Nano-TiO2 immobilized polyvinylidene fluoride based spongy-spheres for ciprofloxacin photocatalytic degradation: antibacterial activity removal, mechanisms, UVA LED irradiation and easy recovery†
Received
8th April 2024
, Accepted 25th June 2024
First published on 25th June 2024
Abstract
TiO2 is promising for the photocatalytic treatment of water contaminated with organic micropollutants. However, it is hard to recover TiO2 slurry from water. Energy-intensive separation methods are required to recover TiO2, which is a setback in the effective use of TiO2 in water treatment. Herein, we present nano-TiO2 immobilized with polyvinylidene fluoride spongy beads (TP) via simple phase inversion of the mixture of TiO2 (1%), PVDF (13%) and PVP (0.7%) for the degradation of ciprofloxacin (CIP), a micropollutant in water, under UVA LED irradiation with easy TP recovery. The stable immobilization of TiO2 with PVDF beads is attributed to interactions between Ti and F atoms. The pseudo first-order rate constant value (kobs = 0.0761 min−1) was determined for the degradation of CIP by TP. Radical scavenging, chronoamperometry, and ESR analysis revealed the presence of O2−˙, h+, HO˙ and 1O2 reactive species. LC-HRMS analysis detected ten degradation byproducts with possible degradation pathways. Importantly, the antibacterial activity of CIP against Bacillus subtilis and Escherichia coli was totally removed after 60 min treatment. TP beads were easily recovered using a simple strainer. TP was recovered and reused 30 times, and the absence of significant TiO2 leaching into water demonstrated its stable immobilization. TP photocatalysis under UVA LED irradiation proves an energy-efficient treatment method with an electrical energy per order of 24.20 kW h m−3. Overall, the study highlights a concrete way to effectively use the TiO2 photocatalyst for water treatment via immobilization using a simple phase inversion method.
Environmental significance
TiO2 is an excellent photocatalyst for water treatment. However, it is difficult to separate TiO2 slurry from water, which hinders TiO2 recovery and its effective use. Herein, we demonstrate that TiO2 immobilized with PVDF (TP) beads suspended in water exhibits excellent photocatalytic degradation of ciprofloxacin (CIP), a micropollutant of major concern, promoted under energy-efficient UVA LED irradiation. Further, the antibacterial activity of CIP was removed after treatment, highlighting the potential of TP photocatalytic treatment to prevent the risk of antimicrobial-resistant bacteria spreading into the environment due to antibiotics in water. Importantly, easily suspended TP beads were recovered by a simple strainer and reused several times. The study reveals the effective use of nano-TiO2 for water treatment by its immobilization with PVDF beads.
|
1. Introduction
Organic micropollutants in water are a major concern as they have potential to cause adverse effects on the environment and health.1 Importantly, the release of antibiotic micropollutants into water has the risk of leading to the emergence of antibiotic-resistant bacteria (ARB).2 Ciprofloxacin (CIP) is one of the largely consumed antibiotics across the world.3 It is mainly used in the treatment of respiratory and urinary tract infections; its incomplete metabolism in the body leads to its large discharge into wastewater and its spread into the environment.3 CIP belongs to the antibiotic class fluoroquinolones.3 It has been reported that quinolones are highly non-biodegradable and lead to accumulation in water, thus posing a risk of the spread of ARB.3
Advanced oxidation processes (AOPs) have acquired utmost attention owing to their ability of removing organic micropollutants from water through the in situ generation of reactive oxygen species, primarily hydroxyl radicals (HO˙).4 Photocatalysis-based AOPs have been widely explored for water treatment.5 TiO2 is one of the majorly explored photocatalysts for water treatment owing to its abundance in the earth's crust, chemical stability, excellent activity, suitable energy band positions, nontoxicity, and low cost.6 Importantly, TiO2-based AOPs are known to oxidize organic micropollutants due to the highly reactive HO˙ together with other oxidative species such as super oxide radicals (O2−˙) and photogenerated holes (h+).7 TiO2-based AOPs have the ability to be promoted by energy-efficient LED sources.8 Moreover, surface adsorption leading to interaction between contaminants and TiO2 for degradation has been reported.5 Nevertheless, it is important to note that TiO2 suspension is highly stable.9 Slurry-type photocatalytic systems suffer from the drawback of poor light penetration throughout the photocatalytic chamber due to scattering and inner filter effects and require careful optimization of the initial catalyst dose.9 Also, the requirement for energy intensive and costly filtration setups for the recovery of the TiO2 catalyst post catalysis is a constraint.9 Therefore, the recovery of the TiO2 photocatalyst remains a challenge and needs to be well explored.
On the other hand, polyvinylidene fluoride (PVDF) is a well explored membrane material used for water treatment.10 PVDF is largely used in membrane water treatment systems due to its great chemical stability and higher mechanical strength.11,12 Additionally, PVDF, being inert to ultraviolet radiations, has the advantage to be used along with TiO2 for UVA-based photocatalytic water treatment systems.12 Earlier, TiO2-PVDF systems were mainly studied as membrane films for photocatalysis that still require the energy-consuming pressurized filtration step.13,14 Herein, we propose TiO2 immobilization with PVDF beads by the simple phase inversion technique. The proposed TiO2-immobilized polymeric beads may have dynamic movement throughout the photocatalytic chamber, effective light exposure, and easy recovery without any energy-intensive processes. Earlier, TiO2 immobilized with beads made up of alginate,15 modified-alginate,16,17 cellulose aerogels,18 SiO2
19-based bead, via cross-linking15–18 and adsorption techniques19 have been reported. Further, the photocatalysis with these reported TiO2 immobilized beads were studied using conventional light sources like mercury and xenon lamps that consume large electrical energy. Moreover, their stability and evaluation of recoverability for the effective use of photocatalysts need to be well studied.
Based on the above facts, for the first time, we aimed to synthesize TiO2-PVDF polymeric beads (TP) by the simple phase inversion technique and investigate its ability to degrade ciprofloxacin (CIP) antibiotic micropollutant under UVA LED irradiation and photocatalyst recovery. The beads were characterized by different techniques, such as FESEM-EDX, XRD, IR, UV-DRS, XPS, and BET. Different parameters such as the effect of TiO2 loading in TP polymeric beads, effect of the hydrophilicity inducing agent PVP, and the pH effect on the photocatalytic degradation efficiency were presented. The recovery of TP beads by a simple strainer was discussed. The recyclability of the TP beads along with ICP-AES analysis was performed to determine the stability of the proposed beads. Radical scavenging, ESR and electrochemical results were obtained, and a degradation mechanism was proposed. The results of LC-HRMS analysis and antibacterial assay before and after treatment were presented. Further, the electrical energy per order (EEo) value was determined.
2. Materials and methods
2.1. Reagents
Ciprofloxacin hydrochloride hydrate (CIP) and titanium dioxide nanopowder (TiO2) were obtained from SRL Pvt. Ltd., India. Polyvinylidene fluoride (PVDF) was obtained from Kynar HSV 900, Arkema, polyvinylpyrrolidone (K85-95) (PVP) was obtained from Acros Organics (Fisher scientific), sodium chloride, sodium sulfate, sodium hydroxide, sulfuric acid, iron(III) nitrate nonahydrate, sodium hydrogen carbonate, sodium hydrogen phosphate, and humic acid were obtained from Qualigens, Thermo Scientific. tert-Butanol (TBA) (AR, 99%), methanol (MeOH) (HPLC Gradient Grade, 99.9%), and acetonitrile (ACN) (HPLC Gradient Grade, 99.9%) were purchased from Finar, India. Standard pH solutions (pH = 4.01, 7.00, 10.01) obtained from Thermo Scientific were used for calibration of the pH meter. Dimethyl-1-pyrroline-N-oxide (DMPO), 1-methyl-2-pyrrolidone (NMP), and 2,2,6,6,-tetramethylpiperidine (TEMP) were purchased from Tokyo Chemical Industry Co., Ltd. (TCI), India. 2,2,6,6-tetramethylpiperidine-1-oxyl (TEMPO) was purchased from Sigma Aldrich. The solutions were prepared in deionized water.
2.2. Synthesis of polymer beads
0.9 g of PVDF (13% w/v) was dissolved in 7 mL NMP solution along with 0.05 g of PVP (0.7% w/v) and stirred for 8 hours at 60 °C, as reported in the earlier literature, to achieve a homogenous slurry of PVDF.10 The polymer slurry was degassed for 12 hours in a vacuum desiccator. The degassed solution was taken in a syringe and dropped at 10 cm height into water to achieve spherical polymeric beads by phase inversion. These beads were designated as PP beads. Beads synthesized without PVP were labeled as PVDF beads. The average diameter of the beads was measured to be ∼2 mm.
2.3. Synthesis of polymer beads with TiO2
To obtain polymeric beads with TiO2, an appropriate amount of TiO2 was taken in a 10 mL NMP and sonicated for 30 min to achieve a homogeneous suspension.10 Then, this sonicated solution was added to the PP polymer slurry (as mentioned in the above section) with constant stirring at 60 °C. The whole slurry was further stirred for 4 hours at 60 °C. The mixture was subjected to degassing in a vacuum desiccator for 12 hours. The degassed solution was dropped into water by a syringe to form TP beads via phase inversion. TP beads with different loading amounts of TiO2 (1%, 5%, 10% and 15%) were synthesized. TP beads with 1% TiO2 loading with different amounts of PVP (0.35, 0.7, 1.4, 2.1 and 2.5) additive were also synthesized.
2.4. Characterization
Scanning electron microscopy (SEM) and energy dispersive X-ray (EDX) analysis were carried out using a Quanta FEG 250 (FE-SEM) equipped with an area mapping spectrometer (EDX). A LEICA EM ACE 200 sputter coater was used for sputtering the sample for surface analysis and elemental composition. Transmission electron microscopy (TEM) images were acquired using a JEOL model JEM-F200/F2 Multi-purpose Electron Microscope (200 kV). Samples for TEM analysis were prepared by drop casting the dispersion of sonicated TiO2 nanopowder ethanolic solution over carbon-coated copper grids and drying under ambient atmosphere. The surface area, pore volume and size distribution were analyzed by the Brunauer–Emmett–Teller (BET) method using an Anton Paar Quantachrome NOVA 1000e through the nitrogen adsorption–desorption method. The structural properties of the PVDF beads and TiO2 were determined by powder X-ray diffraction (XRD) using a Bruker D8 Advance instrument. The analysis was acquired from 2θ = 10–90 at 5° min−1 scanning speed with Cu Kα radiation (λ = 1.5405 Å). The IR spectra were recorded using a Shimadzu FT-IR spectrometer. X-ray photoelectron spectroscopy (XPS) analysis was carried out to identify the surface composition, chemical state of the elements and the interaction between the catalyst and polymer matrix using a K-alpha Thermo Fisher Scientific instrument. A Shimadzu UV-2450 spectrometer was used for the measurement of the UV-visible diffuse reflectance spectra (UV-DRS) for the solid samples with BaSO4 reference in the 200 to 800 nm range. The band gap of TiO2 in TP beads was calculated as per the Tauc eqn (1).20where α is the absorption coefficient, hν is the energy of incident photon, A is the proportionality constant, n = ½ for direct allowed bandgap, and Eg is the band gap.20 The band gap was predicted from the extrapolation of the linear portion in the graph of (αhν)2vs. hν.20
A JEOL Electron spin resonance (ESR) spectrometer was used to record the ESR spectra. Spin trapping agents such as DMPO, TEMPO and TEMP were used for the analysis. The microwave frequency = 9.440 GHz, microwave power = 0.995 mW, and magnetic centre field = 336 mT condition were used. Samples were withdrawn at appropriate time intervals and analysed.
Inductively Coupled Plasma-Atomic Emission Spectrometry (ICP-AES) analysis was carried out using a SPECTRO ARCOS simultaneous ICP spectrometer. The instrument had a minimum detection limit of 10 ppb (μg L−1) for titanium (Ti). The TP beads were separated from water after photocatalysis using a simple strainer, and the water sample was analysed by ICP-AES.
Electrochemical analysis was performed using a BioLogic SP-150e electrochemical workstation. A three-electrode electrochemical cell was used for chronoamperometric measurements and electrochemical impedance spectroscopy (EIS) for constructing the Mott–Schottky and Nyquist plots. The Mott–Schottky plots were obtained by EIS measurements from −1.0 V to 0 V against Ag/AgCl reference electrode with frequency in the range from 100 kHz to 10 Hz. For the photocatalyst-working electrode, the TiO2 slurry was prepared in water–ethanol mixture using alcoholic Nafion as a binder and coated over fluorine-doped tin oxide (FTO) glass slides over an area of 1 cm2. In case of TP, the coating slurry was prepared using NMP as the solvent. The reference electrode was Ag/AgCl (saturated KCl) electrode and graphite was used as the counter electrode. 15 mL of 0.1 M Na2SO4 electrolyte solution and 367 nm LED light source was used for all the photoelectrochemical experiment.
The porosity (ε) of the prepared beads was measured by gravimetric analysis (eqn (2)).21 The water holding capacity can be related to the free volume available over the bead surface and affinity towards water molecules.
| 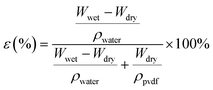 | (2) |
where,
Wwet and
Wdry is the weight of wet and dry bead, respectively, and
ρwater (0.998 g cm
−3) and
ρpvdf (1.785 g cm
−3) are the density of water and PVDF, respectively.
2.5. Photocatalysis
All the photocatalysis experiment were performed in deionised water. In a typical reaction, 50 mL of water was spiked with 1 ppm (1 mg L−1) CIP and appropriate number of TP beads were added in the solution. An ultraviolet A light emitting diode (UVA LED) with maximum wavelength (λmax) at 367 nm was used as the light source. A UVA LED light source was placed at a distance of 5 cm over the water surface for uniform irradiation with an average irradiance of 3.5 mW cm−2. A stock solution of 100 ppm (100 mg L−1) of CIP was used for all the analysis. For studying the pH effect, 0.1 N HCl and 0.1 N NaOH were used to adjust the pH. In real water matrix analysis, municipality tap water and simulated ground water were used (SGW). The characteristics of municipality tap water are provided in Table S1.† The SGW was prepared as per an earlier report.22 The composition of SGW was as follows: Fe (NO3)3·9H2O = 0.24 μM (0.096 mg L−1), NaHCO3 = 1.2 mM (100 mg L−1), Na2SO4 = 0.34 mM (48.29 mg L−1), Na2HPO4 = 0.28 mM (40 mg L−1), NaCl = 0.86 mM (50 mg L−1), humic acid = 10.0 mg L−1.22
The degradation of CIP was monitored by high performance liquid chromatography (HPLC); the HPLC method conditions are discussed in Text S1.† 0.5 mL sample aliquot was collected at 10 min intervals and injected in the HPLC instrument. The degradation byproducts were screened using a high resolution mass spectrometer (LC-HRMS-ToF). The method conditions are described in Text S2.† 2 mL sample aliquot was withdrawn after 60 min of photocatalysis reaction and used for analysis. A EUTECH pH 700 meter was used to measure the solution pH. For the radical scavenging experiment, benzoquinone (BQ), ethylenediaminetetraacetic acid disodium salt (EDTA), sodium azide (NaN3), and tert-butyl alcohol (TBA) were used for the scavenging of the superoxide radical (O2−˙), photogenerated holes (h+), singlet oxygen (1O2), and hydroxyl radical (HO˙), respectively.23 10 mM scavengers were spiked in the reaction mixture for the studies.
2.6. Antibacterial assay
The antibacterial activity of CIP was determined by the agar well diffusion method as per Clinical and Laboratory Standards Institute (CLSI)24 before and after the treatment with different time intervals by TP beads as per the standard protocol.24 The Gram-positive Bacillus subtilis and Gram-negative Escherichia coli bacterial strains were used to evaluate the antibacterial activity of CIP. 90 mm sterile petri dishes were used to prepare plates with 22 mL of nutrient agar to yield 4 mm depth media. The standard spread-plate technique employed 100 μL of inoculum suspension on the solid media plates. Round wells (8 mm in diameter) were punched into agar plates using a sterile well-borer. The wells were filled with 75 μL of the samples using a sterile micropipette. These plates were incubated for 24 h at 37 °C and the inhibition zone (mm) around the well was measured. Experiments were repeated three times and the standard error was calculated.22
3. Results and discussion
3.1. Characterization of polymer beads
3.1.1. SEM–EDX analysis of polymeric beads.
The SEM image of the spherical PP bead reveals the average diameter to be about 2 mm (Fig. 1a). Further, 1000× magnification images reveal that the surface of the bead is porous in nature (Fig. 1b). The cross-section image reveals pores on the surface and channels and large voids spaces inside the bead; overall, the bead has a sponge-like morphology (Fig. 1c). The internal void space is much greater than the surface pore openings (Fig. 1b and d). Earlier, porous PVDF membrane flat sheet with large internal voids, as observed in the present study, was reported.25 The SEM images of the whole TP bead and its corresponding cross-sectional image (Fig. 1e–h) were very similar to that of the PP bead (Fig. 1a–d). The SEM images for beads with different TiO2 loading reveals no significant change in the morphology of the beads (Fig. S1†). Importantly, the SEM-EDX image results clearly reveal that TiO2 was partially distributed on the surface of the beads as well as in the internal void spaces (Fig. S2†). Moreover, the SEM images reveal that the addition of PVP, a known hydrophilic polymer additive, induced more surface pores, leading to a rougher surface of the TP beads (Fig. S3†).26,27 Additionally, the distribution of TiO2 nanoparticles on the surface of the TP beads were not affected by PVP loading, as revealed by SEM EDX analysis (Fig. S4†). Further, a magnified SEM image reveals that TiO2 has a grain-like morphology with an average partial size of 25 nm that was agglomerated into large irregular clusters, and similar features of TiO2 were seen in TP beads (Fig. S5a† and 1f and h). Further, the TEM image reveals the grain-like morphology of TiO2 nanoparticles (Fig. S5b†). Thus, all the above results reveal the immobilization of TiO2 with the PVDF sponge beads.
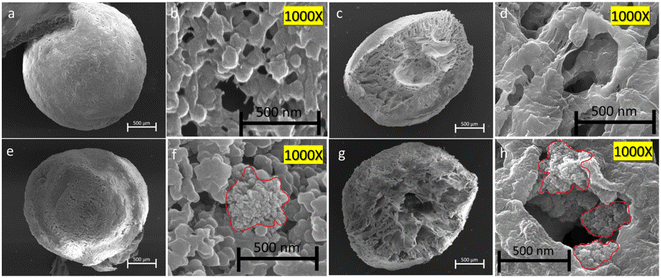 |
| Fig. 1 SEM images of the surface and corresponding cross-section of beads with the respective high magnification images (1000×). PP (a–d) and TP (e–h). TP = 1% TiO2 with PVDF having 0.7% PVP; PP = PVDF having 0.7% PVP. | |
3.1.2. BET analysis and porosity determination.
The surface area, total pore volume, and pore diameter of PVDF, PP, TP beads and TiO2 are summarized in Table S2.† The surface area of PVDF and PP beads (with and without the addition of PVP, a hydrophilic additive) was 2.7550 m2 g−1 and 4.1065 m2 g−1, respectively.26,27 The TP beads had a higher surface area of 8.6374 m2 g−1 compared to that of PVDF beads (2.7550 m2 g−1). The higher surface area was attributed to the contribution of the immobilized high-surface TiO2 nanoparticles (77.4609 m2 g−1) on the surface of the beads (Table S2†). Further, the porosity (%) measured by the gravimetric method for the beads was summarized (Table S2†). These results reveal that the porosity was not significantly affected by the immobilization of TiO2, indicating the TP may exhibit effective mass transfer needed for the photocatalytic degradation of pollutants.
3.1.3. XRD analysis.
The X-ray diffraction patterns of the TiO2, PP, and TP beads were recorded (Fig. 2a). The XRD pattern of TiO2 displayed peaks at 2θ = 25.4°, 37.0°, 37.9°, 38.6°, 48.1°, 54.0° and 55.2° corresponding to the (101), (103), (004), (200), (105), (211), and (204) planes, respectively, revealing the anatase phase of TiO2.28 In case of PP, the peaks at 2θ = 18.5°, 20.4° and 26.4° were attributed to PVDF. The reflection at 18.5° corresponds to the γ (020) plane. The reflection at 20.4° corresponds to (200) and (110) reflections of β overlapped with (021) of the γ phase.29 The reflection at 26.4° represents the (021) plane of the α phase of PVDF. The XRD pattern of TP displayed characteristic peaks of TiO2 and PVDF polymer with no major shift in the 2θ values.28 The crystallite size of TiO2 calculated using the Scherrer's equation (Text S3†) was found to be 20.9 nm for TiO2 and 19.7 nm for TP. Thus, the XRD results reveal that the immobilized TiO2 in the TP bead had a structure similar to the parent TiO2 in the anatase form.
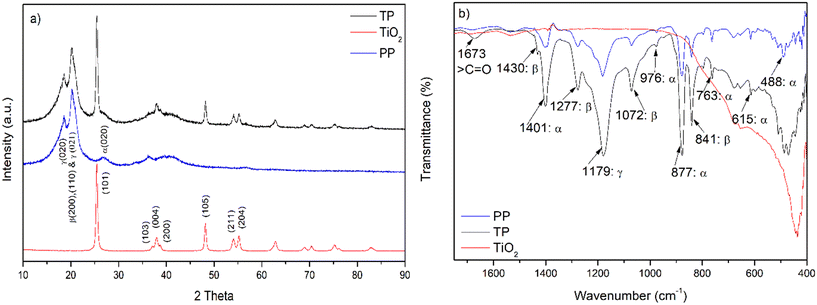 |
| Fig. 2 a) XRD pattern of TiO2, PP and TP beads. b) IR spectra of TiO2, PP and TP beads. TP = 1% TiO2 with PVDF having 0.7% PVP; PP = PVDF having 0.7% PVP. | |
3.1.4. IR analysis.
The IR spectral results for TiO2, PP and TP are presented in Fig. 2b. The IR spectrum of TiO2 shows characteristic strong intense peak at about 420 cm−1 to 490 cm−1 for the Ti–O stretching frequency.30 In case of PP, the IR peaks at 488 cm−1, 615 cm−1, 763 cm−1, 877 cm−1, 976 cm−1, and 1401 cm−1 resulted from the α phase of the PVDF.31 The peaks at 841 cm−1, 1072 cm−1,1277 cm−1 and 1430 cm−1 correspond to the β phase. The γ phase is represented by the peak at 1179 cm−1.31 TP shows a broad Ti–O peak in the range from 420 cm−1 to 490 cm−1. The peak with maximum intensity at 1673 cm−1 represents the pyrrolidone carbonyl stretching frequency of the PVP molecule.32 Importantly, the ratio of β (at 841 cm−1) and α (at 763 cm−1) peaks observed in PVDF was found to be enhanced after the immobilisation of TiO2 in TP, revealing the interaction between TiO2 and PVDF. Earlier, a similar observation was reported for the TiO2 immobilized with PVDF membrane in the flat sheet form.33 Further, the possible interaction between TiO2 and PVDF was investigated by XPS analysis, as discussed below.
3.1.5. XPS analysis.
The XPS spectra of the TiO2 core level Ti 2p showed two peaks at 459.55 eV and 465.16 eV corresponding to Ti 2p3/2 and Ti 2p1/2, respectively (Fig. 3a). In TP, the Ti 2p3/2 and Ti 2p1/2 peaks were observed at 458.68 eV and 464.41 eV, respectively. The shifts in the Ti 2p3/2 and Ti 2p1/2 peaks of TP compared to TiO2 indicate the interaction between Ti and the polymer matrix.34 Further, PP showed a single peak of F 1s at 688.08 eV corresponding to the C–F bond of PVDF (Fig. 3b). Importantly, the C–F peak in case of TP beads was also observed. However, the F 1s peak position was at 687.96 eV (Fig. 3b), corresponding to a shift of 0.12 eV compared to the PP beads. These observations reveal the interaction between Ti and F in TP. Interestingly, a small hump at about 684.22 eV was observed, which is attributed to Ti–F interaction.34 Similar results were observed in case of PVDF-modified TiO2 prepared via the chemisorption of PVDF on to TiO2.34 The O 1s spectra showed peaks at 530.73 eV and 532.45 eV for TiO2 resulting from Ti–O–Ti and O–H bonding, respectively. The peaks at 531.61 eV and 532.82 eV were attributed to functional groups C–O/C
O and O–H, respectively (Fig. 3c).35 The O–H was mainly because of the trapped water molecules during the phase inversion of the polymer into beads (Fig. 3c). The Ti-O-Ti peak of TP was shifted by 0.9 eV compared to TiO2, confirming the strong influence of the highly electronegative F atom on the Ti atom.34 The C 1s spectra of PP showed peaks at 285.36 eV, 286.52 eV, 287.64 eV and 291.02 eV for the C–C, C–H, C–O and C–F bonds, respectively (Fig. 3d).35 In the case of TP, the C 1s peaks were identified with no major shift in the signals compared to the PVDF beads. The XPS survey spectra for TiO2, PP and TP are presented in Fig. S6.† Overall, XPS analysis clearly reveals the immobilization of TiO2 with PVDF and the interaction of Ti and F atoms.
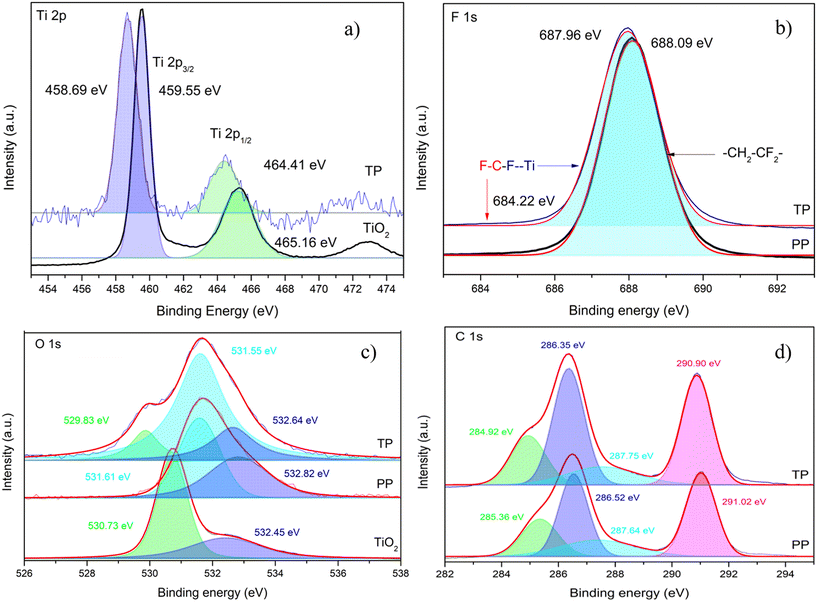 |
| Fig. 3 XPS spectra of the core levels: a) Ti 2p, b) F 1s, c) O 1s, d) C 1s for TiO2, PP and TP. TP = 1% TiO2 with PVDF having 0.7% PVP; PP = PVDF having 0.7% PVP. | |
3.1.6. Absorption and electrochemical characterization.
The band gap of TiO2 and TP was determined to be 3.24 eV and 3.20 eV, respectively (Fig. S7(a)†). No major difference in the band gap reveals that immobilized TiO2 has an electronic distribution similar to free TiO2. Further, the emission spectra of the UVA LED lamp with an emission maximum at 367 nm (corresponding to 3.37 eV) overlaps with the TP absorption spectrum (bandgap (Eg) = 3.26 eV), revealing that UVA LED with 367 nm wavelength is suitable for the promotion of TP photocatalysis (Fig. S7(b)†).23 The flat band potentials obtained from the Mott–Schottky plot for the free TiO2 (−0.702 V vs. Ag/AgCl) and immobilized TiO2 (0.710 V vs. Ag/AgCl) were found to be almost similar (Fig. S8†).36 Additionally, the Nyquist plot showed a similar arc radius, implying no major difference in the electron transfer ability of the TiO2 bound to PVDF beads in comparison to free TiO2 (Fig. S9†).35,36
3.2. Photocatalysis
3.2.1. Photocatalytic degradation of CIP by TP under UVA LED irradiation.
The CIP molecule was nearly stable under only UVA light irradiation, even after 60 min of photolysis (Fig. 4a). Further, the adsorptive removal of CIP by PP and TP beads was not effective, less than 45% after 60 min of dark incubation. Importantly, only in the case of CIP molecule irradiated under UVA light in the presence of TP caused almost complete degradation within 60 min.9,17 The pseudo first-order rate constant (kobs) for the degradation of CIP by TP under UVA irradiation was determined to be 0.0761 min−1.37
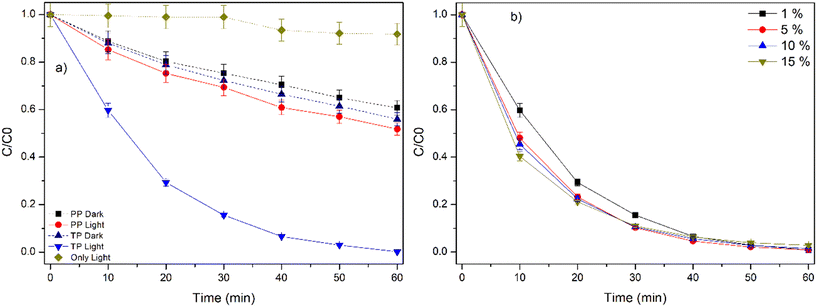 |
| Fig. 4 a) Degradation of CIP by TP under UVA LED irradiation, TP = 1% TiO2 with PVDF having 0.7% PVP, PP = PVDF having 0.7% PVP (control), b) effect of TiO2 loading on the degradation of CIP by TP under UVA LED irradiation. Experimental conditions: [CIP] = 1 ppm, number of TP beads = 100, TP = 1%, 5%, 10% and 15% TiO2, reaction volume = 50 mL. | |
3.2.2. Effect of catalyst loading.
TP with different TiO2 loading (1%, 5%, 10% and 15%) showed no significant difference in the degradation efficiency (Fig. 4b). As discussed in the earlier sections, the cross-sectional SEM images and the corresponding SEM–EDX analysis of the different TP beads revealed the occupancy of TiO2 inside the large voids of TP beads as the amount of TiO2 increased (Fig. S1 and S2†). These facts indicate that the available surface exposed TiO2 were similar, although the TiO2 loading was varied, and accounted for no change in the kobs values. Similar results were reported in the case of TiO2-gC3N4/PVDF flat sheet membranes38 and KCN/alginate beads39 as the catalyst loading was increased.
3.2.3. Effect of PVP.
The effect of PVP strength over the photocatalytic efficiency of the TP beads is presented in Fig. S10.† The PVP strength was varied from 0% to 2.8% in the TP bead synthesis. At 0 PVP, lower photocatalytic efficiency was noted, with incomplete degradation (90%) at 60 min. Upon increasing the PVP loading in the range from 0.35% to 1.4%, the degradation efficiency gradually increased. Further, at higher PVP loading of 2.1% and 2.8%, a minor reduction in the photocatalytic activity of the TP beads was observed. These results indicate that the appropriate loading of PVP is important. Earlier, PVP has been used as an additive in the phase inversion of PVDF to induce rough surface and hydrophilicity, which facilitates proper mass transfer and induces efficient photocatalysis.40 Upon increasing the PVP content, rough surface and enhanced surface pores were observed (Fig. S3†). Nevertheless, the results emphasize that a higher loading (about 2.1%) may cover the TiO2 nanoparticles distributed on the surface pores and retard the photocatalytic efficiency.38
3.2.4. Effect of the initial concentration of CIP.
The influence of the initial concentration of CIP is presented in Fig. 5a. As the concentration of CIP is increased from 1 ppm to 5 ppm, there is a reduction in the photocatalytic efficiency. The pseudo first order rate (kobs) value decreases exponentially from 0.0761 min−1 to 0.0282 min−1 with the increase in the initial concentration of CIP (1–5 ppm) (Table S3,†Fig. 5b). This is mainly due to the limited availability of the active sites of the photocatalyst in comparison to the higher initial concentration of CIP, adsorption of more CIP over the photocatalyst beads, thereby blocking the active site and reducing the photogenerated reactive species.41 Further, the possible inner filter effect at higher CIP concentration, which hinders the effective penetration of light into the photocatalytic reaction chamber, may also retard the reaction.41
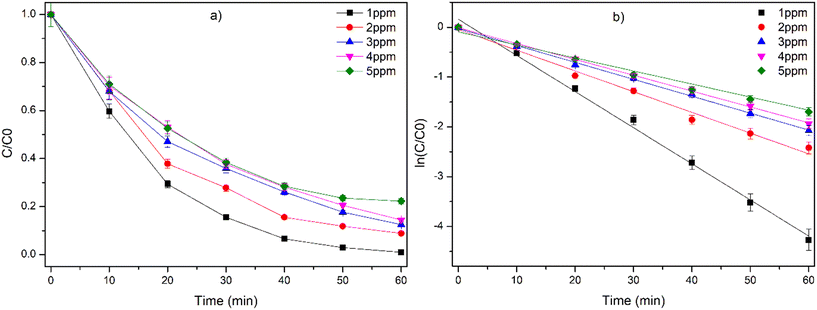 |
| Fig. 5 a) Effect of initial concentration of CIP on the degradation by TP under UVA LED irradiation. b) Graph of ln(C/C0) vs. time for the degradation of CIP. Experimental conditions: number of TP beads = 100, TP = 1% TiO2, 0.7% PVP with PVDF, and reaction volume = 50 mL. | |
3.2.5. Effect of pH on the degradation efficiency of PVDF polymer beads.
In the degradation of organic pollutants by photocatalysis, pH plays a vital role as the solution pH influences the surface charge of the catalyst as well as charge on the target pollutant.41 The degradation of CIP at different pH values (3–10) was performed and is presented in Fig. 6a. The CIP degradation efficiency was retarded to 55% at highly acidic and basic pH 3 and 10, in comparison to pH = 7 (100%). The degradation efficiency was retarded to 65% and 86% at pH = 4 and 8, respectively. The pKα value of CIP was reported to be 5.90 and 8.89 (Fig. 6b). Below pH 5.9, the CIP molecule is positively charged and above 8.89, it is negatively charged.41 At higher pH (pH = 10), the surface charge of TiO2 and overall charge of CIP molecule is negative, resulting in repulsion and lowering of the interaction of the CIP molecule and the catalyst.41 Additionally, ICP-AES analysis showed the leaching of Ti from TP (0.438 ppm = 0.24% at pH = 10), thus indicating the weakening of the coordination bond of Ti-F under the influence of alkaline pH. Similarly, at lower pH, the catalyst and CIP molecule possess a positive charge, leading to electrostatic repulsion, which affects the degradation efficiency.41 Importantly, there was no leaching of TiO2 under acidic and neutral conditions. All these results highlight that near neutral pH is suitable for the degradation of CIP by TP beads.
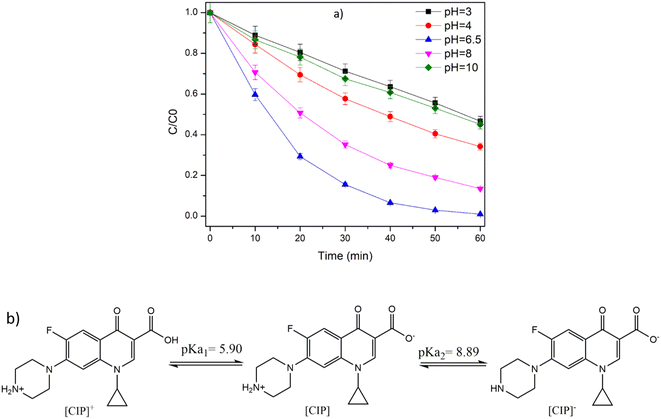 |
| Fig. 6 a) Effect of initial pH on the degradation of CIP by TP under UVA LED irradiation. Experimental conditions: number of TP beads = 100, TP = 1% TiO2, 0.7% PVP with PVDF, reaction volume = 50 mL, and b) zwitterionic form of CIP. | |
3.2.6. Role of reactive species in the degradation of CIP..
TiO2 under UVA irradiation (367 nm) is known to generate photoexcited hole (h+) and electrons (e−).8 In the presence of p-BQ, a O2−˙ quencher, the degradation efficiency was reduced to 48% (Fig. 7a). This result explains the significant role of O2−˙ in the degradation of CIP.42 The photogenerated electrons in TiO2 react with surface adsorbed O2 and undergo photoreduction to generate O2−˙. The CB of TiO2 is reported to be about (−0.55 V), which is more negative compared to the redox potential of O2/O2−˙ (−0.16 V vs. RHE).23 Further, EDTA was also found to retard the degradation efficiency to 61%. This emphasises the role of photogenerated holes (h+) in the degradation of CIP.42 Additionally, in the presence of NaN3 and TBA, the degradation efficiency of 80% and 88% was observed, indicating no major role of 1O2 and HO˙ in the degradation of CIP by TP beads.43 From the quenching experiment, the order of quenching for the reactive species is O2−˙ > h+ ≫ 1O2 > HO˙.
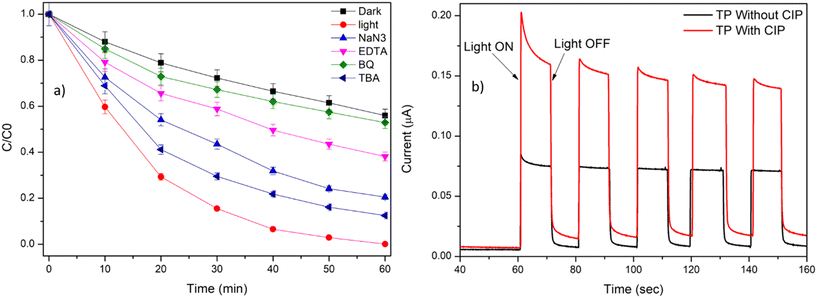 |
| Fig. 7 a) Radical scavenging experiment for the degradation of CIP by TP beads. Experimental conditions: [CIP] = 1 ppm, [TBA] = [p-BQ] = [EDTA] = [NaN3] = 10 mM, number of beads: 100, and TP = 1% TiO2 with PVDF having 0.7% PVP. b) Photocurrent-time dependence curve. Experimental condition: 10 s ON–OFF cycle, voltage = 0.2 V against Ag/AgCl electrode, electrolyte = 0.1 N Na2SO4, and [CIP] = 1 ppm. | |
The photocurrent response with and without the addition of CIP in the electrolyte solution is measured (Fig. 7b). The increase in the photocurrent response was observed in the presence of CIP molecule. These results further emphasise the oxidation of CIP molecule by the photogenerated h+ at the VB of TiO2, thereby inhibiting the electron–hole recombination and increase the photocurrent response.23
Additionally, the generation of the reactive radicals was confirmed by ESR analysis using DMPO, TEMPO and TEMP as the trapping agents. DMPO in the presence of methanol (MeOH) presented the DMPO–O2−˙ signal, confirming the in situ generation of O2−˙ radical during the photolysis of TP beads (Fig. 8a).23,43 In the DMPO-water system, prominent DMPO–HO˙ adduct with intensities of 1
:
2
:
2
:
1 was observed, representing the generation of HO˙ species by TP beads (Fig. 8b).22,43 TEMPO has a triplet signal in the ESR spectrum due to its paramagnetic nature, and the reduction of the signal intensity during photolysis is attributed to the presence of photogenerated holes (h+), which oxidizes the TEMPO (Fig. 8c).43 In the presence of TEMP, singlet oxygen (1O2) probe, triplet signals of equal intensity were observed, depicting the 1O2 generation by the TP beads under UVA irradiation (Fig. 8d).43
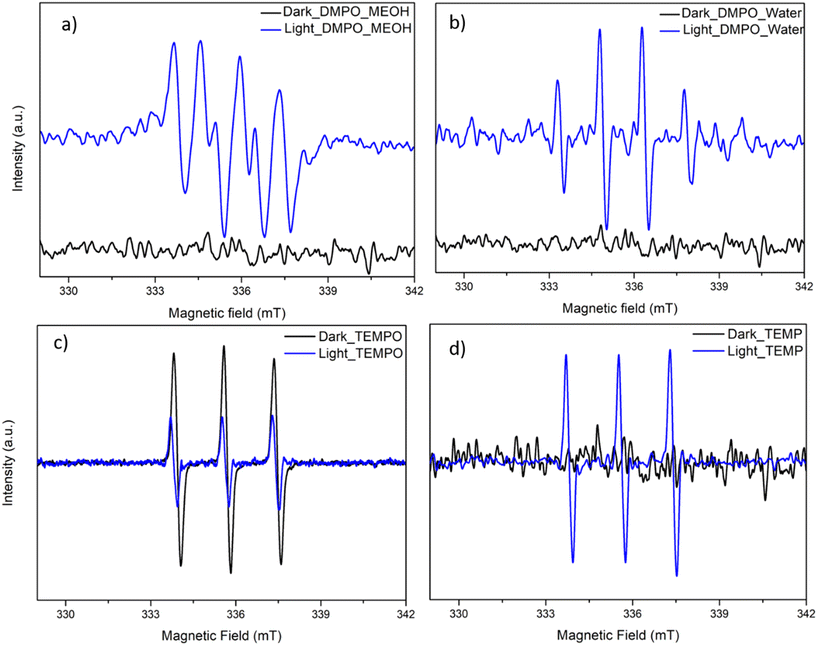 |
| Fig. 8 ESR spectra of (a) DMPO–O2−˙, (b) DMPO–HO˙, (c) TEMPO–h+, and (d) TEMP–1O2 for the light and dark experiment. [TP] = 100 bead/50 mL, [DMPO] = [TEMPO] = [TEMP] = 30 mM, TP = 1% TiO2, and 0.7% PVP with PVDF. | |
Based on all these results, the following photocatalytic mechanism for the generation of reactive species during photolysis and the degradation of CIP is presented below (eqn (3)–(7) and Fig. 9).
| TiO2 + hν(367 nm) → h+ + e− | (3) |
| (TiO2)CBe− + O2 → O2−˙ | (4) |
| (TiO2)VBh+ + H2O → HO˙ | (6) |
| CIP + (h+ + O2−˙ + HO˙ + 1O2) → Degradation Byproducts | (7) |
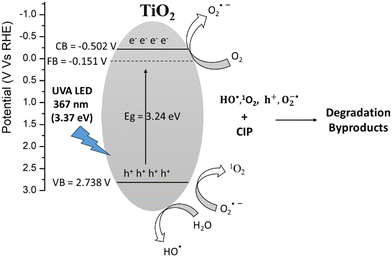 |
| Fig. 9 Photocatalytic mechanism for the degradation of CIP by TP beads under UVA LED irradiation. | |
3.2.7. Degradation in real water matrix.
The degradation of CIP in distilled water, simulated ground water (SGW) and real tap water was performed. The degradation efficiency of CIP in SGW and real tap water was determined to be about 74.6% and 94.6%, respectively (Fig. 10). This was attributed to presence of deactivating water constituents such as Cl−, HCO3−, and NOM.44 The degradation of CIP in the presence of individual water constituents like Cl−, HCO3−, and HA were determined (Fig. S11†). The quenching effect was observed in the order HA > Cl− ≥ HCO3−. Humic acid is known to retard the degradation reaction due to i) the blocking of the active site of the photocatalyst via adsorption, ii) a strong absorption in the UV-visible region that leads to the inner filter effect of the UVA irradiations and iii) consuming HO˙ and other reactive species generated in the reaction system.44,45 In accordance with the literature, Cl− and HCO3− were reported to react with HO˙ and photogenerated hole in TiO2 photocatalysis to generate secondary radicals such as Cl2−˙ and Cl3−˙ (eqn (8)–(13)) that are less reactive than HO˙, thereby decreasing the degradation efficiency.46–48 | HCO3− + HO˙ → CO3−˙ + H2O | (8) |
| HCO3− + h+ → CO3−˙ + H+ | (9) |
| ClHO−˙ + H+ → Cl˙ + H2O | (11) |
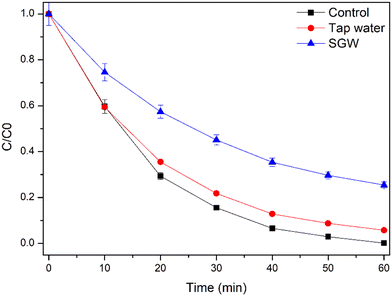 |
| Fig. 10 Degradation of CIP in real water matrix, experimental conditions: [CIP] = 1 ppm, TP = 1% TiO2, 0.7% PVP with PVDF beads, volume: 50 mL. | |
The deactivating water constituents in real tap water (HA = below permissible limit, HCO3− = 23.6 mg L−1, Cl− = 13.91 mg L−1) are relatively lower compared to SGW (HA = 10 mg L−1, HCO3− = 100 mg L−1, Cl− = 50 mg L−1). Hence, less negative impact on the degradation efficiency occurs in the case of real tap water compared to SGW (Fig. 10). Earlier similar results have been observed for the photocatalytic activity by slurry TiO2 nanoparticles.49
3.3. Recyclability and stability studies
One major drawback of TiO2 nanopowder is its recyclability as it forms a stable suspension that does not settle even after a long period of standing. The recovery of the TiO2 nanopowder requires energy-intensive processes such as centrifugation and filtration, which further involve loss of the catalyst. In the present work, the suspended TP beads were easily recovered post photocatalysis using a simple strainer. The recovered beads were investigated for their further use to degrade CIP. The recyclability studies of the TP beads were presented for 30 cycles (Fig. 11). Importantly, there was no reduction in the photocatalytic activity of the beads until 20 cycles. Above 20 cycles, a very small decrease in the photocatalytic activity was observed. At the end of 30 cycles, the efficiency was determined to be 90% (Fig. 11). The slight reduction in efficiency may be attributed to the trace amount of accumulation of organic pollutants over the active site of the photocatalyst.
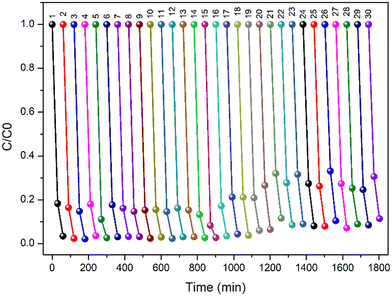 |
| Fig. 11 Recyclability test of TP bead, experimental conditions: [CIP] = 1 ppm, TP = 1% TiO2, 0.7% PVP with PVDF beads, and volume: 50 mL. | |
Further, the stability of immobilized TiO2 over PVDF beads was monitored by ICP-AES analysis. Importantly, no leaching of TiO2 was observed for the 30 cycles. The recyclability studies show that TP is highly reusable without the loss of TiO2 into water. Importantly, the FTIR spectra and SEM images were recorded for used TP beads (Fig. S12 and S13†), respectively. The IR result showed no difference in the functional group of the used TP bead and the SEM images showed the presence of TiO2 with no change in the surface morphology of the TP beads. All the above results strongly depict the effective use of TiO2 photocatalyst by the immobilization of TiO2 catalyst with PVDF beads via the simple phase inversion method for water treatment.
3.4. Identification of degradation byproducts of CIP
The LC-HRMS data of degradation byproducts along with the molecular formula, double bound equivalence (DBE) and the ppm error in the formula generated are presented in Table 1. Ten degradation byproducts were identified by LC-HRMS analysis (Scheme 1). The extracted ion chromatograms (EIC) for all the degradation byproducts are presented in Fig. S14–S24.† The plausible degradation pathways are presented in Scheme 1. Piperazine ring oxidation due to the attack by reactive species (O2−˙), h+, 1O2, HO˙) involving ring opening led to the degradation byproducts (2–8).50–52 Structures 9 and 10 are the decarboxylated products of 5 and 8, respectively.52–54 The structure 11 is the defluorinated product of structure 8 (Scheme 1).51
Table 1 HR-MS data for the degradation byproducts of CIP
Sr. no |
Compound |
(RT) (min) |
Experimental mass [(M + H)]+ |
DBE |
Mass error (ppm) |
1. |
Ciprofloxacin |
1.9 |
332.1408 |
10 |
0.82 |
C17H18FN3O3 (1) |
2. |
C17H16FN3O5 (2) |
2.0 |
362.1146 |
11 |
0.07 |
3. |
C17H18FN3O4 (3) |
2.0 |
348.0993 |
11 |
0.69 |
4. |
C16H16FN3O4 (4) |
2.1 |
334.1199 |
10 |
0.40 |
5. |
C16H16 FN3O3 (5) |
2.4 |
318.2791 |
9 |
−3.96 |
6. |
C15H16FN3O3 (6) |
1.8 |
306.1248 |
9 |
0.20 |
7. |
C14H11FN2O4 (7) |
2.1 |
291.0775 |
10 |
−0.08 |
8. |
C13H11FN2O3 (8) |
2.1 |
263.0828 |
9 |
0.84 |
9. |
C15H16FN3O (9) |
1.9 |
274.0983 |
9 |
−0.88 |
10. |
C12H11FN2O (10) |
2.3 |
219.1744 |
8 |
−4.36 |
11. |
C13H12N2O3 (11) |
2.1 |
245.0848 |
9 |
−4.94 |
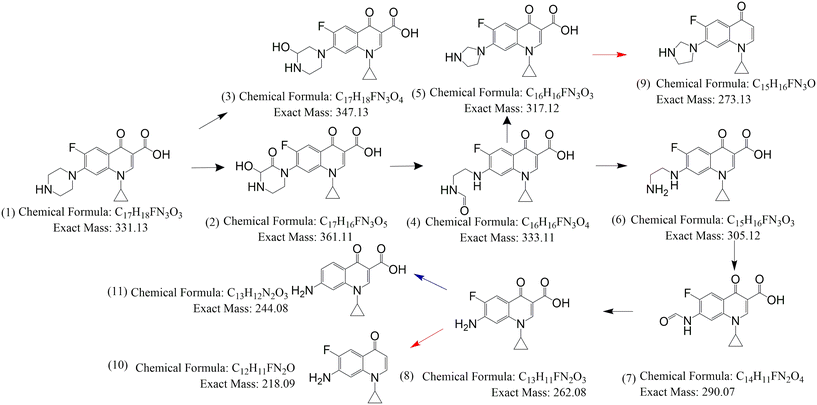 |
| Scheme 1 Possible degradation mechanism of CIP by TP beads; TP = 1% TiO2 with PVDF having 0.7% PVP. | |
3.5. Antibacterial activity removal
The antibacterial activity of CIP before treatment and after treatment (at 20, 40 and 60 min) by TP were determined (Fig. 12). These results reveal that CIP before treatment (0 min) showed the zone of inhibition of 1.8 ± 0.2 cm and 2.5 ± 0.1 cm against the target B. subtilis (Fig. 12a) and E. coli (Fig. 12b), respectively. A decrease in the zone of inhibition was observed as the treatment time was increased, both in case of B. subtilis and E. coli organisms. Importantly, there was no zone of inhibition against B. subtilis and E. coli after 60 min of treatment (Fig. 12). The complete removal of antibacterial activity is important to prevent the risk of antimicrobial resistance bacteria spreading into the environment due to antibiotics in water.
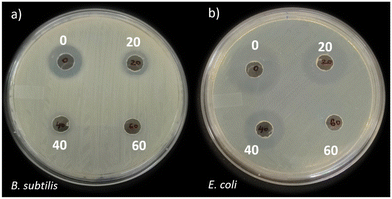 |
| Fig. 12 Antibacterial activity of 1 ppm CIP (0) and 20, 40 and 60 min TP bead-treated CIP against (a) B. subtilis and (b) E. coli; TP = 1% TiO2 with PVDF having 0.7% PVP. | |
3.6. Electrical efficiency
The electrical energy per order for the TP photocatalytic degradation of CIP under UVA LED irradiation was determined to be 24.20 kW h m−3, as discussed in Text S4.† Kokate et al., reported similar EEo values for the degradation of the dye pollutant by the 400 nm LED light source.55 The lower EEo value depicts the UVA LED as an energy-efficient light source for photocatalytic degradation.55 To the best of our knowledge, the present study is the first to report a stable immobilization of the TiO2 photocatalyst with PVDF bead support, which is extensively used as a polymeric membrane for water treatment in degrading the CIP, a widely detected antibiotic micropollutants with its antibacterial activity removed by an energy-efficient UVA LED light source.
4. Conclusion
The study presents the immobilization of TiO2 with PVDF beads (TP) by the phase inversion method and photocatalytic degradation of CIP by TP under UVA LED irradiation. The SEM images reveal the TP bead with a sponge-like morphology and immobilization of TiO2. The Ti-F coordination bond in TP revealed by XPS analysis was attributed to the effective immobilization of TiO2 in the spongy beads. UVDRS and Mott-Schottky analysis showed no significant modification in the electronic properties of TiO2 after immobilization with PVDF. The photocatalytic degradation of CIP obeyed pseudo first-order kinetics with kobs = 0.0761 min−1. Excess PVP, a hydrophilic additive, hinders the photocatalytic activity due to the masking of TiO2 nanoparticles. Highly acidic and basic pH conditions retarded the degradation efficiency. O2−˙, h+, HO˙, and 1O2 were identified as reactive species responsible for the degradation of CIP by TP according to the radical scavenging, photocurrent measurements and ESR. The efficient degradation of CIP was demonstrated in both tap water and surface groundwater. The easy recovery of TP beads from water was achieved by collecting the beads with a simple strainer. 30 cycles of photodegradation without the loss of TiO2 from TP beads were demonstrated. The degradation of CIP molecule due to the oxidation of the piperazine ring and defluorination reactions were proposed for the generation of the degradation byproducts. Additionally, antibacterial assays showed the removal of the antibacterial activity after the TP photocatalytic degradation of CIP. Energy efficiency calculations revealed that TP photocatalysis is an energy-efficient water treatment method under UVA LED irradiation with easy recovery. The study highlights polymeric beads with TiO2 obtained by the phase inversion technique as promising to enhance the usage of TiO2 nanoparticles for water treatment.
Data availability statement
All the data is contained within the article.
Author contributions
Laxman G. Raikar: methodology, validation, formal analysis, investigation, writing – original draft, writing – review & editing, visualization, Atul Patel: methodology, investigation Jemi Gandhi: methodology, investigation, KVK Gupta: resources, Halan Prakash: conceptualization, resources, writing – review & editing, supervision, funding acquisition.
Conflicts of interest
There are no conflicts to declare.
Acknowledgements
The authors gratefully acknowledge the support provided by the European Commission and the Department of Science and Technology (DST) of India through the “PANIWATER” project. This project falls within the framework of Indo-EU International Water Cooperation, funded by the European Union's Horizon 2020 research and innovation program under grant agreement number 820718. Furthermore, the authors express their gratitude for the central sophisticated instrumentation facility (CSIF) and laboratory facilities extended by BITS Pilani, K K Birla Goa Campus, Goa, India. The authors also extend their appreciation to Syngenta Bioscience Pvt. Ltd., Goa, India, for generously granting access to the HRMS facility. Furthermore, they acknowledge the Indian Institute of Technology, Bombay (IIT Bombay), India, for providing access to the EPR and ICP-AES facilities and University of Hyderabad for the TEM facility.
References
- Y. Tong, P. J. McNamara and B. K. Mayer, Adsorption of organic micropollutants onto biochar: a review of relevant kinetics, mechanisms and equilibrium, Environ. Sci.: Water Res. Technol., 2019, 5, 821–838 RSC.
- J. F. Kerrigan, K. D. Sandberg, D. R. Engstrom, T. M. LaPara and W. A. Arnold, Small and large-scale distribution of four classes of antibiotics in sediment: association with metals and antibiotic resistance genes, Environ. Sci.: Processes Impacts, 2018, 20, 1167–1179 RSC.
- M. D. J. S. Chaves, J. Kulzer, P. D. R. Pujol De Lima, S. C. Barbosa and E. G. Primel, Updated knowledge, partitioning and ecological risk of pharmaceuticals and personal care products in global aquatic environments, Environ. Sci.: Processes Impacts, 2022, 24, 1982–2008 RSC.
- R. Andreozzi, Advanced oxidation processes (AOP) for water purification and recovery, Catal. Today, 1999, 53, 51–59 CrossRef CAS.
- C. Byrne, G. Subramanian and S. C. Pillai, Recent advances in photocatalysis for environmental applications, J. Environ. Chem. Eng., 2018, 6, 3531–3555 CrossRef CAS.
- M. C. Dlamini, M. S. Maubane-Nkadimeng and J. A. Moma, The use of TiO2/clay heterostructures in the photocatalytic remediation of water containing organic pollutants: A review, J. Environ. Chem. Eng., 2021, 9, 106546 CrossRef CAS.
- A. Ajmal, I. Majeed, R. N. Malik, H. Idriss and M. A. Nadeem, Principles and mechanisms of photocatalytic dye degradation on TiO 2 based photocatalysts: a comparative overview, RSC Adv., 2014, 4, 37003–37026 RSC.
- H. Ding and J. Hu, Degradation of ibuprofen by UVA-LED/TiO2/persulfate process: Kinetics, mechanism, water matrix effects, intermediates and energy consumption, Chem. Eng. J., 2020, 397, 125462 CrossRef CAS.
- D. E. Willis, E. C. Sheets, M. R. Worbington, M. Kamat, S. K. Glass, M. J. Caso, T. Ofoegbuna, L. M. Diaz, C. Osei-Appau, S. D. Snow and K. M. McPeak, Efficient Chemical-Free Degradation of Waterborne Micropollutants with an Immobilized Dual-Porous TiO 2 Photocatalyst, ACS ES&T Eng., 2023, 3, 1694–1705 Search PubMed.
- D. P. Utomo, T. D. Kusworo, A. C. Kumoro and M. H. D. Othman, Developing a versatile and resilient PVDF/CeO2@GO-COOH photocatalytic membrane for efficient treatment of antibiotic-contaminated wastewater, J. Water Process Eng., 2023, 56, 104353 CrossRef.
- S. Xu, Y. Liu, Y. Yu, X. Zhang, J. Zhang and Y. Li, PAN/PVDF chelating membrane for simultaneous removal of heavy metal and organic pollutants from mimic industrial wastewater, Sep. Purif. Technol., 2020, 235, 116185 CrossRef CAS.
- H. S. Zakria, M. H. D. Othman, R. Kamaludin, S. H. S. A. Kadir, T. A. Kurniawan and A. Jilani, Immobilization techniques of a photocatalyst into and onto a polymer membrane for photocatalytic activity, RSC Adv., 2021, 11, 6985–7014 RSC.
- A. Ashley, B. Thrope, M. R. Choudhury and A. H. Pinto, Emerging investigator series: photocatalytic membrane reactors: fundamentals and advances in preparation and application in wastewater treatment, Environ. Sci.: Water Res. Technol., 2022, 8, 22–46 RSC.
- H. P. Ngang, B. S. Ooi, A. L. Ahmad and S. O. Lai, Preparation of PVDF–TiO2 mixed-matrix membrane and its evaluation on dye adsorption and UV-cleaning properties, Chem. Eng. J., 2012, 197, 359–367 CrossRef CAS.
- S. Wan, W. Zhao, D. Xiong, S. Li, Y. Ye and L. Du, Novel alginate immobilized TiO 2 reusable functional hydrogel beads with high photocatalytic removal of dye pollutions, J. Polym. Eng., 2022, 42, 978–985 CrossRef CAS.
- I. Dalponte Dallabona, Á. L. Mathias and R. M. M. Jorge, A new green floating photocatalyst with Brazilian bentonite into TiO2/alginate beads for dye removal, Colloids Surf., A, 2021, 627, 127159 CrossRef CAS.
- S. Chkirida, N. Zari, R. Achour, H. Hassoune, A. Lachehab, A. E. K. Qaiss and R. Bouhfid, Highly synergic adsorption/photocatalytic efficiency of Alginate/Bentonite impregnated TiO2 beads for wastewater treatment, J. Photochem. Photobiol., A, 2021, 412, 113215 CrossRef CAS.
- K. Wu, B. Li, X. Dong, P. Wu, K. Sun, S. Yang, J. Wu, J. Hou, Z. Liu and X. Guo, Flexible And Recyclable PPy-TiO 2 @WO 3 Photocatalyst Supported By Cellulose Aerogel Beads, ChemistrySelect, 2020, 5, 6527–6536 CrossRef CAS.
- D. Cani and P. P. Pescarmona, Macroscopic TiO2–SiO2 porous beads: Efficient photocatalysts with enhanced reusability for the degradation of pollutants, J. Catal., 2014, 311, 404–411 CrossRef CAS.
- S. K. Mehta, S. Kumar, S. Chaudhary and K. K. Bhasin, Nucleation and growth of surfactant-passivated CdS and HgS nanoparticles: Time-dependent absorption and luminescence profiles, Nanoscale, 2010, 2, 145–152 RSC.
- N. M. Mokhtar, W. J. Lau, A. F. Ismail and B. C. Ng, Physicochemical study of polyvinylidene fluoride–Cloisite15A® composite membranes for membrane distillation application, RSC Adv., 2014, 4, 63367–63379 RSC.
- L. G. Raikar, J. Gandhi, K. V. K. Gupta and H. Prakash, Degradation of Ampicillin with antibiotic activity removal using persulfate and submersible UVC LED: Kinetics, mechanism, electrical energy and cost analysis, Chemosphere, 2024, 349, 140831 CrossRef CAS PubMed.
- S. Gupta, J. Gandhi, S. Kokate, L. G. Raikar, V. G. Kopuri and H. Prakash, Augmented photocatalytic degradation of Acetaminophen using hydrothermally treated g-C3N4 and persulfate under LED irradiation, Heliyon, 2023, 9, e16450 CrossRef CAS PubMed.
-
F. R. Cockerill, Methods for dilution antimicrobial susceptibility tests for bacteria that grow aerobically: approved standard, CLSI, Wayne, Pa, 9th edn, 2012 Search PubMed.
- T. M. H. Le, R. Wang and S. Sairiam, Self-protecting PVDF-PDA-TiO2 membranes towards highly efficient and prolonged dye wastewater treatment by photocatalytic membranes, J. Membr. Sci., 2023, 683, 121789 CrossRef CAS.
- M. O. Mavukkandy, M. R. Bilad, A. Giwa, S. W. Hasan and H. A. Arafat, Leaching of PVP from PVDF/PVP blend membranes: impacts on membrane structure and fouling in membrane bioreactors, J. Mater. Sci., 2016, 51, 4328–4341 CrossRef CAS.
- Z. Guo, X. Xu, Y. Xiang, S. Lu and S. P. Jiang, New anhydrous proton exchange membranes for high-temperature fuel cells based on PVDF–PVP blended polymers, J. Mater. Chem. A, 2015, 3, 148–155 RSC.
- O. T. Alaoui, Q. T. Nguyen, P. Schaetzel and C. Mbareck, Dye and bacteria photodegradations with anatase-loaded microporous poly(vinylidene fluoride) membranes, Catal. Sci. Technol., 2011, 1, 1412 RSC.
- C.-L. Liang, Q. Xie, R.-Y. Bao, W. Yang, B.-H. Xie and M.-B. Yang, Induced formation of polar phases in poly(vinylidene fluoride) by cetyl trimethyl ammonium bromide, J. Mater. Sci., 2014, 49, 4171–4179 CrossRef CAS.
- Y. Zhang, G. Zhang, S. Liu, C. Zhang and X. Xu, “Naked” TiO2 capsulated in nanovoid microcapsule of poly(vinylidene fluoride) supporter with enhanced photocatalytic activity, Chem. Eng. J., 2012, 204–206, 217–224 CrossRef CAS.
- Z. Cui, N. T. Hassankiadeh, Y. Zhuang, E. Drioli and Y. M. Lee, Crystalline polymorphism in poly(vinylidenefluoride) membranes, Prog. Polym. Sci., 2015, 51, 94–126 CrossRef CAS.
- M. Wang, D. Fang, N. Wang, S. Jiang, J. Nie, Q. Yu and G. Ma, Preparation of PVDF/PVP core–shell nanofibers mats via homogeneous electrospinning, Polymer, 2014, 55, 2188–2196 CrossRef CAS.
- N. D. Kulkarni and P. Kumari, Development of highly flexible PVDF-TiO2 nanocomposites for piezoelectric nanogenerator applications, Mater. Res. Bull., 2023, 157, 112039 CrossRef CAS.
- L. Ye, C. Yang, L. Tian, L. Zan and T. Peng, Tunable photocatalytic selectivity of fluoropolymer PVDF modified TiO2, Appl. Surf. Sci., 2011, 257, 8072–8077 CrossRef CAS.
- Z. Tian, Y. Song, J. Zhang, S. Qin, Y. Yang, J. Li and Z. Cui, In situ growth of TiO2 and its immobilization on PVDF films for the adsorption and photocatalytic degradation of dye, Int. J. Hydrogen Energy, 2024, 51, 837–847 CrossRef CAS.
- S. N. S. Nasir, N. A. Mohamed, M. A. Tukimon, M. F. M. Noh, N. A. Arzaee and M. A. M. Teridi, Direct extrapolation techniques on the energy band diagram of BiVO4 thin films, Phys. B, 2021, 604, 412719 CrossRef CAS.
- N. Agrawal, R. S. Ray, M. Farooq, A. B. Pant and R. K. Hans, Photosensitizing Potential of Ciprofloxacin at Ambient Level of UV Radiation: Photochemistry and Photobiology, 2007, 83, Photochem. Photobiol., 2007, 83, 1226–1236 CrossRef CAS PubMed.
- X. Zheng, Y. Liu, X. Liu, Q. Li and Y. Zheng, A novel PVDF-TiO2@g-C3N4 composite electrospun fiber for efficient photocatalytic degradation of tetracycline under visible light irradiation, Ecotoxicol. Environ. Saf., 2021, 210, 111866 CrossRef CAS PubMed.
- A. Balakrishnan, M. Chinthala and R. K. Polagani, 3D kaolinite/g-C3N4-alginate beads as an affordable and sustainable photocatalyst for wastewater remediation, Carbohydr. Polym., 2024, 323, 121420 CrossRef CAS PubMed.
- J. Ma, Y. Tang, G. Lu, Y. Wang, W. Niu, D. Fu, K. Zhang, D. W. Bahnemann and J. H. Pan, Incorporating Mesoporous Anatase TiO 2 Spheres to Conductive Carbon Black Filled PVDF Membrane for Self-Cleaning Photo(electro)catalytic Filtration, J. Phys. Chem. C, 2023, 127, 7998–8005 CrossRef CAS.
- M. Manjunatha and H. Mahalingam, Upcycling of waste EPS beads to immobilized codoped TiO2 photocatalysts for ciprofloxacin degradation and E. coli disinfection under sunlight, Sci. Rep., 2023, 13, 14631 CrossRef CAS PubMed.
- E. Erusappan, S. Thiripuranthagan, R. Radhakrishnan, M. Durai, S. Kumaravel, T. Vembuli and N. J. Kaleekkal, Fabrication of mesoporous TiO2/PVDF photocatalytic membranes for efficient photocatalytic degradation of synthetic dyes, J. Environ. Chem. Eng., 2021, 9, 105776 CrossRef CAS.
- C. Chen, S. Wang, F. Han, X. Zhou and B. Li, Synergy of rapid adsorption and photo-Fenton-like degradation in CoFe-MOF/TiO2/PVDF composite membrane for efficient removal of antibiotics from water, Sep. Purif. Technol., 2024, 333, 125942 CrossRef CAS.
- A. R. Lado Ribeiro, N. F. F. Moreira, G. Li Puma and A. M. T. Silva, Impact of water matrix on the removal of micropollutants by advanced oxidation technologies, Chem. Eng. J., 2019, 363, 155–173 CrossRef CAS.
- Y.-J. Lee, Y. Jae Jeong, I. Sun Cho, C.-G. Lee, S.-J. Park and P. J. J. Alvarez, The inhibitory mechanism of humic acids on photocatalytic generation of reactive oxygen species by TiO2 depends on the crystalline phase, Chem. Eng. J., 2023, 476, 146785 CrossRef CAS.
- A. Bianco, M. I. Polo-López, P. Fernández-Ibáñez, M. Brigante and G. Mailhot, Disinfection of water inoculated with Enterococcus faecalis using solar/Fe(III)EDDS-H2O2 or S2O82− process, Water Res., 2017, 118, 249–260 CrossRef CAS PubMed.
- C.-R. Zhong, T.-W. Lee, J.-A. Li, Y.-H. Lai, T.-J. Ha and C. Chen, Origin of the enhanced photocatalytic activity of graphitic carbon nitride nanocomposites and the effects of water constituents, Carbon, 2020, 167, 852–862 CrossRef CAS.
- J. Gandhi and H. Prakash, Photo-disinfection processes for bacterial inactivation and underlying principles for water constituents' impact: A review, Chem. Eng. J. Adv., 2023, 14, 100482 CrossRef CAS.
- N. Jallouli, L. M. Pastrana-Martínez, A. R. Ribeiro, N. F. F. Moreira, J. L. Faria, O. Hentati, A. M. T. Silva and M. Ksibi, Heterogeneous photocatalytic degradation of ibuprofen in ultrapure water, municipal and pharmaceutical industry wastewaters using a TiO2/UV-LED system, Chem. Eng. J., 2018, 334, 976–984 CrossRef CAS.
- T. An, H. Yang, G. Li, W. Song, W. J. Cooper and X. Nie, Kinetics and mechanism of advanced oxidation processes (AOPs) in degradation of ciprofloxacin in water, Appl. Catal., B, 2010, 94, 288–294 CrossRef CAS.
- T. Ahamad, Mu. Naushad and S. M. Alshehri, Analysis of degradation pathways and intermediates products for ciprofloxacin using a highly porous photocatalyst, Chem. Eng. J., 2021, 417, 127969 CrossRef CAS.
- X. Zheng, S. Xu, Y. Wang, X. Sun, Y. Gao and B. Gao, Enhanced degradation of ciprofloxacin by graphitized mesoporous carbon (GMC)-TiO2 nanocomposite: Strong synergy of adsorption-photocatalysis and antibiotics degradation mechanism, J. Colloid Interface Sci., 2018, 527, 202–213 CrossRef CAS PubMed.
- D. Wu, J. Li, J. Guan, C. Liu, X. Zhao, Z. Zhu, C. Ma, P. Huo, C. Li and Y. Yan, Improved photoelectric performance via fabricated heterojunction g-C3N4/TiO2/HNTs loaded photocatalysts for photodegradation of ciprofloxacin, J. Ind. Eng. Chem., 2018, 64, 206–218 CrossRef CAS.
- J. Feng, L. Wang, X. Ran, B. Xiao, L. Lei, J. Zhu, R. Li, X. Xi and G. Feng, Adsorption and photocatalytic synergistic removal of ciprofloxacin on mesoporous ErFeO3/g-C3N4 heterojunction, Environ. Technol. Innovation, 2022, 28, 102785 CrossRef CAS.
- S. Kokate, S. Gupta, V. G. Kopuri and H. Prakash, Energy efficient photocatalytic activation of peroxymonosulfate by g-C3N4 under 400 nm LED irradiation for degradation of Acid Orange 7, Chemosphere, 2022, 287, 132099 CrossRef CAS PubMed.
|
This journal is © The Royal Society of Chemistry 2024 |
Click here to see how this site uses Cookies. View our privacy policy here.