and 10 mM CNDB, followed by the measurement of absorbance at 340 nm to assess the formation of the GST-catalysed product.
2.12 Gene expression
The mRNA expression of the stress response gene – Hsp70Bc, genotoxic stress response gene – p53 and apoptosis marker in Drosophila melanogaster model – rpr (reaper) were analysed in male flies from PE MPs treatment and control group. Twenty flies were selected for RNA isolation using the TRIzol method, and the purity of RNA was assessed using NanoDrop. cDNA synthesis was carried out by adding the 1 μg RNA to a reaction mixture prepared according to the iScript cDNA Synthesis Kit protocol. The expression levels of Hsp70Bc, rpr, and p53 genes were measured by quantitative real-time polymerase chain reaction (qRT-PCR), which was carried out on a QuantStudio 5 Real-Time PCR system (Applied Biosystems) using a 20 μL reaction mixture made up of cDNA template, gene-specific primers (sequence provided in Table 1) and 2 × TB Green® Premix Ex Taq™ II (Takara). qRT-PCR was performed using the following cycling conditions: 30 seconds of initial denaturation at 95 °C, 35 cycles of denaturation at 95 °C for 5 seconds, and 35 cycles of annealing/extension at 60 °C for 35 seconds. Using rp49 as the housekeeping gene and the comparative Ct (threshold cycle) technique (2-ΔΔCt), relative gene expression levels were determined. All the samples were run in triplicates .
Table 1 Primers for qRT-PCR
Genes |
Forward primer |
Reverse primer |
Hsp70Bc
|
AAGAACCTCAAGGGTGAGCG |
CGAACAGAGATCCCTCGTCG |
rpr
|
TGCGCCAGTACACTTCATGT |
GCTTGCGATATTTGCCGGAC |
p53
|
TTGGATGTACTCGATTCCGCT |
TTAGACTTGAACTGAACGTCCAC |
rp49
|
GTTTCCGGCAAGGTATGTGC |
AAATACGAGCCGCACACTTG |
2.13 Identification of PE MPs in larvae gut
The accumulation of PE MPs in the gut of Drosophila larvae has been identified by staining them with Nile red, using a previously described method with slight modifications.27 Briefly, 0.5 ml of Nile red (in 50% acetone) was added to the PE MPs and stirred at room temperature for 8 hours in the dark. This mixture was then centrifuged at 12
000 rpm for 30 minutes, and after centrifugation, the supernatant was discarded, and the pellet was washed with 0.1 M phosphate-buffered saline (PBS) (in 50% ethanol) to remove excess stain. Subsequently, the pellet was dried and mixed with cornmeal agar, and the larvae were exposed to this food for 48 hours. The presence of microplastics was observed under a fluorescence microscope by examining the dissected larvae gut. Additionally, the larval gut was also stained with DAPI37 and DCFDA.38
2.14 Statistical analysis
The values in the graphs are reported as mean ± SEM. ANOVA followed by Dunnett's multiple comparison tests were used in the statistical analysis, which was conducted using GraphPad Prism 6.0, to compare the treatment groups to the controls. The significance level was set at p < 0.05.
3 Result and discussion
3.1 Transmission electron microscope imaging of PE MPs
Basic characterization of the commercial PE MPs through TEM imaging and selected area electron diffraction (SAED) patterns revealed amorphous agglomerated particles in the 35–50 μm size range, as shown in Fig. 1.
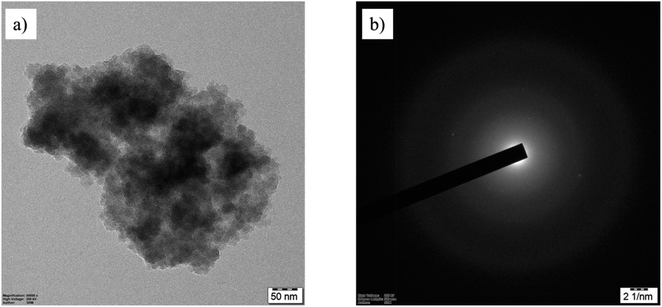 |
| Fig. 1 Characterization of PE MPs. (a) TEM image of PE MPs. (b) The diffused ring patterns observed in the SAED data confirm PE MPs as agglomerates. | |
3.2 Crawling assay
Drosophila larvae's locomotor function may be used to assess possible neuronal damage since their movement is dependent on body wall musculature contraction, which is controlled by motor neurons in the ventral ganglion's dorsal area.39 Additionally, the larval crawling assay serves as a tool to assess toxicity in Drosophila by examining its impact on larval crawling ability.
In this context, the distances covered by control larvae is 46 mm min−1, while distances travelled by larvae from 1 mg ml−1, and 10 mg ml−1 treatment group were recorded as 49 mm min−1, and 33.5 mm min−1, respectively (Fig. 2a). The decrease in crawling activity observed in Drosophila larvae from the 10 mg ml−1 treatment group compared to the control group could be due to attribution to various factors including stress induced by exposure to PE MP, nutritional deficiencies affecting energy levels, and the possibility of neurotoxic effects caused by MPs. This is similar to the results of Kauts et al. who saw a similar decrease in the crawling activity of larvae from 20 g l−1 and 40 g l−1 PET microplastic.27 Studies have also shown microplastic accumulation in larvae guts, potentially harming their digestive system and overall health.40,41 This can lead to reduced mobility and energy levels, hindering their crawling ability. Microplastic exposure during the larval stage might disrupt their development.
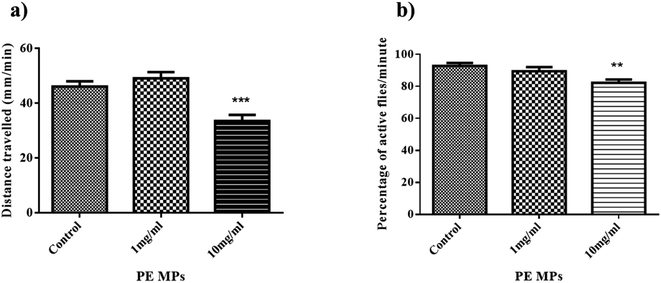 |
| Fig. 2 Effect of PE MPs on the behaviour of the third-instar larvae and adult flies. (a) The crawling activity of Drosophila larvae (b) The climbing activity of Drosophila flies. The data are presented as mean ± SEM, and they were analysed using ANOVA and Dunnett's multiple comparison test. Statistical significance was considered at p < 0.05. **indicates p < 0.01 ***indicates p < 0.001. | |
3.3 Climbing assay
Negative geotaxis in Drosophila refers to the instinctive behavior of these fruit flies to move against the force of gravity.42 This behavior is commonly used in climbing assays to assess locomotor abilities. After 14 days of exposure, the proportion of flies that could pass the 3 cm mark in 10 seconds in the control, 1 mg ml−1, and 10 mg ml−1 treatment groups was 92%, 89%, and 82%, respectively (Fig. 2b). The climbing ability of flies from the 10 mg ml−1 treatment group significantly decreased compared to that of the control group. A similar result was observed by Kauts et al., where flies from the 20 g l−1 and 40 g l−1 PET MPs treatment group have significantly decreased climbing activity.27 The observed decrease in climbing ability following exposure to 10 mg ml−1 of PE MPs suggests changes in metabolism as well as behavioural factors, signifying alterations in energy utilization and potentially disrupted physiological processes. Studies suggest microplastic accumulates in their bodies, potentially affecting their nervous system and muscles.43 This can lead to reduced mobility and strength, making climbing difficult.
3.4 Protein estimation
Protein content in Drosophila larvae and flies was measured using Lowrey's method. In Drosophila flies, the protein content in the control, 1 mg ml−1, and 10 mg ml−1 treatment groups was measured at 1.163 mg ml−1, 1.112 mg ml−1, and 1.120 mg ml−1 respectively (Fig. 3a). Although there was a slight decrease in protein content in the exposed groups compared to the control group, these changes were not statistically significant. Similarly, in Drosophila larvae, the protein content in the control, 1 mg ml−1, and 10 mg ml−1 groups was determined to be 1.198 mg ml−1, 1.22 mg ml−1, and 0.98 mg ml−1 respectively (Fig. 3a). Like in the flies, there was a nonsignificant decrease in protein content in the exposed groups compared to the control group. Protein concentration was used to estimate the enzyme activity in flies and larvae.
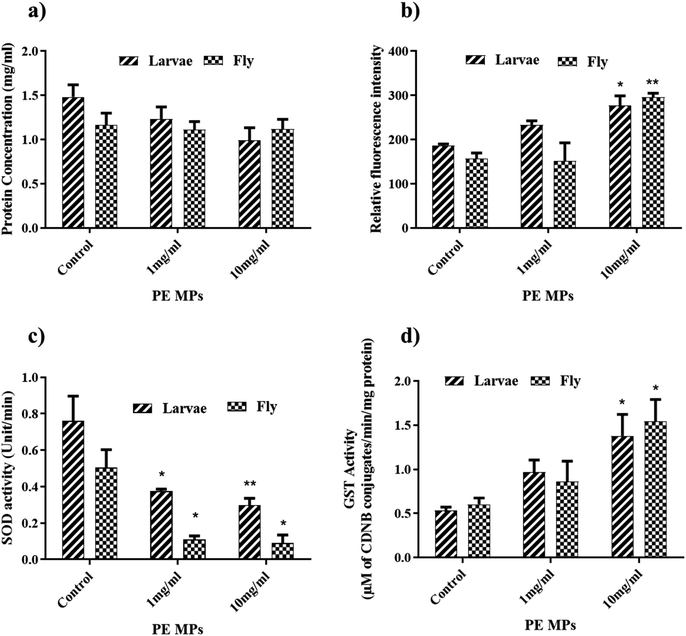 |
| Fig. 3 Biochemical parameters were analysed on adult male flies and third-instar larvae after exposure to PE MPs. (a) Estimation of protein levels. (b) Reactive oxygen species levels. (c) Superoxide dismutase activity. (d) Glutathione S-transferase activity. The data were presented as mean ± SEM and analysed using ANOVA and Dunnett's multiple comparison test. Statistical significance was considered at p < 0.05. * indicates p < 0.05 **indicates p < 0.01. | |
3.5 ROS estimation
Since high levels of reactive oxygen species (ROS) are a known indicator of toxicity, and past research links oxidative stress to behavioural changes, we investigated ROS levels in homogenates of Drosophila flies and larvae. In flies (Fig. 3b) a significant increase in ROS levels was observed only in the 10 mg ml−1 PE MP exposure group compared to the control and 1 mg ml−1 exposure groups. Larvae (Fig. 3b) exhibited a non-significant increase in ROS levels in the 1 mg ml−1 exposure group compared to the control, however, the 10 mg ml−1 exposure group showed a significant increase in ROS levels. The significant increase in ROS levels in both flies and larvae exposed to the 10 mg ml−1 treatment group suggests a dose-dependent effect of PE MPs on oxidative stress. This indicates that higher concentrations of PE MPs have a more pronounced impact on inducing oxidative stress in both life stages of Drosophila.
Mohamed Alaraby et al. also observed a similar result where at higher PET NPs concentrations (500 μg ml−1 food) intracellular ROS in Drosophila larvae has been detected.44 Evidence from various experimental models indicates that exposure to polyethylene (PE) microplastics significantly increases reactive oxygen species (ROS) levels, contributing to oxidative stress. For instance, studies have demonstrated that microplastics induce ROS production in Daphnia magna and rodent models, leading to reproductive and developmental toxicity. Specifically, exposure to PE microplastics has been shown to activate MAPK signalling pathways via oxidative stress, which correlates with increased ROS levels and subsequent cellular damage45.
3.6 SOD activity
SOD is an important antioxidant enzyme that defends cells against oxidative stress. Three groups were established for both flies and larvae: control, 1 mg ml−1, and 10 mg ml−1 microplastic exposure. SOD activity was measured in units per minute per milligram of protein (U per min per mg protein). In flies, SOD activity (Fig. 3c) showed a significant decrease with increasing microplastic concentration. Compared to the control group (0.203 U per min per mg protein), flies exposed to 1 mg ml−1 and 10 mg ml−1 microplastic exhibited reductions in SOD activity of 47% (0.108 U per min per mg protein) and 39% (0.123 U per min per mg protein), respectively.
Similar to flies, larvae displayed a concentration-dependent decline in SOD activity (Fig. 3c). The control group exhibited the highest activity (0.76 U per min per mg protein), while the 1 mg ml−1 and 10 mg ml−1 exposure groups showed reductions of 50% (0.375 U per min per mg protein) and 61% (0.299 U per min per mg protein), respectively. Superoxide dismutase (SOD) is a group of enzymes that convert superoxide radicals into molecular oxygen and hydrogen peroxide.46 The observed decrease in SOD activity in both flies and larvae suggests that microplastic exposure disrupts their antioxidant defence system. This implies that microplastics may induce oxidative stress in Drosophila flies and larvae. One possible mechanism for this is the excessive production of reactive oxygen species (ROS) could oxidize and inactivate SOD enzymes.47 This hypothesis is supported by the results of the ROS assay conducted on flies and larvae homogenate, which revealed elevated levels of ROS in the PE MPs treatment groups. This finding aligns with previous studies conducted by Gopi et al.48 and Vutukuru et al.49 which proposed that decreased SOD activity may result from the overproduction of ROS. These excessive ROS can cause damage to the SOD enzyme by oxidizing cysteine residues within the enzyme structure.50
3.7 GST activity
The glutathione S-transferase (GST) assay conducted on Drosophila flies and larvae following exposure to polyethylene microplastics (PE MPs) at concentrations of 1 mg ml−1 and 10 mg ml−1 revealed significant alterations in GST activity, indicative of potential detoxification responses caused by microplastic exposure. GST activity (μ moles of CDNB conjugates per min per mg of protein) in the control, 1 mg ml−1 and 10 mg ml−1 flies treatment group were 0.603, 0.861 and 1.54 respectively (Fig. 3d).
In larvae, GST activity in control, 1 mg ml−1 and 10 mg ml−1 is 0.495, 0.660 and 1.139 respectively (Fig. 3d). There is a significant increase in the GST activity in the 10 mg ml−1 treatment group from both flies and larvae. Drosophila flies and larvae treated with 1 mg ml−1 displayed elevated GST activity, though this increase was not statistically significant compared to the control group. GST is a phase 2 detoxification enzyme which catalyses the conjugation of glutathione with a wide range of toxic compounds, including environmental pollutants, drugs, and carcinogens. This conjugation reaction facilitates the excretion of these toxic compounds from the organism, thereby reducing their harmful effects.51 The observed increase in GST activity in both flies and larvae upon exposure to PE MPs suggests a potential activation of the detoxification system. This response might be an attempt to eliminate or neutralize the toxic compounds associated with microplastics. Previous studies have also reported similar increases in GST enzyme activity in mussels caused by PE microplastics.52 Further Pandi et al. (2022) reported that the PE microplastic exposure increased GST activity and upregulated the GST gene in zebrafish.53
Overall, the redox stress data like ROS levels and antioxidant enzyme activities (GST and SOD) indicate the presence of redox radicals and the activation of antioxidant defence mechanisms in response to PE MP exposure in the fruit fly model. In comparison, similar outcomes have been noted in murine models reported by Kehinde S. et al., 2024. The study demonstrated pronounced levels of alterations in ROS, lipid peroxidation, and GSH levels reflecting significant cellular stress by PE MP.54 Studies in rats have demonstrated enhanced redox stress-mediated genotoxic and epigenetic effects in response to dose-dependant PE MP exposure as reported by Farag A. et al., 2023.55 Additionally, in rodent, fly and marine models, PE exposure increases reactive oxygen species (ROsS) levels, which contributes to lipid peroxidation and DNA damage.56–58 Cell-based models, such as murine fibroblasts, further highlight mitochondrial dysfunction and impaired antioxidant enzyme activity following PE exposure.59 These effects align with similar outcomes observed in studies of polystyrene and PET microplastics, indicating a broader pattern of oxidative stress across different plastic types.60–65 However, more comparative research is needed to connect these findings to human health risks directly.
3.8 Gene expression analysis
The effects of polyethylene microplastic on the gene expression of p53, reaper (rpr), and Hsp70Bc in Drosophila melanogaster after 14 days of exposure were studied. These genes play vital roles in cell death regulation, stress response, and protein folding/maintenance. Three groups were established: control, 1 mg ml−1, and 10 mg ml−1 PE MP. Gene expression levels were compared between groups.
Upregulation of the reaper (rpr) gene was observed in both the 1 mg ml−1 and 10 mg ml−1 exposure groups compared to the control (Fig. 4); however, only the 10 mg ml−1 treatment group showed statistical significance. Similar to rpr, p53 gene expression increased in flies exposed to PE MPs at both concentrations (1 mg ml−1 and 10 mg ml−1) compared to the control (Fig. 4), but statistical significance was observed only in the 10 mg ml−1 treatment group. Additionally, Hsp70Bc upregulation was only observed in the 10 mg ml−1 exposure group, not the 1 mg ml−1 group (Fig. 4).
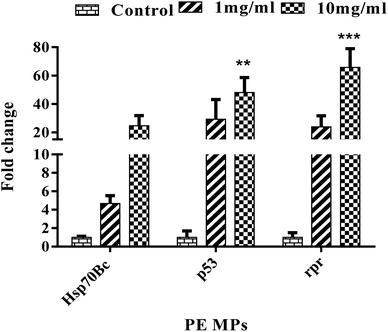 |
| Fig. 4 PE MPs alter gene expression in Drosophila. Relative mRNA expression of Hsp70Bc, p53 and rpr in adult male flies exposed to PE MPs for 14 days. The data are presented as mean ± SEM, and they were analyzed using ANOVA and Dunnett's multiple comparison test. Statistical significance was considered at p < 0.05. **indicates p < 0.01, ***indicates p < 0.001. | |
Our findings reveal that Drosophila exposed to microplastics exhibited significant upregulation of Hsp70Bc, indicating possible activation of the cellular stress response. This suggests an attempt to counteract microplastic-induced protein dysfunction and oxidative stress, consistent with similar stress responses reported for polystyrene (PS) microplastics.66 Elevated ROS levels likely drive this upregulation, potentially linked to reduced SOD activity, as both Hsp70 and SOD play crucial roles in oxidative stress management.67
Additionally, p53 expression was upregulated, reflecting the probable activation of DNA repair pathways and cell cycle arrest in response to genotoxic stress. This aligns with findings in zebrafish exposed to PS microplastics, further supporting the notion that microplastics induce DNA damage.68 The p53-driven response promotes apoptosis pathways, as indicated by the upregulation of rpr. This pro-apoptotic gene facilitates programmed cell death by inhibiting DIAP1 and activating caspase pathways, ensuring that damaged cells are eliminated to maintain tissue integrity.69 The apoptotic response observed mirrors similar stress responses reported in Drosophila larvae exposed to cadmium and DTT, suggesting that microplastic-induced oxidative and genotoxic stress triggers conserved mechanisms across different toxic exposures.70,71
Additionally, the induction of pro-apoptotic genes (e.g., rpr, hid, and grim) aligns with the commonly associated cellular strategy to eliminate damaged cells and maintain tissue homeostasis. This is particularly concerning because chronic microplastic exposure could lead to excessive apoptosis, impairing tissue regeneration or organ function.72 If similar pathways are triggered in human tissues, prolonged microplastic exposure could compromise cellular health, potentially accelerating ageing, inflammation, and disease progression. Given that oxidative stress is associated with several chronic diseases in humans, these findings highlight the importance of further studies across different models and exposure conditions. Understanding the cellular responses to microplastics is essential to assess their long-term impact on human health, particularly as microplastics accumulate in ecosystems, food chains, and human tissues.
3.9 Visualization of PE MPs in the gut of larvae
Nile red dye is used for microplastic staining because it has a high affinity for hydrophobic materials, such as plastic polymers.73 When Nile red (NR) binds to microplastics, it produces a strong fluorescence signal under 450–490 nm wavelengths of light, making the microplastics easily detectable under a fluorescence microscope.74 Our results reveal a notable distribution of PE MPs within the gut of larvae exposed to PE MPs treatment groups. Further analysis employing DAPI and DCHF-DA staining techniques indicates the presence of oxidative stress-induced cellular damage at sites of microplastic accumulation. Notably, Fig. 5 illustrates increased fluorescence intensities, reflecting the extensive accumulation of NR-stained PE MPs (red), along with evidence of subsequent nuclear damage (blue) and redox imbalance (green) within the larval gut following exposure to concentrations of 1 mg ml−1 and 10 mg ml−1. These findings strongly suggest that sites of particle accumulation experience pronounced stress and undergo cellular degradation and further serve as additional evidence for our stress-response gene expression patterns as noted in Fig. 5.
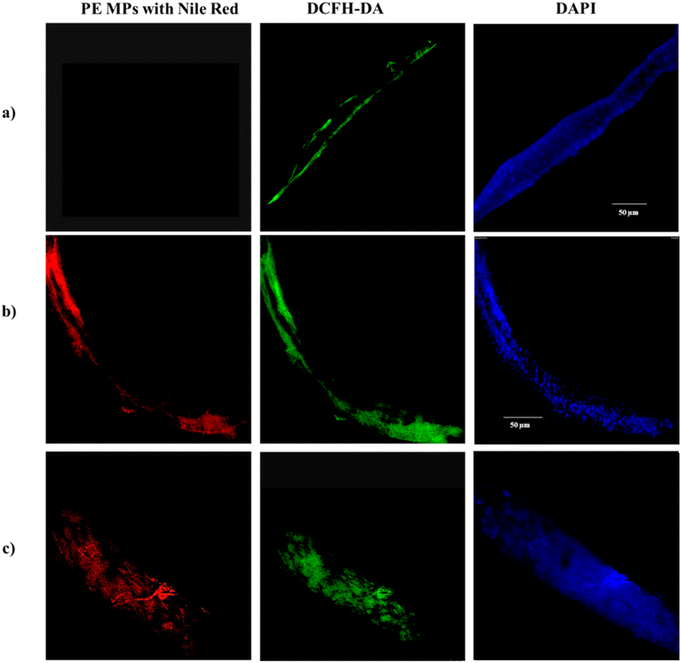 |
| Fig. 5 Fluorescent microscopic images of PE MPs in larval gut. (a) Control group larvae guts did not contain PE MPs. (b) Illustrates the Nile red stained PE MP in the gut of larvae from 1 mg ml−1 treatment group (c) Illustrates the Nile red stained PE MP in the gut of larvae from 10 mg ml−1 treatment group. | |
4 Conclusion
PE MPs at 10 mg ml−1 concentration decreased the locomotor functions at larval and adult stages, triggering redox stress factors and their associated mechanisms. In conclusion, PE MPs with size 35–50 μm induced systemic oxidative stress in a dose-dependent manner at larval and adult stages of the Drosophila melanogaster model. This inference was supported by the changes in the expression of a few vital stress response genes like rpr, p53, and Hsp70Bc and the accumulation pattern observed at the larval gut. Overall, the findings of this study highlight the detrimental effects of PE MPs on Drosophila melanogaster. The observed alterations in locomotor functions and gene expression patterns indicate the widespread impact of microplastic pollution and can easily be translated to humans, emphasizing the importance of further research into its ecological and health implications.
Data availability
All data sets used and/or generated in this work are obtainable from the corresponding author upon reasonable request.
Author contributions
Himanshu Ranjan – conceptualization, methodology, data curation, writing – original draft, writing – review & editing, software, validation; Swetha Senthil Kumar – conceptualization, methodology, data curation, writing – original draft, writing – review & editing, software, validation; Sharine Priscilla – methodology, data curation, writing – review & editing, software, validation; Subhashini Swaminathan – validation, writing – review & editing and supervision; Masakazu Umezawa – validation, writing – review & editing; Sahabudeen Sheik Mohideen – conceptualization, methodology, validation, writing – review & editing and supervision.
Conflicts of interest
The authors disclose no conflicts of interest.
Acknowledgements
The authors acknowledge the SRM-DBT platform for Advanced Life Science's support in imaging and qPCR studies.
References
-
Plastic Pollution – Our World in Data[Internet]. [cited 2024 Apr 23]. Available from: https://ourworldindata.org/plastic-pollution.
- S. U. Ain Bhutto and X. You, Spatial distribution of microplastics in Chinese freshwater ecosystem and impacts on food webs, Environ. Pollut., 2022, 293, 118494 Search PubMed.
- A. Tursi, M. Baratta, T. Easton, E. Chatzisymeon, F. Chidichimo and M. De Biase,
et al., Microplastics in aquatic systems, a comprehensive review: origination, accumulation, impact, and removal technologies, RSC Adv., 2022, 12(44), 28318–28340 Search PubMed . Available from: https://rsc.66557.net/en/content/articlehtml/2022/ra/d2ra04713f.
- H. Bouwmeester, P. C. H. Hollman and R. J. B. Peters, Potential Health Impact of Environmentally Released Micro- and Nanoplastics in the Human Food Production Chain: Experiences from Nanotoxicology, Environ. Sci. Technol., 2015, 49, 8932–8947 Search PubMed.
- P. Roy, A. K. Mohanty and M. Misra, Microplastics in ecosystems: their implications and mitigation pathways, Environ. Sci.: Adv., 2022, 1(1), 9–29 Search PubMed . Available from: https://rsc.66557.net/en/content/articlehtml/2022/va/d1va00012h.
- S. L. Wright, R. C. Thompson and T. S. Galloway, The physical impacts of microplastics on marine organisms: a review, Environ. Pollut., 2013, 178, 483–492 CrossRef CAS PubMed.
-
O. Setälä, M. Lehtiniemi, R. Coppock and M. Cole, Microplastics in marine food webs, in Microplastic Contamination in Aquatic Environments: an Emerging Matter of Environmental Urgency, Elsevier, 2018, pp. 339–363 Search PubMed.
- Y. Lu, Y. Zhang, Y. Deng, W. Jiang, Y. Zhao and J. Geng,
et al., Uptake and Accumulation of Polystyrene Microplastics in Zebrafish (Danio rerio) and Toxic Effects in Liver, Environ. Sci. Technol., 2016, 50(7), 4054–4060 Search PubMed.
- C. Zhang, J. Wang, Z. Pan, S. Wang, L. Zhang and Q. Wang,
et al., A dosage-effect assessment of acute toxicology tests of microplastic exposure in filter-feeding fish, Fish Shellfish Immunol., 2021, 113, 154–161 Search PubMed.
- J. S. Choi, S. H. Hong and J. W. Park, Evaluation of microplastic toxicity in accordance with different sizes and exposure times in the marine copepod Tigriopus japonicus, Mar. Environ. Res., 2020, 153, 104838 Search PubMed.
- K. Jabeen, B. Li, Q. Chen, L. Su, C. Wu and H. Hollert,
et al., Effects of virgin microplastics on goldfish (Carassius auratus), Chemosphere, 2018, 213, 323–332 CrossRef CAS PubMed.
- G. Malafaia, A. M. de Souza, A. C. Pereira, S. Gonçalves, A. P. da Costa Araújo and R. X. Ribeiro,
et al., Developmental toxicity in zebrafish exposed to polyethylene microplastics under static and semi-static aquatic systems, Sci. Total Environ., 2020, 700, 134867 Search PubMed.
- P. Wang, Q. Q. Li, J. Hui, Q. Q. Xiang, H. Yan and L. Q. Chen, Metabolomics reveals the mechanism of polyethylene microplastic toxicity to Daphnia magna, Chemosphere, 2022, 307, 135887 CrossRef CAS PubMed.
- R. Gautam, J. H. Jo, M. Acharya, A. Maharjan, D. E. Lee and P. B. Pramod,
et al., Evaluation of potential toxicity of polyethylene microplastics on human derived cell lines, Sci. Total Environ., 2022, 838, 156089 Search PubMed.
- A. Z. Urbisz, K. Małota, L. Chajec and M. K. Sawadro, Size-dependent and sex-specific negative effects of micro- and nano-sized polystyrene particles in the terrestrial invertebrate model Drosophila melanogaster, Micron, 2024, 176, 103560 CrossRef CAS PubMed.
- L. M. Thornton Hampton, S. M. Brander, S. Coffin, M. Cole, L. Hermabessiere and A. A. Koelmans,
et al., Characterizing microplastic hazards: which concentration metrics and particle characteristics are most informative for understanding toxicity in aquatic organisms?, Microplast. Nanoplast., 2022, 2(1), 1–16 Search PubMed . Available from: https://link.springer.com/articles/10.1186/s43591-022-00040-4.
- M. M. Monkul and H. O. Özhan, Microplastic contamination in soils: A review from geotechnical engineering view, Polymers, 2021, 13(23), 4129 Search PubMed.
- J. L. Conkle, C. D. Báez Del Valle and J. W. Turner, Are We Underestimating Microplastic Contamination in Aquatic Environments?, Environ. Manage., 2018, 61(1), 1–8 CrossRef PubMed.
- K. Oleksiuk, K. Krupa-Kotara, A. Wypych-Ślusarska, J. Głogowska-Ligus, A. Spychała and J. Słowiński, Microplastic in Food and Water: Current Knowledge and Awareness of Consumers, Nutrients, 2022, 14, 4857 Search PubMed [Internet]. Available from: https://www.mdpi.com/2072-6643/14/22/4857/html.
- S. Karbalaei, P. Hanachi, T. R. Walker and M. Cole, Occurrence, sources, human health impacts and mitigation of microplastic pollution, Environ. Sci. Pollut. Res., 2018, 25, 36046–36063 CrossRef CAS PubMed.
- N. P. Mortensen, T. R. Fennell and L. M. Johnson, Unintended human ingestion of nanoplastics and small microplastics through drinking water, beverages, and food sources, NanoImpact, 2021, 21, 100302 Search PubMed.
- K. D. Cox, G. A. Covernton, H. L. Davies, J. F. Dower, F. Juanes and S. E. Dudas, Human Consumption of Microplastics, Environ. Sci. Technol., 2019, 53(12), 7068–7074 CrossRef CAS PubMed.
-
G. U. Obuzor and U. B. Onyedikachi, Chemical Leaching into Food and the Environment Poses Health Hazards, in Modernity in Health and Disease Diagnosis: the Account from STEM Women, ed. E. O. Nwaichi, Springer Nature Switzerland, Cham, 2023. pp. 129–148, DOI:10.1007/978-3-031-34963-8_14.
-
Microplastics found in human stools for the first time | Plastics | The Guardian[Internet]. [cited 2024 Apr 23]. Available from: https://www.theguardian.com/environment/2018/oct/22/microplastics-found-in-human-stools-for-the-first-time.
- A. Ragusa, A. Svelato, C. Santacroce, P. Catalano, V. Notarstefano and O. Carnevali,
et al., NC-ND license Plasticenta: First evidence of microplastics in human placenta, Environ. Int., 2021, 146, 106274, DOI:10.1016/j.envint.2020.106274.
- S. El Kholy, K. Wang, H. R. El-Seedi and Y. Al Naggar, Dopamine modulates drosophila gut physiology, providing new insights for future gastrointestinal pharmacotherapy, Biology, 2021, 10(10), 983 Search PubMed . Available from: https://www.mdpi.com/2079-7737/10/10/983/html.
- S. Kauts, Y. Mishra, S. Yousuf, R. Bhardwaj, S. K. Singh and F. M. Alshabrmi,
et al., Toxicological Profile of Polyethylene Terephthalate (PET) Microplastic in Ingested Drosophila melanogaster (Oregon R+) and Its Adverse Effect on Behavior and Development, Toxics, 2023, 11, 782 CrossRef CAS PubMed . Available from: https://www.mdpi.com/2305-6304/11/9/782/html.
- S. El Kholy and Y. Al Naggar, Exposure to polystyrene microplastic beads causes sex-specific toxic effects in the model insect Drosophila melanogaster, Sci. Rep., 2023, 13(1), 1–11 CrossRef PubMed . Available from: https://www.nature.com/articles/s41598-022-27284-7.
- V. Sundararajan, P. Dan, A. Kumar, G. D. Venkatasubbu, S. Ichihara and G. Ichihara,
et al., Drosophila melanogaster as an in vivo model to study the potential toxicity of cerium oxide nanoparticles, Appl. Surf. Sci., 2019, 490, 70–80 Search PubMed.
- P. Dan, V. Sundararajan, H. Ganeshkumar, B. Gnanabarathi, A. K. Subramanian and G. D. Venkatasubu,
et al., Evaluation of hydroxyapatite nanoparticles - induced in vivo toxicity in Drosophila melanogaster, Appl. Surf. Sci., 2019, 484, 568–577 CrossRef CAS.
- V. Sundararajan, P. Dan, A. Kumar, G. D. Venkatasubbu, S. Ichihara and G. Ichihara,
et al., Drosophila melanogaster as an in vivo model to study the potential toxicity of cerium oxide nanoparticles, Appl. Surf. Sci., 2019, 490, 70–80 CrossRef CAS.
- S. Senthilkumar, R. Raveendran, S. Madhusoodanan, M. Sundar, S. S. Shankar and S. Sharma,
et al., Developmental and behavioural toxicity induced
by acrylamide exposure and amelioration using phytochemicals in Drosophila melanogaster, J. Hazard. Mater., 2020, 394, 122533 CrossRef CAS PubMed.
- O. H. Lowry, N. J. Rosebrough, A. L. Farr and R. J. Randall, Protein Measurement with the Folin Phenol Reagent, J. Biol. Chem., 1951, 193, 265–275 CrossRef CAS PubMed.
- F. Valéria Soares De Araújo Pinho, D. S. G. Felipe, M. G. Echeverria, K. Raquel Muller, I. Kemmerich Martins and A. P. Lausmann Ternes,
et al., Phytochemical Constituents and Toxicity of Duguetia furfuracea Hydroalcoholic Extract in Drosophila melanogaster, Evid.-Based Complementary Altern. Med., 2014, 838101 CrossRef PubMed.
- V. Sundararajan, G. D. Venkatasubbu and S. Sheik Mohideen, Investigation of therapeutic potential of cerium oxide nanoparticles in Alzheimer’s disease using transgenic Drosophila, 3 Biotech, 2021, 11(4), 159 CrossRef PubMed.
- S. Umamaheswari, S. Priyadarshinee, M. Bhattacharjee, K. Kadirvelu and M. Ramesh, Exposure to polystyrene microplastics induced gene modulated biological responses in zebrafish (Danio rerio), Chemosphere, 2021, 281, 128592 CrossRef CAS PubMed.
- S. Senthilkumar, R. Raveendran, S. Madhusoodanan, M. Sundar, S. S. Shankar and S. Sharma,
et al., Developmental and behavioural toxicity induced by acrylamide exposure and amelioration using phytochemicals in Drosophila melanogaster, J. Hazard. Mater., 2020, 394, 122533 CrossRef CAS PubMed.
- A. Sarkar, T. S. Mahendran, A. Meenakshisundaram, R. V. Christopher, P. Dan and V. Sundararajan,
et al., Role of cerium oxide nanoparticles in improving oxidative stress and developmental delays in Drosophila melanogaster as an in-vivo model for bisphenol a toxicity, Chemosphere, 2021, 284, 131363 CrossRef CAS PubMed.
-
R. Walters, J. Manion and G. G. Neely, Dissecting motor neuron disease with Drosophila melanogaster, Frontiers in Neuroscience, Frontiers Media S.A., 2019, vol. 13 Search PubMed.
- Y. Zhang, M. B. Wolosker, Y. Zhao, H. Ren and B. Lemos, Exposure to microplastics cause gut damage, locomotor dysfunction, epigenetic silencing, and aggravate cadmium (Cd) toxicity in Drosophila, Sci. Total Environ., 2020, 744, 140979 Search PubMed.
-
F. T. Demir, G. Akkoyunlu, E. Demir. Ingested polystyrene microplastics as a carrier of heavy metals (cadmium or silver): uptake, gut damage, oxidative stress, and DNA damage in Drosophila larvae Running title: Interactions between polystyrene microplastics and heavy metals in Drosophila [Internet]. Available from: https://ssrn.com/abstract=4130894.
- D. J. Liao, D. C. Lo, S. D. Macdonald, J. W. Tsai and S. Yahyavi, Effect of temperature on the negative geotaxis response in Drosophila melanogaster, The Expedition, 2012, 2 Search PubMed.
- H. Raj, A. Raj, S. Ranjan, G. Azad and S. Yasmin, Exposure of polystyrene microplastics induces oxidative stress and physiological defects in Drosophila melanogaster, Asian J. Basic Sci. Res., 2024, 06(01), 55–64 CrossRef . Available from: https://ajbsr.net/data/uploads/5268.pdf.
- M. Alaraby, A. Villacorta, D. Abass, A. Hernández and R. Marcos, The hazardous impact of true-to-life PET nanoplastics in Drosophila, Sci. Total Environ., 2023, 863, 160954 CrossRef CAS PubMed.
- I. Dubey, S. Khan and S. Kushwaha, Developmental and reproductive toxic effects of exposure to microplastics: A review of associated signaling pathways, Front. Toxicol., 2022, 4, 901798 Search PubMed.
-
P. Saxena, K. Selvaraj, S. K. Khare and N. Chaudhary, Superoxide dismutase as multipotent therapeutic antioxidant enzyme: Role in human diseases, Biotechnology Letters, Springer Science and Business Media B.V., 2022, vol. 44 Search PubMed.
- Y. Chang, L. Mao, L. Zhang, Y. Zhang and H. Jiang, Combined toxicity of imidacloprid, acetochlor, and tebuconazole to zebrafish (Danio rerio): acute toxicity and hepatotoxicity assessment, Environ. Sci. Pollut. Res., 2020, 27(10), 10286–10295 CrossRef CAS PubMed.
- N. Gopi, S. Vijayakumar, R. Thaya, M. Govindarajan, N. S. Alharbi and S. Kadaikunnan,
et al., Chronic exposure of Oreochromis niloticus to sub-lethal copper concentrations:
Effects on growth, antioxidant, non-enzymatic antioxidant, oxidative stress and non-specific immune responses, J. Trace Elem. Med. Biol., 2019, 55, 170–179 Search PubMed.
- S. S. Vutukuru, S. Chintada, K. Radha Madhavi, J. Venkateswara Rao and Y. Anjaneyulu, Acute effects of copper on superoxide dismutase, catalase and lipid peroxidation in the freshwater teleost fish, Esomus danricus, Fish Physiol. Biochem., 2006, 32(3), 221–229 Search PubMed.
- A. P. Francisco, P. H. R. Aride, A. B. de Souza, M. F. Polese, H. D. Lavander and L. C. Gomes, Toxicity of Copper in Epinephelus marginatus (Perciformes; Serranidae) After Ingestion of Contaminated Food, Bull. Environ. Contam. Toxicol., 2020, 105(5), 711–714 CrossRef CAS PubMed.
- K. Kim and J. Yim, The diverse biological functions of glutathione S-transferase omega in Drosophila, Pteridines, 2013, 24, 117–120 CrossRef CAS.
- G. Hariharan, R. Purvaja, I. Anandavelu, R. S. Robin and R. Ramesh, Accumulation and ecotoxicological risk of weathered polyethylene (wPE) microplastics on green mussel (Perna viridis), Ecotoxicol. Environ. Saf., 2021, 208, 111765 CrossRef CAS PubMed.
- P. Pandi, J. Madhuvandhi, K. K. Priya, R. Thiagarajan, S. Gopalakrishnan and S. Elumalai,
et al., Weathered polyethylene microplastics exposure leads to modulations in glutathione-S-transferase activity in fish, Front. Mar. Sci., 2022, 9, 990351 CrossRef.
- S. A. Kehinde, T. P. Fatokun, A. T. Olajide, S. M. Praveena, A. A. Sokan-Adeaga and A. P. Adekunle,
et al., Impact of polyethylene microplastics exposure on kallikrein-3 levels, steroidal-thyroidal hormones, and antioxidant status in murine model: protective potentials of naringin, Sci. Rep., 2024, 14(1), 1–10 CrossRef PubMed . Available from: https://www.nature.com/articles/s41598-024-74637-5.
- A. A. Farag, H. S. Youssef, R. E. Sliem, W. B. El Gazzar, N. Nabil and M. M. Mokhtar,
et al., Hematological consequences of polyethylene microplastics toxicity in male rats: Oxidative stress, genetic, and epigenetic links, Toxicology, 2023, 492, 153545 CrossRef CAS PubMed . Available from: https://pubmed.ncbi.nlm.nih.gov/37169321/.
- S. L. Hsieh, Y. C. Wu, R. Q. Xu, Y. T. Chen, C. W. Chen and R. R. Singhania,
et al., Effect of polyethylene microplastics on oxidative stress and histopathology damages in Litopenaeus vannamei, Environ. Pollut., 2021, 288, 117800 CrossRef CAS PubMed.
- K. Kadac-Czapska, J. Ośko, E. Knez and M. Grembecka, Microplastics and Oxidative Stress—Current Problems and Prospects, Antioxidants, 2024, 13(5), 579 CrossRef CAS PubMed . Available from: https://pmc.ncbi.nlm.nih.gov/articles/PMC11117644/.
- E. Demir and F. Turna Demir, Drosophila melanogaster as a dynamic in vivo model organism reveals the hidden effects of interactions between microplastic/nanoplastic and heavy metals, J. Appl. Toxicol., 2023, 43(2), 212–219 CrossRef CAS PubMed . Available from: https://pubmed.ncbi.nlm.nih.gov/35644834/.
- M. Herrala, M. Huovinen, E. Järvelä, J. Hellman, P. Tolonen and M. Lahtela-Kakkonen,
et al., Micro-sized polyethylene particles affect cell viability and oxidative stress responses in human colorectal adenocarcinoma Caco-2 and HT-29 cells, Sci. Total Environ., 2023, 867, 161512 Search PubMed.
- J. Shen, B. Liang, D. Zhang, Y. Li, H. Tang and L. Zhong,
et al., Effects of PET microplastics on the physiology of Drosophila, Chemosphere, 2021, 283, 131289 CrossRef CAS PubMed . Available from: https://pubmed.ncbi.nlm.nih.gov/34182651/.
- S. Bauri, H. Shekhar, H. Sahoo and M. Mishra, Investigation of the effects of nanoplastic polyethylene terephthalate on environmental toxicology using model Drosophila melanogaster, Nanotoxicology, 2024, 18(4), 354–372 CrossRef CAS PubMed . Available from: https://pubmed.ncbi.nlm.nih.gov/38958196/.
- S. Kauts, Y. Mishra, S. Yousuf, R. Bhardwaj, S. K. Singh and F. M. Alshabrmi,
et al., Toxicological Profile of Polyethylene Terephthalate (PET) Microplastic in Ingested Drosophila melanogaster (Oregon R+) and Its
Adverse Effect on Behavior and Development, Toxics, 2023, 11(9), 782 CrossRef CAS PubMed . Available from: https://www.mdpi.com/2305-6304/11/9/782/html.
- S. Kauts, Y. Mishra and M. P. Singh, Impact of Polyethylene Terephthalate Microplastics on Drosophila melanogaster Biological Profiles and Heat Shock Protein Levels, Biology, 2024, 13(5), 293 CrossRef CAS PubMed . Available from: https://www.mdpi.com/2079-7737/13/5/293/htm1.
- H. P. Liu, J. Cheng, M. Y. Chen, T. N. Chuang, J. C. Dong and C. H. Liu,
et al., Neuromuscular, retinal, and reproductive impact of low-dose polystyrene microplastics on Drosophila, Environ. Pollut., 2022, 292, 118455 CrossRef CAS PubMed (Pt B). Available from: https://pubmed.ncbi.nlm.nih.gov/34742817/.
- H. Raj, A. Raj, S. Ranjan, G. Azad and S. Yasmin, Exposure of polystyrene microplastics induces oxidative stress and physiological defects in Drosophila melanogaster, Asian J. Basic Sci. Res., 2024, 06(01), 55–64 CrossRef.
- S. Matthews, E. G. Xu, E. Roubeau Dumont, V. Meola, O. Pikuda and R. S. Cheong,
et al., Polystyrene micro- And nanoplastics affect locomotion and daily activity ofDrosophila melanogaster, Environ. Sci.: Nano, 2021, 8(1), 110–121 RSC.
- B. Xia, K. Chen, Y. Lv, D. Huang, J. Liu and G. Liang,
et al., Increased oxidative stress and plasma Hsp70 levels among gasoline filling station attendants, Toxicol. Ind. Health, 2017, 33(2), 171–181 CrossRef CAS PubMed.
- S. Umamaheswari, S. Priyadarshinee, K. Kadirvelu and M. Ramesh, Polystyrene microplastics induce apoptosis via ROS-mediated p53 signaling pathway in zebrafish, Chem. Biol. Interact., 2021, 345, 109550 CrossRef CAS PubMed.
-
A. Baonza, S. Tur-Gracia, M. Pérez-Aguilera and C. Estella, Regulation and coordination of the different DNA damage responses in Drosophila, Frontiers in Cell and Developmental Biology, Frontiers Media S.A, 2022, vol. 10 Search PubMed.
- Y. Wang, M. Misto, J. Yang, N. Gehring, X. Yu and B. Moussian, Toxicity of Dithiothreitol (DTT) to Drosophila melanogaster, Toxicol Rep, 2021, 8, 124–130 CrossRef CAS PubMed.
- P. Yang, X. Yang, L. Sun, X. Han, L. Xu and W. Gu,
et al., Effects of cadmium on oxidative stress and cell apoptosis in Drosophila melanogaster larvae, Sci. Rep., 2022, 12(1), 4762 CrossRef CAS PubMed.
- P. A. Colussi, L. M. Quinn, D. C. S. Huang, M. Coombe, S. H. Read and H. Richardson,
et al., Debcl, a Proapoptotic Bcl-2 Homologue, Is a Component of the Drosophila melanogaster Cell Death Machinery, J. Cell Biol., 2000, 148, 703–714 CrossRef CAS PubMed . Available from: http://www.jcb.org.
- A. Bianco, L. Carena, N. Peitsaro, F. Sordello, D. Vione and M. Passananti, Rapid detection of nanoplastics and small microplastics by Nile-Red staining and flow cytometry, Environ. Chem. Lett., 2023, 21(2), 647–653 Search PubMed . Available from: https://link.springer.com/article/10.1007/s10311-022-01545-3.
- M. T. Sturm, H. Horn and K. Schuhen, The potential of fluorescent dyes—comparative study of Nile red and three derivatives for the detection of microplastics, Anal. Bioanal. Chem., 2021, 413(4), 1059–1071 Search PubMed . Available from: https://link.springer.com/article/10.1007/s00216-020-03066-w.
|
This journal is © The Royal Society of Chemistry 2024 |
Click here to see how this site uses Cookies. View our privacy policy here.