DOI:
10.1039/D3NA00738C
(Review Article)
Nanoscale Adv., 2024,
6, 1974-1991
Nanotechnology-enabled sonodynamic therapy against malignant tumors
Received
5th September 2023
, Accepted 9th February 2024
First published on 26th March 2024
Abstract
Sonodynamic therapy (SDT) is an emerging approach for malignant tumor treatment, offering high precision, deep tissue penetration, and minimal side effects. The rapid advancements in nanotechnology, particularly in cancer treatment, have enhanced the efficacy and targeting specificity of SDT. Combining sonodynamic therapy with nanotechnology offers a promising direction for future cancer treatments. In this review, we first systematically discussed the anti-tumor mechanism of SDT and then summarized the common nanotechnology-related sonosensitizers and their recent applications. Subsequently, nanotechnology-related therapies derived using the SDT mechanism were elaborated. Finally, the role of nanomaterials in SDT combined therapy was also introduced.
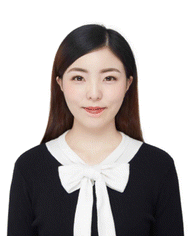 Yunxi Huang | Yunxi Huang, PhD, is currently a postdoc research scholar in Guangxi Medical University, and she completed her PhD from Guangxi Medical University in 2023. During her PhD study, she focused on the crosstalk between cancer metabolism and immune microenvironment, applications of nanomaterials in the diagnosis and treatment of cancer. Now, in her postdoctoral research phase, she will continue to conduct in-depth research in these field. |
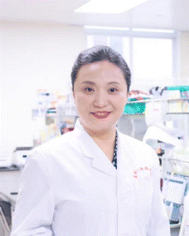 Junjie Liu | Junjie Liu, PhD, is a professor of Guangxi Medical University and the chief physician of the Guangxi Medical University Cancer Hospital at Department of Ultrasound. Dr Liu completed her PhD from Guangxi Medical University, Nanning, in 2012 and postdoc from Guangxi Medical University in 2015. Her present research interests include the development of advanced nano-materials for cancer therapy and drug/gene delivery in cancer, contrast agent material synthesis and application. |
1 Introduction
Tumor treatment is still a problematic issue in the field of medical research. Traditional treatment methods including chemotherapy, surgery, and radiation therapy that still suffer from severe side effects and narrow appropriate windows remain unsatisfactory since no substantial progress has been made in malignant tumor treatment. Therefore, it is urgent to find a tumor-specific treatment method featuring high efficiency and safety. In an attempt to chase for a new methodology, some new therapies, such as immune therapy, biological therapy, chemodynamic therapy, photodynamic therapy (PDT) and sonodynamic therapy (SDT), have attracted increasing attention due to their non-invasive and highly targeted characteristics, among which some have been applied in clinics. Typically, PDT has been approved for actinic keratosis, basal cell carcinoma and Bowen's disease in clinics.1
It has been extensively accepted that SDT using ultrasound as a trigger has evolved from PDT, but shares deeper penetration than PDT.2 Generally, low-intensity ultrasound (US) (0.5–5.0 w/cm−2) was used to stimulate sonosensitizers to generate reactive oxygen species (ROS)3 (Fig. 1A), thus exerting a killing effect on tumor cells and achieving tumor inhibition or even repression. Specifically, intervening intratumoral ROS metabolism, breaking the redox equilibrium and reshaping the tumor microenvironment can significantly reinforce SDT against tumors.4 Compared with PDT, SDT is endowed with the following advantages.5,6 Firstly, US as a mechanical wave has a deep tissue penetration, which overcomes the penetration depth limit of PDT and provides the possibility for SDT of deep-seated malignant tumors. Second, US can kill tumor cells through a variety of mechanisms. Thirdly, US can be focused and selectively confined at the site of the lesion, which determines that SDT has strong targeting and safety in tumor treatment, especially for deep tumors. Fourthly, the phototoxicity of photosensitizers can be greatly reduced by ultrasonic activation. Fifth, the equipment is simple and easy to operate and has a wide range of applications. Moreover, it can be targeted at different depths and different sites of tumors. Fig. 1B shows the comparison between SDT and PDT. In light of these advantages, SDT has been highly valued by scholars all over the world as soon as it was proposed.7,8
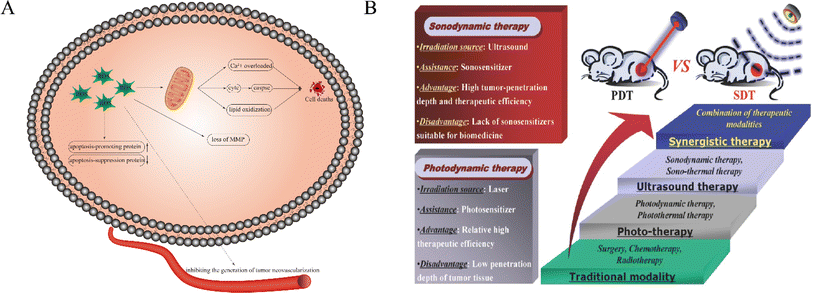 |
| Fig. 1 (A) Schematic illustration of the possible anticancer mechanism of ROS in SDT. ROS play a key role in the process of cell apoptosis. ROS can lead to apoptosis through calcium overload, cytC release and lipid oxidation. In addition, they can also cause cell death by regulating apoptosis-related gene expression, tumor angiogenesis and loss of MMP. (B) The comparison between SDT and PDT.8 Reproduced from ref. 8 with permission from John Wiley & Sons, copyright 2016. | |
Up to now, the underlying mechanism of SDT has not been completely understood. It is generally believed that there are two main types of pathways, as categorized in Fig. 2, wherein the first one is the ultrasonic cavitation effect.9 The ultrasonic cavitation effect means that ultrasonic waves cause an acoustic pressure change in the liquid medium, resulting in bubble generation in the interstitial fluid. After the tiny bubbles expand and shrink cyclically until they collapse, energy is released instantaneously, resulting in drastic elevations of temperature up to 10
000 K and pressure up to 81 MPa in the surrounding microenvironment.10 This part of energy imposes on the cell mechanically, causing membrane permeability variation, thus damaging the cell.11 The cavitation effect can be divided into no-inertial cavitation and inertial cavitation12 (Fig. 3) according to the pressure intensity of applying ultrasonic waves. When low pressure intensity is applied, microbubbles (MBs) expand and shrink in the ultrasonic field responding to the negative pressure and positive pressure phases of US. This process is called no-inertial cavitation. In contrast, when a relatively high pressure intensity is applied, the MBs grow rapidly to exceed a critical size and eventually collapse, during which shock waves are accompanied to further enhance the sonoporation. This phenomenon is well known as inertial cavitation. Inertial cavitation is closely related to ROS production,10 and the extreme physical conditions produced by collapse will have destructive impacts on the cytoskeleton, membrane structure and enzyme activity, and even kill the surrounding cells. In addition, the enhanced sonoporation caused by inertial cavitation can make the cell membrane and blood vessels permeable and promote the effective entry of drugs into diseased tissues.
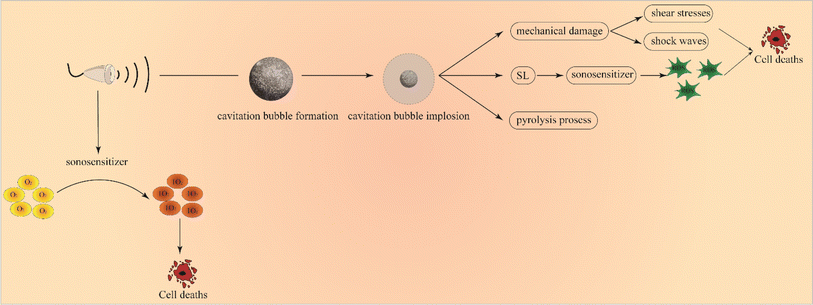 |
| Fig. 2 Schematic illustration of the possible mechanism of SDT. US can induce cavitation implosion and further lead to cell death through mechanical damage, SL and the pyrolysis process. Furthermore, US can directly activate the sonosensitizer to produce singlet oxygen leading to cell death. | |
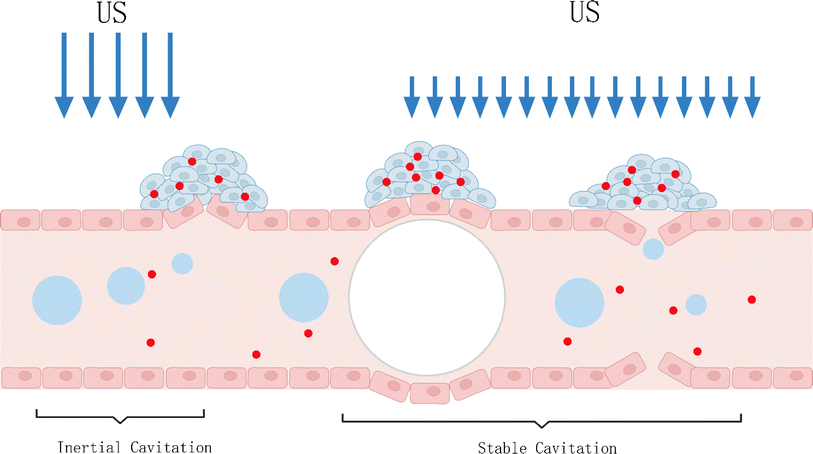 |
| Fig. 3 Microbubble interactions with ultrasound. Inertial cavitation events at a high mechanical index, causing disruption of the endothelial lining. At lower US pressures, volumetric oscillations of the microbubble can stretch or distend the blood vessel. | |
The other is the killing effect of sonosensitizers activated using US. Some documents reported that sonoluminescence (SL) arising from photo-excited sonosensitizers when MBs burst brought about ROS, leading to cell death.13 As a paradigm, Sazgarnia et al.14 successfully detected SL in gel-based phantom by using protoporphyrin IX coupled to gold NPs, indicating that SL played an effective role in SDT. However, Hachimine et al.15 developed a novel porphyrin derivative that was denoted as DCPH-P-Na(I) and found that the sensitizer could not only absorb light, but also exert high cytotoxic effects on cancer cells under US irradiation, suggesting no SL's contribution to improving SDT efficacy. Therefore, the SL contribution has not been fully clarified yet and further research is needed to definitely unravel its role as a sonosensitizer activator. As well, US has also been believed to directly excite sonosensitizers. In this case, sonosensitizers can absorb US mechanical energy and generate electron jump from a low energy state to a high energy state. When it returns to the low energy state, a large amount of energy is released and transferred to oxygen species to give birth to highly reactive singlet oxygen for killing tumor cells. Taking all the above together, two underlying principles are co-existing in SDT.
It has been reported that nanotechnology can significantly improve the ability and photostability of fluorescence imaging.16 A coordination-dependent longitudinal relaxation tuning (CLRT) that occurs between a Mn “donor” and a Mn “acceptor” was confirmed to enable biological target sensing and has great potential to detect the occurrence, invasion and metastasis of malignant tumors.17 Moreover, recently, the rapid development of nanotechnology has brought new development directions to the treatment of cancer and nanoparticles (NPs) are getting a lot of attention, which has greatly changed the concept of traditional disease treatment.18–20 They are defined as submicron-sized (<1 mm) colloidal particles. It is well known that the size of NPs has a great influence on their therapeutic activity, which can significantly influence the mechanism and rate of cell internalization. Therefore, smaller NPs are more easily absorbed by tumor cells than larger ones, increasing the concentration of the drug at the site of action. Moreover, NPs can effectively invade tissues during local accumulation and release the drugs carried, thus improving treatment efficiency and minimizing side effects.21 There is no doubt that NPs will greatly promote the development of SDT and open a new way for the future design of sonosensitizers. Additionally, since inertial cavitation is a key mechanism for the delivery of microvesically targeted drugs, NPs can be stabilized and used as cavitation nuclei to initiate acoustic inertial cavitation of nanobubbles on their surfaces and in specific cavities. It has been found that a rough, hydrophobic NP surface preserves surface nanobubbles better and induces effective inertial cavitation.22 Therefore, due to high precision, deep tissue penetration, and minimal side effects, SDT has a great application prospect in anti-tumor therapy.
Unlike previous reviews, in this review, we systematically and comprehensively summarize the role of nanotechnology in SDT, not just the role of nanosonosensitizers. In short, the anti-tumor mechanism of SDT is briefly described. In addition, the application of nanoparticles in sonosensitizers, the application of nanoparticles in SDT combined with other therapies, and the application of nanobubbles in drug delivery are also introduced. It is of vital significance to understand the development of SDT, which may help it enter clinical practice as soon as possible, further significantly improving the prognosis of tumor patients.
2 Anti-tumor mechanism of SDT
As a new tumor treatment method, SDT can provide the possibility of noninvasive and targeted eradication of solid tumors through the comprehensive effects of many aspects. In addition to inducing cell apoptosis, lipid peroxidation, and mitochondrial membrane potential loss, SDT also damages DNA and activates different ROS signaling pathways. Many studies have confirmed that the production of a large amount of intracellular ROS induced by SDT can produce direct cytotoxicity in tumor cells and lead to tumor cell death or induce tumor cell apoptosis to produce anti-tumor effects.23–25 SDT can also make the body produce a lot of antigens and stimulate the anti-tumor immunity of the body. In addition, tumor blood vessels, as an important component of the tumor microenvironment, play an important role in the process of tumor recurrence and metastasis. SDT can effectively cut off tumor blood supply by inhibiting the generation of tumor neovascularization or can also destroy tumor vascular epithelial cells and make them release thromboxins, forming thrombosis in tumor vessels and causing ischemic necrosis of tumor tissues, thus inhibiting tumor growth.
2.1 The cytotoxic effects of SDT
The mechanism of cytotoxic effects of SDT is a complex topic, and the cytotoxicity of SDT cannot be easily understood. There may be two mechanisms by which SDT induces cytotoxicity. The cavitation effect caused by US irradiation causes MBs to break, which causes mechanical cell death through the generated shear force or shock wave, or indirectly causes cell death through the generation of ROS. Moreover, US can also activate NPs to generate ROS and kill cells through the cavitation effect. Oxidative stress plays a key role in SDT-induced cytotoxicity, and the types of cell death induced under different conditions are also different,26,27 which may cause apoptosis or necrosis, and the US intensity can determine the proportion of apoptosis and necrosis. Increased ROS and loss of mitochondrial membrane potential (MMP) have been reported to be associated with apoptosis and necrosis of macrophages induced by SDT.28 Intracellular ROS can also damage the mitochondrial membrane by promoting lipid peroxidation, leading to depolarization of MMP and an increase in mitochondrial membrane permeability, leading to cell apoptosis.29 Furthermore, ROS leads to damage of the mitochondrial membrane, which further leads to the release of cytochrome C (Cyt C) from mitochondria into cytoplasm and subsequently activates the caspase-dependent apoptosis pathway to indirectly kill tumor cells.30,31 Research results by Li et al.32 showed that US irradiation alone or chloride E6 (Ce6) alone showed no significant anti-tumor effect on non-small cell lung carcinoma (NSCLC), but Ce6 combined with US irradiation had a significant inhibitory effect on tumor growth. Flow cytometry analysis showed that Ce6 mediated the sonodynamic effect mainly through ROS – induced cell necrosis. A study on the possible mechanism of enhanced US of hematoporphyrin monomethyl ether (HMME) on the cytotoxicity of osteosarcoma has found that its cytotoxicity may be related to the increase of intracellular ROS and Ca(2+),33 whereas Bismuth et al.,34 in their study of the killing mechanism of safe low energy tumor cells by SDT, found that immediate cell lysis is closely linked to inertial cavitation, which is known to produce shear forces that significantly disrupt cellular membranes.
Metal NPs due to their inherent biological inertia and low toxicity are widely used as nanocarriers for delivering drugs, or as sonosensitizers to increase the ability of SDT to kill tumor cells. Moreover, they can be functionalized using surface-available active targeting units (proteins, peptides, monoclonal antibodies, small molecules, etc.) to avoid non-specific uptake, thus achieving tumor-specific targeted therapy. The cytotoxicity mechanism of metal NPs induced by US is highly dependent on the properties and characteristics of both US and NPs and is significantly influenced by environmental conditions. For instance, even if they are in the same category, sonosensitizers such as metal oxide NPs may exhibit distinct behaviors under the same experimental conditions. Additionally, varying ultrasonic parameters, such as frequency and intensity, may elicit different reactions to the same sonosensitizer. The three precise mechanisms of metal NPs in the application of SDT are:35: (I) the generation of ROS; (II) the mechanical destruction of cell membranes; (III) the metal-ion release. The production of ROS may be based on their chemical structure, which could be efficiently induced by the US stimulus in the context of SDT, or due to the interaction between NPs and the electron transport chain placed in the cell mitochondrial apparatus. The precise mechanism of cell membrane destruction remains to be further elucidated. The dissociation of NPs due to US can amplify physical interactions with cell membranes, leading to increased cytotoxic effects. Moreover, certain metal oxide NPs possess inherent cytotoxicity.36,37 US stimulation is known to induce the release of metal ions, which is often regarded as the primary or exclusive cause of toxicity associated with metal oxide NPs. One instance is the dissolution of ZnO NPs into Zn2+ ions, which can trigger mitochondria-driven apoptosis and protein imbalance toxicity.38
2.2 The inhibitory tumor angiogenesis effect of SDT
With the exception of leukemia and other hematological malignancies, primary tumors inherently rely on nutrients and oxygen supply from available vascular networks, so tumor angiogenesis is essential for the growth and metastasis of invasive tumors, which is the key point in controlling cancer progression. Studies have shown that SDT-induced ROS production can significantly inhibit the proliferation, migration and invasion of endothelial cells, as well as angiogenesis.39 In addition, in a tumor-bearing mouse model, SDT can significantly inhibit tumor growth, intracellular angiogenesis and expression of the vascular endothelial growth factor (VEGF).40 Xiong et al.41 found that sonosensitizer HMME could enhance the US-induced antitumor effect by selectively assisting US targeting of glioma angiogenesis inhibition. Yao et al.42 showed that 5-aminolevulinic acid (5-ALA)-based SDT could enhance the cytotoxicity of bleomycin (BLM) toward mouse mammary tumor cells. Moreover, 5-ALA-mediated SDT combined with sinoporphyrin sodium (DVDMS) inhibited tumor growth not only by direct killing of cancer cells, but also by the effect on tumor angiogenesis. These methods of inhibiting the nutritional supply of tumors are also called starvation therapies and they can be reinforced using NPs.43
2.3 The induced apoptosis effect of SDT
Apoptosis is a process of programmed cell death that eliminates aged, damaged, or superfluous cells via a range of intracellular and extracellular signals. Apoptotic cells exhibit various characteristics such as cytoskeletal contraction, chromatin condensation, DNA fragmentation, and caspase activation. Although the mechanism of apoptosis induced by SDT is not fully understood, some studies suggest that SDT can promote cell apoptosis, which in turn affects in vivo angiogenesis. When microvesicles are exposed to US, they can damage blood vessels and vascular endothelium at the tumor site, leading to the formation of intravascular thrombosis and blocking the blood supply to the tumor. The resulting lack of nutrients and oxygen in tumor blood vessels creates an anoxic environment that induces oxidative stress and apoptosis in cancer cells.44 Moreover, SDT can also induce apoptosis by regulating the expression of apoptosis-related genes, caspase-3 activation or Ca2+ overload in the mitochondrial membrane.45 Meng et al.46 conducted an in vitro study on the apoptotic effect and mechanism of HMME-mediated SDT on oral squamous cell carcinoma (OSCC). The results showed that SDT treatment induced apoptosis in OSCC cells, which was associated with the generation of ROS and MMP loss. Furthermore, SDT treatment significantly increased the protein expressions of Bax, Caspase-9, and Caspase-3, while decreasing the expressions of Bcl-2 and FAS-L, with the expression of the Fas protein remaining unchanged. Similarly, another study investigated the effect of HMME-SDT on endometrial cancer and found that it could promote apoptosis.47 The author discovered that HMME-SDT was more potent in promoting ROS production, inducing MMP loss, and increasing the intracellular Ca2+ concentration. Furthermore, HMME-SDT upregulated the expression of pro-apoptotic proteins, such as Bax, Fas, and FAS-L, while downregulating the expression of anti-apoptotic proteins, including Bcl-2 and Survivin. Additionally, the HMME-SDT groups exhibited significantly elevated levels of cleaved caspase-3 and caspase-8. Hao et al.48 explored the impact of intracellular Ca2+ overload on HMME-mediated SDT-induced apoptosis of C6 glioma cells. The findings demonstrated that in the presence of a Ca2+-supplemented medium, SDT treatment led to increased intracellular ROS production, decreased MMP, and the release of Cytc from mitochondria. As a result, the combination of low-intensity US and HMME was found to enhance the apoptosis rate of C6 glioma cells when compared to US treatment alone, with Ca2+ overload playing a crucial role in the activation of mitochondrial signaling pathways in SDT-induced cell apoptosis. In addition, Wang et al.49 found that SDT also caused some inflammation while destroying the tumor, indicating a “vaccine” effect. Thus, apoptotic cell fragments may become tumor antigens, triggering an immune response that resists tumor recurrence and metastasis. Moreover, combining nanosonosensitizer-augmented SDT with anti-PD-L1 can induce an anti-tumour response.50 Additionally, the highly accumulative ROS arising from continuous US-triggered inertial cavitation have been demonstrated to induce robust immunogenic cell death (ICD).51
Apoptosis and autophagy can be seen in SDT treated tumor cells at the same time. Autophagy is considered a double-edged sword, which may coordinate, exacerbate or antagonize apoptosis, and ROS plays an important role in apoptosis and autophagy. Autophagy may have a cell-protective effect,52 but another study found that blocking autophagy was expected to eliminate cell resistance to SDT.53 Therefore, the role of autophagy in the anti-tumor process of SDT has not been fully elucidated, and whether its specific role is positive or negative needs further study.
3 Nano-sonosensitizers
Photodynamic therapy (PDT) refers to a new method of treating tumor diseases with photosensitive drugs and laser activation. It requires a photosensitizer, light energy and oxygen to generate ROS. SDT is developed on the basis of PDT,54 and its principle is similar. The SDT effect was first recognized in 1989 by Yumita and Umemura,55 who observed the cytotoxic effects of hematoporphyrin (HP) in acoustic fields and subsequently referred to such photosensitizers as senosensitizers.56 The sonosensitizer itself has no inhibitory activity and low toxicity and biological activity only after exposure to US. As an important component of SDT, sonosensitizers can maximize the therapeutic effect of US irradiation on tumors. However, most sonosensitizers are hydrophobic and tend to accumulate in the physiological environment. The presence of hydrophobic interaction results in strong intermolecular forces between sonosensitizers, reducing their water solubility and decreasing the production of ROS. Additionally, many sonosensitizers exhibit poor pharmacokinetic properties and are easily eliminated from the vascular system, limiting their exposure to pathological sites. Furthermore, their non-specific biological distribution and weak selectivity towards diseased tissue result in only a small fraction of their dosage reaching target sites. This inadequate intracellular concentration of sonosensitizers may not produce a therapeutic effect. To enhance the effectiveness of sonosensitizers in SDT, researchers have combined various nano-carriers with organic sonosensitizers and developed new inorganic sonosensitizers to improve biocompatibility and enhance sonodynamic efficiency.57 The classification of common nano-sonosensitizers is presented in Table 1.
Table 1 Category of common nanosonosensitizer materials in recent years
Nanoparticle-assisted sonosesitizers |
Porphyrins and their derivatives |
DVDMS-TiO2 |
Tumors |
64
|
Lipo-TPP-HMME |
MCF-7 cells |
67
|
Gold nanoparticle– PpIX |
Tumors |
69
|
MnO @Tf-ppIX |
Orthotopic glioblastoma |
70
|
MSN-DOx-CE6 |
MDA-MB-231 cells |
72
|
CBP NPs |
Breast cancer cell |
73
|
Xanthones |
BT-RB |
Hela cells |
74
|
RBD4 |
HepG2 cells |
75
|
RB-MB |
HT-29 tumor |
76
|
Non-steroid anti-inflammatory drugs |
PEGylated LFLXs |
Sarcoma 180 cells |
79
|
Other sonosensitizers |
Curcumin |
CNE2 cells |
80 and 81 |
HypocrellinB |
CNE2 cells and HepG2 cells |
82 and 83 |
Chlorophyll |
PC-3 cells and DU-145 cells |
84
|
Nanoparticles as intrinsic sonosensitizers |
TiO2 |
HSIPT-NPs |
SCC7 cells |
85
|
MnOx/TiO2-GR |
4T1 cells |
89
|
HTiO2 |
SCC7 cells |
90
|
HAu–TiO |
SCC7 cells |
91
|
SiNPs |
CFO |
Tumors |
93 and 94 |
DSiNPs |
HepG2 cells |
95
|
Fe3O4 |
Fe3O4 NPs |
MCF-7 cells |
96 and 97 |
Fe-HMME |
CT26 cells |
98 and 99 |
3.1 Nanoparticle-assisted sonosensitizers for SDT
3.1.1 Porphyrins and their derivatives.
Porphyrin was modified to reduce its optical toxicity, and a series of porphyrin derivatives were produced. The primary sonosensitizers of porphyrin and its derivatives are the most commonly used in practical research. Subsequently, porphyrin was modified to reduce its optical toxicity, and a series of porphyrin derivatives were produced, such as hematoporphyrin (Hp), photofrin, hematoporphyrin monomethylether (HMME), photoporphyrin IX (PpIX), ATX-70, DCPH-P-Na(I) and some other porphyrin derivatives.58–62 However, porphyrin and its derivatives are difficult to use in aqueous media due to their hydrophobic properties and their tendency to aggregate by stacking planar molecules. Thus, wrapping them in NPs or attaching them to various transport carriers has been used to improve transport characteristics. DVDMS is an active ingredient extracted from photosensitizers approved by the Food and Drug Administration (FDA). As mentioned above, DVDMS inhibits tumor proliferation by inducing tumor cell apoptosis and inhibiting tumor angiogenesis.63
Furthermore, TiO2 has been found to enhance the anti-tumor effectiveness of DVDMS.64 The combination of HMME and US irradiation shows promise as a potential cancer treatment. Studies have demonstrated that HMME-SDT can effectively induce apoptosis in endometrial cancer65 and enhance the killing effect of US on hepatic carcinoma.66 Chen et al.67 utilized mitochondrial targeting liposomes to load a hydrophobic HMME sonosensitizer. They discovered that upon US irradiation, HMME could be released from the liposome due to lipid oxidation. This process effectively prevented sonosensitizer aggregation and enhanced the anti-tumor effectiveness of HMME.
PpIX is a new type of hematoporphyrin derivative (HPD), and due to its easy uptake by cancer cells, PpIX showed stronger potential cytotoxicity upon US irradiation than hematoporphyrin (HP) under the same experimental conditions.68 Sazgarnia et al.69 successfully achieved targeted tumor delivery of PpIX by preparing gold NPs that complexed PpIX, significantly reducing its toxicity to normal tissues, and significantly enhanced the synergistic therapeutic effect on tumors by combining gold NP mediated thermotherapy with PpIX mediated SDT. Liang et al.70 constructed an intelligent nanoplatform based on holo-transferrin (Holo-TF) with in situ growth of MnO nanocrystals. Furthermore, PpIX, acting as a sonosensitizer, is then conjugated into Holo-TF to obtain MnO@Tf-ppIX nanoparticles (TMP). TMP can effectively traverse the blood–brain barrier (BBB) and be used for highly specific magnetic resonance imaging (MRI) of orthotopic glioblastoma, showing an obvious anti-tumor effect in SDT treatment.
Ce6 is a new kind of sonosensitizer, which can induce mitochondrial and caspase-dependent apoptosis through its mediated SDT and produce cytotoxicity in breast cancer.71 Mesoporous silica NPs synthesized using Ce6 (MSN-DOx-CE6) significantly enhanced the anti-tumor effects of doxorubicin (DOX) and SDT upon US irradiation.72 Chen et al.73 successfully designed and constructed biocompatible photothermal/photodynamic therapeutic nanoparticles (CBP NPs) based on Ce6 and polydopamine (PDA), which are more effective at killing tumors and have fewer side effects.
3.1.2 Xanthones.
Xanthones are heterocyclic compounds. It is an important organic synthesis intermediate and its parent does not exist in plants, but its derivatives are widely found in nature. Xanthones have a range of dyes including erythrina B, rose red (RB), eosin and rhodamine. RB is a tetrachlorotetriodized derivative of fluorescein and has no cytotoxicity when used alone. RB combined with US can selectively inhibit tumor growth without damaging normal tissue. The high water solubility and poor bioavailability of RB can be significantly improved by chemical modification of the RB surface. Sun et al.74 created barium titanate (BT) nanoparticles from RB, resulting in BT-RB NPs that were subsequently coated with PAH (polyacrylamide hydrochloride). The findings revealed that BT-RB NPs produced more ROS than RB alone, BT alone, or a combination of RB + BT NPs. Importantly, BT-RB NPs did not induce any cellular toxicity. Chen et al. designed, synthesized and characterized a series of amphiphilic RB derivatives and they found that RBD4 could most effectively improve cell uptake of light and US among them, enhancing the production efficiency of intracellular ROS, and thus significantly improve anticancer activity. Furthermore, it is worth noting that the synthesized derivatives had similar relative potency to DVDMS.75 Hou et al. designed a rose-colored Bengal microbubble (RB-MB), which combined RB-MBS with US to build a new delivery system of sonosensitizers with minimal side effects and significantly improve the efficacy of SDT.76 Moreover, MBs with an average particle size of around 1.7 µm and conjugated with sonosensitizer Rose Bengal (RB) can significantly enhance the effect of SDT.77
3.1.3 Non-steroid anti-inflammatory drugs.
Some non-steroidal anti-inflammatory drugs (NSaids) have significant anticancer effects upon US irradiation. It is suggested that quinolone antibiotics could have an anti-tumor effect on 180 cells of sarcoma. Moreover, the non-steroidal anti-inflammatory drugs tenoxicam and piroxicam can also promote the generation of intracellular ROS in sarcoma 180 cells after US irradiation and further showed significantly enhanced cytotoxicity.78 In addition, piroxicam enhanced the anti-tumor effect of US without damaging the normal tissue around the tumor.79 Komori et al. synthesized PEGylated LFLXs coupled with methoxypolyethylene glycol and demonstrated that the derivative had superior sonodynamics in anti-tumor activity than LFLX.
3.1.4 Other sonosensitizers.
Certain active compounds found in traditional Chinese medicine, including curcumin, hypocrellin B, and chlorophyll, exhibit promising sonosensitive properties with minimal toxicity, rendering them suitable candidates for disease treatment. Curcumin, a natural pigment extracted from Chinese herbs, has been identified as an effective sonosensitizer that can hinder macrophage growth by disrupting the mitochondrial membrane structure, promoting SLC1A5-mediated ferroptosis, lysing cytoskeleton, and interfering with the cell function.80 US sonication in the presence of curcumin significantly killed CNE2 cells and induced ultrastructural damage and the dysfunction of mitochondria.81 As a plant pigment, anthocyanin B combined with US irradiation can significantly induce apoptosis of CNE2 cells82 and HepG2 cells.83 Chlorophyll has very hydrophobic and low selective cancer tissue. To obtain an aqueous solution formulation of this natural pigment, BoscaF et al. transformed unmodified plant-extracted chlorophyll into nanoparticles of varying compositions and structures, such as liposomes, solid lipid nanoparticles, and poly (lactic-glycolic acid) nanoparticles. These nanoparticles were then incubated with human prostatic cancer cells (PC-3) and spheroids (DU-145). Results indicated that the poly (lactic-glycolic acid) nanoparticle loaded with chlorophyll produced the highest sonodynamic cytotoxicity, making it a promising candidate for future clinical investigations on SDT.84
3.2 Nanoparticles as intrinsic sonosensitizers for SDT
Recently, researchers have found that some NPs have photoacoustic activity, so they are also known as nanosensitizers. These sonosensitizers are nano-sized, which enables their dispersal in water without the need for additional solubilization, thereby evading the complement system and blood clearance. Moreover, their nanoscale size facilitates efficient cellular uptake and penetration into deep tissues. TiO2, a novel functional nanomaterial, is characterized by good biocompatibility, low cost, and ease of preparation with biological tissues, making it a popular research topic. Kim et al.85 were the first to demonstrate that ultrasonic stimulation of TiO2 nanoparticles can induce tumor cell death in both in vitro and in vivo settings. This process is thought to occur via US activation of TiO2 to generate ROS, thereby inhibiting the progression of deeper tumors. TiO2 can be synthesized in three forms: solid nanoparticles, mesoporous nanoparticles, and core/shell structured nanoparticles with functional cores.8 TiO2 can be activated using US to generate ROS. Additionally, the mesoporous structure of TiO2 NPs can encapsulate and deliver antitumor drugs, thereby achieving a concurrent and synergistic effect of chemotherapy and SDT. Furthermore, the functional core of core/shell-structured TiO2 NPs can provide diagnostic imaging capabilities, allowing for imaging-guided SDT, such as using a magnetic Fe3O4 core for MRI imaging.8
However, the biological distribution of traditional TiO2 NPs is not ideal. Modification of TiO2 NPs through selective selection of polyethylene glycol (PEG), glucan, polyionic complexes and other polymer materials can reduce the acute and long-term toxicity of TiO2 nanosonosensitizers.86–88 Moreover, it can also improve the water dispersibility of NPs, reduce their immunogenicity, and further enhance their biocompatibility so as to improve the anti-tumor effect of SDT. Wang et al.89 successfully prepared nano-TiO2 with an ultra-fine rod structure and modified it with PEG (Fig. 4A–C). Compared with the traditional nanosonosensitizers TiO2 NPs, PEG-TiO2 NRS, due to the anoxic structure of TiO2 NR, has a higher efficiency in sonoinduction of ROS, thus enhancing the therapeutic effect of SDT. In addition, PEG-TiO2 NRS also showed the activity of horseradish peroxidase nanase and could generate a hydroxyl radical (OH) from endogenous H2O2 in the tumor, so as to realize chemical kinetic therapy (CDT) and increase the therapeutic effect of SDT. Dai et al.90 report discussed the enhancement of semiconductor TiO-based nanosonosensitizer sonocatalytic efficiency for highly effective SDT by integrating two-dimensional (2D) ultrathin graphene with TiO2 nanosonosensitizers, leading to higher ROS production in vitro. Additionally, surface modification of TiO2 nanoparticles with carboxymethyl dextran (CMD) to produce hydrophilized TiO2 (HTiO2) nanoparticles was demonstrated to be effective in generating ROS under US irradiation (as shown in Fig. 4D). Moreover, HTiO2 NPs effectively suppressed the growth of superficial tumors and induced damage to tumor vasculature after US treatment (as shown in Fig. 4E–G).91 In addition, by introducing some precious metals to combine with TiO2 NPs, such as gold, silver and platinum, higher ROS quantum yield can be obtained and completely inhibit tumor growth in vivo.92
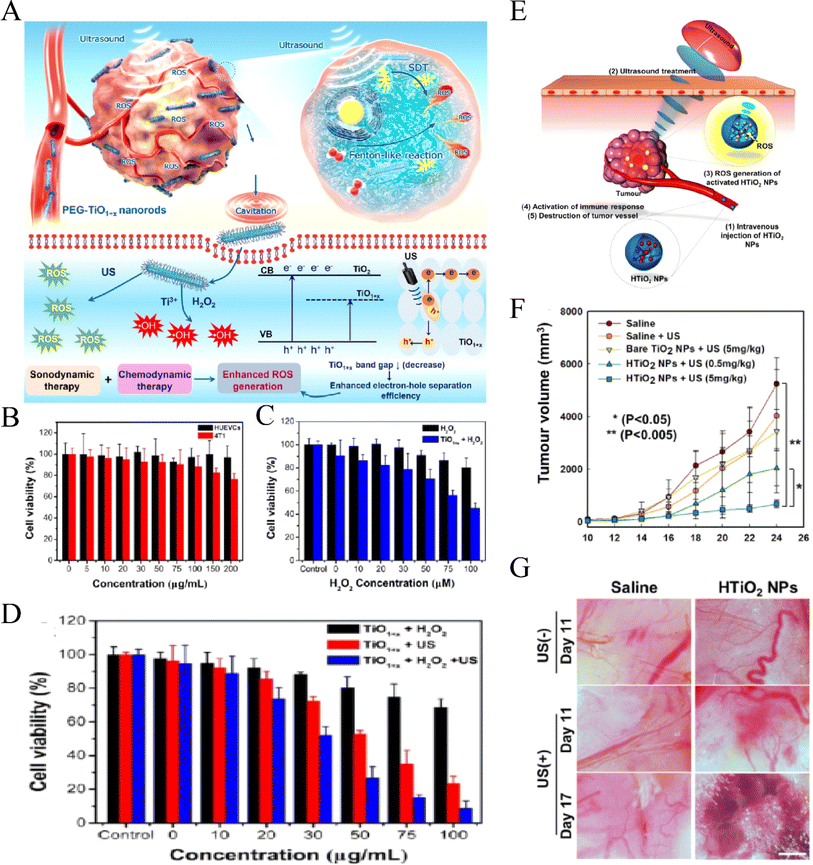 |
| Fig. 4 CDT-enhanced SDT of PEG–TiO1+x NRs. (A) Schematic illustration of using HTiO2 NPs as the sonosensitizer for efficient tumor SDT and (B) relative viabilities of HUVECs and 4T1 cells after being incubated with different concentrations of PEG–TiO1+x NRs; (C) relative viabilities of 4T1 cells incubated with H2O2 at different concentrations and treated with PEG–TiO1+x NRs (50 µg mL−1); (D) relative viabilities of 4T1 cells incubated with PEG–TiO1+x NRs and treated with US irradiation and H2O2 (50 µM) + US irradiation (40 kHz, 5 min).89 “Reproduced from ref. 89 with permission from American Chemical Society, copyright 2020.” (E) Schematic illustration of using HTiO2 NPs as the sonosensitizer for efficient tumor SDT. (F) Changes in tumor volume for each treatment group. (G) Bright-field images of tumor vasculature after SDT with US.91 Reproduced from ref. 91 with permission from Springer Nature, copyright 2016. | |
Aside from TiO2 nanoparticles, sonosensitizers based on Si nanoparticles also demonstrate promising potential for SDT applications due to their high SDT effect and excellent biocompatibility. Upon US irradiation, SiNPs can potentially kill tumor cells through hyperthermia effects93 or mechanical injury.94 Sviridov et al. developed dextran-coated Si nanoparticles (DSiNPs) by mechanically grinding luminescent porous silicon coated with dextran, which can effectively kill HepG2 cells under US radiation.95 This mechanism of this killer cell may be caused by the local hyperthermia effect of Si NPs via efficient absorption of US energy.96 Another common class of inorganic sonosensitizers is magnetic NPs, such as Fe3O4 NPs.97 Based on the Fenton reaction, hydrogen peroxide and iron ions catalyzed the reaction upon US irradiation to generate hydroxyl radicals, which are toxic to cells.98 Additionally, Xu et al. assembled HMME with Fe(III) ions and modified it with phospholipids to make it have high hydrophilicity and stability, so as to have highly efficient ROS production, long-term tumor retention, extremely high accumulation in the tumor and a good synergistic effect of chemotherapy and sonodynamic therapy.99 Besides, magnetic NPs can be used as contrast agents to provide diagnostic and therapeutic possibilities for MRI.100
4 Microbubble-assisted US for drug delivery purposes
SDT not only acts through sonosensitizers, but also generates MBs via US to exert anti-tumor effects. US MBs are usually used in ultrasonic imaging to improve the contrast of images by changing the ultrasonic characteristics of tissues. However, with the development of SDT, more and more roles of US MBs in anti-tumor therapy have been revealed. The cavitation effect of US can break gas-filled MBs up to generate sonoluminescence emission, which further excites the encapsulated sonosensitizer via an energy transfer process and induces the generation of toxic ROS and singlet oxygen (Fig. 5).101 Moreover, US microvesicles can also be used as gene and drug delivery carriers, providing a dual advantage in the diagnosis and treatment of tumors. Lin et al.102 found that new DOX-loaded MBs could be safely applied in rats, resulting in a corresponding increase in the local drug concentration and inhibition of tumor growth. In clinical case studies of patients with locally advanced and inoperable pancreatic cancer, it was found that the combination of US, MBs and chemotherapy significantly increased the treatment cycle and extended the quality of life of patients with pancreatic adenocarcinoma compared with chemotherapy alone.103,104 The blood–brain barrier (BBB) is characterized by tight vascular endothelial connections, which prevent large molecules from entering the interstitional space.105 US-based drug delivery methods using microvesicles can be used to facilitate drug passage through the BBB. It has been reported that phospholipids (SonoVue®) combined with US could successfully improve the response of cancer patients to gemcitabine.106 Dong et al.107 used phospholipid MBs to evaluate BBB opening in the glioblastoma, and the results showed that low-frequency ultrasonic bursts could cause local and reversible BBB damage without adverse long-term effects. Additionally, phospholipid MBs were combined with systemically administered DOX to achieve therapeutic levels in early-stage brain metastasis of breast cancer (BMBC).108 These examples demonstrated the feasibility of using low-intensity US targeted microbubble blasting to enhance targeted drug delivery and the role of US-generated MBs in drug delivery has been gradually highlighted after these studies.
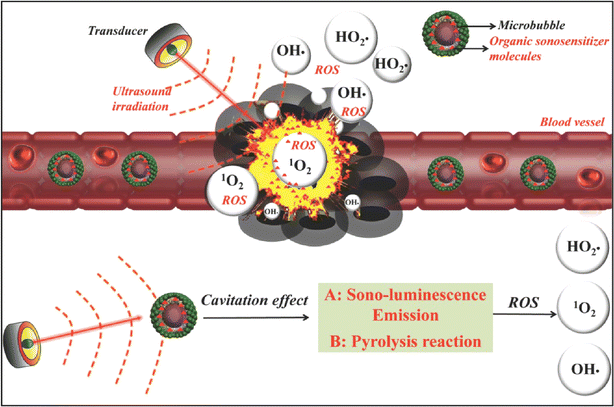 |
| Fig. 5 Schematic illustration of the possible mechanism of microbubble-augmented SDT. The MBs can circulate within the blood vessels and accumulate in tumor tissues. These MBs can produce sono-luminescence emission and undergo a pyrolysis reaction through the cavitation effect and further induce the generation of toxic ROS and singlet oxygen from loaded organic sonosensitizer molecules upon US irradiation, which cause the death of cancer cells afterwards.8 Reproduced from ref. 8 with permission from John Wiley & Sons, copyright 2016. | |
However, conventional US microvesicles (such as SonoVue® microvesicles) have limited drug load and low drug delivery efficiency. Ultrasound-triggered microbubble destruction (UTMD) can play a certain role, but the actual operation is still difficult to control. By contrast, encapsulating cancer drugs in nanobubbles is a new strategy. Due to their small size, nanobubbles can accumulate in tumor sites outside blood vessels through the high-permeability and retention (EPR) effect. Moreover, the cavitation effect of US can be used to break the nanobubbles so as to achieve accurate drug release and improve the efficiency of drug delivery. Lipid MBs are susceptible to low stability during the storage and delivery of guest molecules such as sonosensitizers. This instability poses a challenge to continuous SDT and restricts its efficiency. To solve this critical issue, McEwan et al.109 used polylactic acid – hydroxyacetic acid copolymer (PLGA) to replace the lipid layer of MBs so as to improve the stability of MBs. Li et al.110 developed nanosystems capable of co-delivering the sonosensitizer methylene blue (MB) and the magnetic resonance contrast agent gadodiamide (GD-DTPA-BMA). These nanosystems utilized PLGA nanoparticles that were modified with a tumor homing penetrating peptide (F3) and PEG to enhance the aggregation and penetration of nanoparticles at the tumor site. The results demonstrated that F3-PLGA@MB/Gd NPs exhibited a superior ability to induce tumor cell adhesion and apoptosis. Hou et al.76 designed a conjugated Bengal rose conjugate to prepare rose-colored Bengal microbubbles (RB-MBs) with a high drug load and high contrast enhancement in ultrasonic imaging. Abdalkader et al.111 developed a novel phospholipid-based microbubble formulation containing DOX and perfluoropropane gas (DLMB) and demonstrated that DLMBs combined with US irradiation significantly inhibited the growth of B16F10 melanoma cells in mice.
Additionally, the sonodynamic effect caused by US can generate ROS, and ROS sensitive drugs or groups can be used as media to construct the nanosystem. Drugs can be decomposed and released under the action of ROS generation by co-loaded sonosensitizers, which is a method of indirectly using US to promote drug release. Kim et al.112 prepared DOX coordination titania nanoparticles (TNPs) using phenylborate bonds. Due to the ROS dissociation properties of the phenylborate bond, the loaded DOX is easy to release upon US radiation, thus achieving an anti-tumor effect. By encapsulating DOX in a PH/ROS responsive polymer micelle, Zhang et al.113 constructed a nano-drug delivery platform with PH-triggered charge-reversal capability and ROS-induced drug release, and the results showed that the system has excellent tumor inhibition ability in vivo. Therefore, the development of a US-guided nano-microbubble drug delivery system will provide a new direction for drug targeted therapy.
5 Nanomaterials enhance SDT
With the deepening of tumor research, it has been found that the insufficient blood supply inside solid tumors and the proliferation of tumor cells consume a large amount of oxygen, leading to a hypoxic microenvironment in tumors, which will affect the therapeutic effects of radiotherapy, chemotherapy, PDT, immunotherapy and SDT. Therefore, it is of great practical significance to develop efficient and safe tumor oxygenation technology, change tumor microenvironments, and achieve enhanced tumor therapeutic effects. Based on the natural enzyme system of red blood cells (RBCs), Li et al.114 wrapped AGS quantum dots (QDs) on the RBC follicular membrane, prepared biomimetic NPs (QD@P)Rs, and used the anti-tumor drug PEITC to increase the concentration of HO in tumor cells. The experimental results showed that (QD@P) Rs has good biocompatibility and the ability to extend blood circulation and can effectively alleviate the degree of tumor hypoxia. Under the guidance of fluorescence imaging, (QD@P) Rs generates ROS under the action of US to induce tumor cell death, and the increase in oxygen content significantly enhances the effects of SDT. Pan et al.115 prepared MOF-derived two-layer hollow manganese silicate NPs (DHMS) with high ROS yield upon US radiation. Moreover, DHMS can produce oxygen in the tumor microenvironment, which is helpful to overcome hypoxia in solid tumors and thus improve treatment efficiency. Additionally, Chen et al. developed an efficient oxygen-self-produced sonodynamic therapy (SDT) nanoplatform for the treatment of hypoxic pancreatic cancer. This nanoplatform involved a modified fluorocarbon (FC)-chain-mediated oxygen delivery protocol to achieve highly effective SDT.116 Besides, US + IR780@O2+FHMON can significantly reduce tumor volume compared to other groups.
6 SDT-based synergistic therapy
As a new method for treating malignant tumors, SDT has great potential for application. However, the effectiveness of SDT alone in eliminating tumors in vivo remains unsatisfactory in its current state. Moreover, clinical translation of sonosensitizers is limited by biosafety concerns. While nanostructure-enhanced sonosensitizers hold promise and represent a new direction for developing nanotechnology-based tools to treat various diseases, this approach is not universally applicable to all sonosensitizers. On the other hand, the efficacy of monotherapy for tumors is weakened by current treatment limitations. Therefore, combining SDT with other therapies such as chemotherapy, PDT, and PTT based on nanomaterials is considered the best strategy for treating cancer. This integration of complementary and synergistic effects between different therapeutic approaches is crucial for achieving optimal results.117
6.1 Combination of SDT and PDT (SPDT)
As a new treatment method, PDT has been gradually applied to the treatment of various cancers since 1990. However, PDT inevitably limits its application due to its low penetration of tissues and skin phototoxicity. SDT is developed on the basis of PDT, and the first generation of sonosensitizers is photosensitizers. Therefore, some sensitizers with both sonosensitivity and photosensitivity may have a synergistic anti-tumor effect through the combined action of SDT and PDT and reduce the toxic and side effects in single use. Liu et al.118 evaluated the combined effect of SDT and PDT on breast cancer in vitro and in vivo by using DVDMS, and the results showed that the therapeutic effects of DVDMS-SPDT on tumors were significantly better than those of single use and had no significant effect on the main organs in tumor-bearing mice. Miyoshi et al. used TiO2 NPs and 5-ALA as sonosensitizers and photosensitizers respectively in combination with SDT and PDT to treat cancer. The results showed that, compared with monotherapy, the anti-tumor efficiency of combination therapy was significantly improved. Moreover, SDT followed by PDT had a greater effect on cancer treatment.119 Jin and co-workers used a newly developed photosensitizer, PH-1126, and a commonly used sonosensitizer, ATX-70, to evaluate the efficacy of PDT combined with SDT on tumor-bearing mice. They found that the combination of SDT and PDT (92% and 98% tumor inhibition efficiency) was significantly better than the single treatment (27% and 77% tumor inhibition efficiency).120
6.2 Combination of SDT and chemodynamic therapy (CDT)
CDT is an emerging tumor treatment method based on the Fenton reaction, which produces a large amount of ROS and thus has a killing effect on tumor cells. Moreover, US can greatly improve the mass transfer rate and chemical reaction rate through the acoustic cavitation effect and thus improves the Fenton reaction efficiency and produces a synergistic anti-tumor effect.121 Chen et al.100 developed the PH-responsive GOD@caco-Fe3O4 particle as a catalytic nanodrug for CDT (Fig. 6A–C).Upon US irradiation, tumor Fenton reaction efficiency can be significantly improved to produce a large number of highly toxic hydroxyl radicals to kill cancer cells. Zhang et al.122 reported for the first time novel hypoxic-responsive Cu-Mof NPs for chemokinetic and sonodynamic therapy (CDT/SDT)(Fig. 6D–H).Large-size Cu-Mof NPs exhibit good stability under constant oxygen partial pressure, aggregation at tumor sites, and rapid degradation of Cu and Ce6 in an anoxic tumor microenvironment (TME), significantly enhancing tumor penetration and the SDT/CDT synergistic effect, thus achieving selective and effective killing of MCF-7 cells. Lin et al.123 found that Janus Au-Mno nanoparticles (JNPs) with dual responses to US and glutathione (GSH) vesicles were coated with PEG and reactive oxygen sensitive polymers (Fig. 6I–M). After US irradiation, the vesicles were decomposed into small Janus Au-Mno nanoparticles (NPs) with the ability to promote penetration. Subsequently, GSH-induced MnO degradation simultaneously releases smaller Au NPs as a large number of void nucleation sites and Mn is used for CDT, leading to an increase in the production of ROS. Inhibition of orthotopic liver tumor growth was obtained by synergistic SDT/CDT.
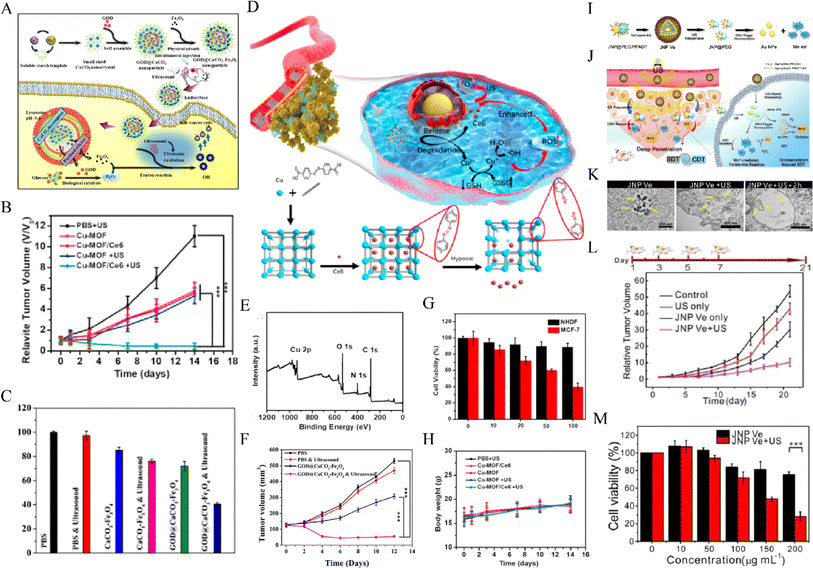 |
| Fig. 6 (A) The synthetic procedure for GOD@CaCO3–Fe3O4 particles and schematic illustration of the sequential catalytic-therapeutic mechanism of GOD@CaCO3–Fe3O4 particles. (B) Tumor volumes of mice in different groups within 12 days of treatments. (C) Cytotoxicity profiles of A549 cells incubated with GOD@CaCO3–Fe3O4 or CaCO3–Fe3O4 particles.100 Reproduced from ref. 100 with permission from Elsevier, copyright 2019. (D) Schematic illustration of the synthetic procedure and hypoxia-responsive copper metal–organic framework nanosystem for improved cancer therapy. (E) XPS spectra of Cu-MOF. (F) Cell viability of NHDF cells and MCF-7 cells incubated with Cu-MOF/Ce6 at different concentrations (10, 20, 50 100 µg mL−1). (G) Relative tumor volumes and (H) body weight of mice that received different treatments.122 Reproduced from ref. 122 with permission from Elsevier, copyright 2020. Illustration of (I) self‐assembly of amphiphilic Janus Au–MnO NPs into functional vesicles and (J) their sequential US and GSH‐induced disassembly into small JNPs with deep tumor penetration for synergistic SDT/CDT. (K) Images of ultrathin sections of MCF-7 cells treated with JNP Ve, JNP Ve with US, and JNP Ve with US and GSH decomposition. (L) Tumor volume curves in the different groups. The mice were treated on days 1, 3, 5, and 7. (M) The viability of cells treated with different concentrations of JNP Ve with or without US irradiation.123 Reproduced from ref. 123 with permission from John Wiley & Sons, copyright 2020. | |
6.3 Combination of photothermal-sonodynamic therapy
Tumor cells can be inactivated through heating the tumor area. In view of the advantages of US, such as focalization, controllability, maintaining a sufficient tissue attenuation coefficient when reaching the deep target tumor tissue, etc., the use of US induced hyperthermia may show greater advantages.124 Additionally, hyperthermic cells can also be killed by a non-thermal effect (cavitation and direct effect) of US under various gas conditions.125 Yang et al.126 reported that compared with SDT alone, SDT combined with 5-ALA mediated hyperthermia could inhibit the growth of human glioma more effectively by in vitro and in vivo experiments. As a new kind of light and sound responsive nanomaterial, ALA-lipid/PLGA MB-mediated SDT was a promising treatment for pancreatic cancer. Gong et al.127 utilized liquid-phase exfoliation to produce titanium hydride (TiH 1.924) nanodots from its powder form. They discovered that these TiH nanodots exhibit a highly efficient acoustic sensitization effect and can produce ROS under US irradiation. Additionally, they possess a strong near-infrared (NIR) absorption capacity, making them an effective photothermal agent. Therefore, by using the mild photothermal effect to boost tumor blood flow and improve oxygenation, a remarkable synergistic therapeutic effect was achieved in the combined photothermal and sonodynamic therapy.
6.4 Combination of chemotherapy and SDT
Chemotherapy plays a very important role in the clinical treatment of tumors, and improving the sensitivity of tumors to chemotherapy drugs has always been a hot topic in clinical research. SDT can down-regulate the expression of p-glycoproteins and drug-resistance-related proteins in drug-resistant cells, increase the sensitivity of cells to drugs, and reverse multidrug resistance in tumors. In addition, SDT can also lead to changes in cell membrane permeability through enhanced sonoporation,128 increasing the targeted uptake of chemotherapy drugs by tumor cells and reducing toxicity and side effects of normal cells, thereby increasing the effect of chemotherapy. In recent years, thanks to the rapid development of nanotechnology, NP enhanced SDT can accelerate the release of chemotherapy drugs from different nano-carriers and release drugs as needed, which can effectively increase the anti-tumor effect of chemotherapy drugs. Research has shown that mesoporous NPs with adjustable pore size and large surface area are ideal materials for reactive drug release systems.12,129
DOX is a type of anthracycline antibiotic that works by inserting itself between base pairs, thereby intercalating DNA. Yang et al.130 synthesized HPDF NPs by complexing HP with DOX to form the hydrophobic core and coating the surface with Pluronic F68 to create the hydrophilic shell. Under US irradiation, HPDF NPs exhibited remarkable synergistic effects on the cytotoxicity, apoptosis, and cell cycle arrest of HepG2 cells. Moreover, they significantly reversed drug resistance in Nanog-positive tumor stem cells (CSCs) in HCC. In nude mice with HCC tumors, HPDF NPs combined with US irradiation demonstrated excellent targeted delivery ability for HCC and significantly inhibited tumor growth, angiogenesis, and collagen deposition. Mesoporous silica NPs (MSN) have been extensively investigated as drug carriers due to their good biocompatibility and drug carrying capacity. Xu et al.72 synthesized and characterized mesoporous silica NPs (MSN-Dox-CE6) loaded with DOX and the sonosensitizer Ce6. They confirmed that MSN-Dox-CE6 nanocomposites enhanced the anti-tumor effects of DOX and SDT upon US irradiation. In addition, MSN can also be used as an effective sonosensitizer to induce apoptosis after US treatment. In order to improve the tumor targeting ability of Dox@MSN, Ding et al.131 covered the surface of Dox@MSN with a photoinitiated polymerized cross-linked methacrylate hyaluronic acid (M-HA) gel, resulting in the degradation of the M-HA shell by hyaluronidase (HAase), which is concentrated in the tumour environment, resulting in the release of DOX@MSN. In vivo and in vitro studies demonstrated that DOX@MSN-HA not only possessed excellent accumulation ability in tumor cells, but also showed good biocompatibility and satisfactory anti-tumor activity in tumor-bearing mice.
NPs can not only increase the sensitivity of tumors to chemotherapeutic drugs, but also, due to their good biocompatibility and low toxicity, be used as carriers of chemotherapeutic drugs to target tumors and release drugs as needed, thus increasing the efficacy of drugs. Shi et al.132 developed a new type of mesoporous TiO2 NP (MTN) with a well-defined pore size and low cytotoxicity. They achieved high-efficiency loading of the chemotherapeutic drug docetaxel (DTX) into the pores of mesoporous titanium dioxide nanoparticles (MTN), while attaching β-cyclodextrin (β-CD), a large gatekeeper, to the outer surface of MTN via a ROS-sensitive linker to block the pores (MTN@DTX-CD). Upon US radiation, MTN generates a significant amount of ROS, leading to the cleavage of ROS-sensitive linkers and the rapid release of DTX. Moreover, ROS production can be utilized for tumor-specific SDT. Additionally, MTN@DTX-CD can help minimize spleen and liver toxicity in tumor-bearing mice, significantly reducing DTX-associated side effects.
6.5 Combination of immunotherapy and SDT
In recent years, the vigorous development of immunotherapy has brought a new dawn to the treatment of tumors. However, immunotherapy still has its limitations, such as immune cell depletion and immune escape at the tumor site. Combination therapy is a new form of tumor treatment and has a certain prospect of clinical transformation in cancer treatment. A triphenylphosphonium (TPP) -encapsulated nano-metal–organic framework (nMOF) structure [Zr-TCPP(TPP)/R837@M] was constructed to generate a homologous mitochondrial targeting platform with a high sonosensitizer loading rate. To reduce TME immunosuppression by inducing immunogenic cell death (ICD) after US irradiation and combining with anti-CTLA-4 ICI therapy, so as to obtain stronger anti-tumor efficacy compared with single therapy.133 Chen et al. conducted experiments on multiple tumor models, combining co-encapsulation of sonosensitizers (hematoporphyrin monomethyl ether (HMME)) and immune adjuvants (imiquimod (R837)) to augment SDT with anti-PD-L1, resulting in an anti-tumor response that not only halted primary tumor progression but also prevented lung metastasis.50 The coordination of Fe3+ with COF gives the nanoparticles chemokinetic therapy (CDT) properties and glutathione (GSH) depletion capacity, and the resulting nanoscale sonosensitizer (PgP@Fe–COF NPs) has enhanced sonodynamic properties. In addition, researchers found that the combination of PgP@Fe–COF NPs with anti-PD-L1 antibody had more efficient tumor immunotherapy.134 Together, these findings indicate the efficacy and feasibility of SDT combined with immunotherapy, and more clinical studies are needed to promote its clinical translation.
7 Conclusions
Herein, we briefly discuss the anti-tumor mechanism of SDT and the application of SDT based nanomaterials in the treatment of malignant tumors. Compared with traditional treatment methods, SDT has great potential in anti-tumor therapy due to its advantages of deep invasion and fewer side effects. With the rapid development of nanomaterials, nanotechnology-enabled SDT has been getting a lot of attention, playing a more and more important role in anti-tumor effects. As commonly used nanomaterials, NPs can be combined with sonosensitizers to increase the water solubility of sonosensitizers and increase the accumulation of sonosensitizers in tumor sites through an enhanced permeability and retention (EPR) effect, thus increasing the efficacy of SDT. In addition, NPs themselves can also be used as sonosensitizers in SDT or as carriers to transmit sonosensitizers. Nano-sonosensitizers obviously improve the efficiency of SDT, but there is still a lack of research on the biosafety of nano-sonosensitizers, which hinders further clinical transformation. In contrast, inorganic sonosensitizers, although with good chemical/biological stability and multifunctions, may be harmful due to their poor biodegradability. For example, carbon nanomaterials135 can cause coagulation of macrophage chromatin and contraction of organelles and vesicles. SiO2 NPs136 have significant toxicity on human umbilical vein endothelial cells, liver, spleen, kidney, lung and other organs. Therefore, it is a great challenge to improve the biosafety of nanosonosensitizers in clinical application of SDT. This may require more systematic and long-term research into nanotoxicology and the development of new and efficient sonosensitizers. Additionally, single therapy cannot meet the needs of cancer patients, so combination therapy is very important. Strategies combining SDT with chemotherapy, thermotherapy and PDT have shown promise, and many studies have demonstrated synergies between them,137 but there is still a long way to go before they can be applied in the clinic, requiring multidisciplinary cooperation to ensure the effectiveness and safety of clinical transformation.
Therefore, despite considerable efforts, no very efficient nano-sonosensitizers have yet been able to be used in clinics. The main goal of this review is to systematically review the mechanism and application of nanomaterials in SDT and provide inspiration for the screening and further exploration of safe, reliable and efficient sonosensitizers in the future. Moreover, future research on the combination therapy of SDT is also critical, which may take a great step towards the treatment of tumors and bring a new dawn for the cure of tumors.
Data availability
No data were used for the research described in the article.
Conflicts of interest
The authors have declared that no competing interest exists.
Acknowledgements
This study was supported by grants 82160341 and 82260345 from the National Natural Science Foundation of China.
References
- A. K. Bhatta,
et al., Therapeutic effect of Imiquimod enhanced ALA-PDT on cutaneous squamous cell carcinoma, Photodiagn. Photodyn. Ther., 2018, 23, 273 CrossRef CAS PubMed.
- P. Huang, Y. Zhang, Y. Liao, Q. Tang and J. Lin, Biomimetic nanoemulsion for synergistic photodynamic‐immunotherapy against hypoxic breast tumor, Angew. Chem., Int. Ed., 2021, 10647–10653 Search PubMed.
- H. Tsuru, H. Shibaguchi, M. Kuroki, Y. Yamashita and M. Kuroki, Tumor growth inhibition by sonodynamic therapy using a novel sonosensitizer, Free Radical Biol. Med., 2012, 53, 464 CrossRef CAS PubMed.
- X. Guan, H. A. Yin, X. O. Xu, G. Xu and K. Zhang, Tumor Metabolism Engineered Composite Nanoplatforms Potentiate Sonodynamic Therapy via Reshaping Tumor Microenvironment and Facilitating Electron–Hole Pairs’ Separation, Adv. Funct. Mater., 2020, 2000326 CrossRef CAS.
- A. P. McHale, J. F. Callan, N. Nomikou, C. Fowley and B. Callan, Sonodynamic Therapy: Concept, Mechanism and Application to Cancer Treatment, Adv. Exp. Med. Biol., 2016, 880, 429 CrossRef CAS PubMed.
- J. Huang,
et al., Nanodrug with Dual-Sensitivity to Tumor Microenvironment for Immuno-Sonodynamic Anti-Cancer Therapy - ScienceDirect, Biomater., 2021, 120636 CrossRef CAS PubMed.
- H. Shibaguchi, H. Tsuru, M. Kuroki and M. Kuroki, Sonodynamic cancer therapy: a non-invasive and repeatable approach using low-intensity ultrasound with a sonosensitizer, Anticancer Res., 2011, 31, 2425 Search PubMed.
- X. Qian, Y. Zheng and Y. Chen, Micro/Nanoparticle-Augmented Sonodynamic Therapy (SDT): Breaking the Depth Shallow of Photoactivation, Adv. Mater., 2016, 28, 8097 CrossRef CAS PubMed.
- K. G. Baker, V. J. Robertson and F. A. Duck, A Review of Therapeutic Ultrasound, Journal of Womens Health Physical Therapy, 2010, 34, 111 CrossRef.
- I. Rosenthal, Sonodynamic therapy? a review of the synergistic effects of drugs and ultrasound, Ultrason. Sonochem., 2004, 349–363 CrossRef CAS PubMed.
- W. Hiraoka, H. Honda, L. Feriljr, N. Kudo and T. Kondo, Comparison between sonodynamic effect and photodynamic effect with photosensitizers on free radical formation and cell killing, Ultrason. Sonochem., 2006, 13, 535 CrossRef CAS PubMed.
- S. R. Sirsi and M. A. Borden, State-of-the-art materials for ultrasound-triggered drug delivery, Adv. Drug Delivery Rev., 2014, 72, 3 CrossRef CAS PubMed.
- S. Hilgenfeldt, S. Grossmann and D. Lohse, A simple explanation of light emission in sonoluminescence, Nature, 1999, 398, 402 CrossRef CAS.
- A. Sazgarnia,
et al., Detection of sonoluminescence signals in a gel phantom in the presence of Protoporphyrin IX conjugated to gold nanoparticles, Ultrasonics, 2013, 53, 29 CrossRef CAS PubMed.
- K. Hachimine,
et al., Sonodynamic therapy of cancer using a novel porphyrin derivative, DCPH-P-Na(I), which is devoid of photosensitivity, Cancer Sci., 2007, 98, 916 CrossRef CAS PubMed.
- K. Zhang, H. A. Li, J. I. Lang, X. O. Li and C. Xu, Quantum Yield‐Engineered Biocompatible Probes Illuminate Lung Tumor Based on Viscosity Confinement‐Mediated Antiaggregation, Adv. Funct. Mater., 2019, 29, 1905124 CrossRef CAS.
- K. Zhang,
et al., Coordination-Responsive Longitudinal Relaxation Tuning as a Versatile MRI Sensing Protocol for Malignancy Targets, Adv. Sci., 2018, 5, 1800021 CrossRef PubMed.
- S. Wang, N. Zeng, Q. Zhang, M. Chen and Q. Huang, Nanozyme Hydrogels for Self-Augmented Sonodynamic/Photothermal Combination Therapy, Front. Oncol., 2022, 12, 888855 CrossRef CAS PubMed.
- Y. Lai, N. Lu, A. Ouyang, Q. Zhang and P. Zhang, Ferroptosis promotes sonodynamic therapy: a platinum(ii)–indocyanine sonosensitizer, Chem. Sci., 2022, 13, 9921 RSC.
- J. Wang,
et al., 'Mito-Bomb': a novel mitochondria-targeting nanosystem for ferroptosis-boosted sonodynamic antitumor therapy, Drug Delivery, 2022, 29, 3111 CrossRef CAS PubMed.
- S. Bhattacharya, K. R. M, J. Priyadarshani, R. Ganguly and S. Chakraborty, Targeting Magnetic Nanoparticles in Physiologically Mimicking Tissue Microenvironment, ACS Appl. Mater. Interfaces, 2022, 14, 31689 CrossRef CAS PubMed.
- A. Yildirim, R. Chattaraj, N. T. Blum and A. P. Goodwin, Understanding Acoustic Cavitation Initiation by Porous Nanoparticles: Toward Nanoscale Agents for Ultrasound Imaging and Therapy, J. Mater. Chem., 2016, 28, 5962 CrossRef CAS PubMed.
- J. Tang,
et al., Multiple stimuli-responsive nanosystem for potent, ROS-amplifying, chemo-sonodynamic antitumor therapy, Bioact. Mater., 2022, 15, 355 CAS.
- P. H. Zhao,
et al., Aggregation-Enhanced Sonodynamic Activity of Phthalocyanine-Artesunate Conjugates, Angew. Chem., 2022, e202113506 CAS.
- J. Zhao,
et al., ROS-Activated nanoscale coordination polymers for enhanced ultrasound-mediated therapy for the treatment of cancer, Acta Biomater., 2022, 143, 372–380 CrossRef CAS PubMed.
- S. Guan,
et al., Intracellular Mutual Amplification of Oxidative Stress and Inhibition Multidrug Resistance for Enhanced Sonodynamic/Chemodynamic/Chemo Therapy, Small, 2022, 18, e2107160 CrossRef PubMed.
- J. A. Simplicio,
et al., Contribution of oxidative stress and prostanoids in endothelial dysfunction induced by chronic fluoxetine treatment, Cancers, 2022, 14(8), 2036 CrossRef PubMed.
- C. Huang,
et al., Interference With Redox Homeostasis Through a G6PD-Targeting Self-Assembled Hydrogel for the Enhancement of Sonodynamic Therapy in Breast Cancer, Front. Chem., 2022, 10, 908892 CrossRef CAS PubMed.
- C. Mcewan,
et al., Oxygen carrying microbubbles for enhanced sonodynamic therapy of hypoxic tumours, J. Controlled Release, 2015, 51–56 CrossRef CAS PubMed.
- X. Sun, Y. Shu, G. Ye, C. Wu and J. Zhang, Histone deacetylase inhibitors inhibit cervical cancer growth through Parkin acetylation-mediated mitophagy, Acta Pharm. Sin. B, 2021, 838–852 Search PubMed.
- K. Zhang,
et al., Ultrasound-Triggered Nitric Oxide Release Platform Based on Energy Transformation for Targeted Inhibition of Pancreatic Tumor, ACS Nano, 2016, 10816 CrossRef CAS PubMed.
- W. Li,
et al., Nanodrug-loaded Bifidobacterium bifidum conjugated with anti-death receptor antibody for tumor-targeted photodynamic and sonodynamic synergistic therapy, Acta Biomater., 2022, 146, 341 CrossRef CAS PubMed.
- H. Gao,
et al., pH-Responsive Nanoparticles for Enhanced Antitumor Activity by High-Intensity Focused Ultrasound Therapy Combined with Sonodynamic Therapy, Int. J. Nanomed., 2022, 17, 333 CrossRef CAS PubMed.
- M. Bismuth,
et al., Acoustically Detonated Microbubbles Coupled with Low Frequency Insonation: Multiparameter Evaluation of Low Energy Mechanical Ablation, Bioconjugate Chem., 2022, 33, 1069 CrossRef CAS PubMed.
- F. Zhuang,
et al., Sequential Ultrasound-Triggered and Hypoxia-Sensitive Nanoprodrug for Cascade Amplification of Sonochemotherapy, ACS Nano, 2022, 16, 5439 CrossRef CAS PubMed.
- S. J. Soenen,
et al., Cellular toxicity of inorganic nanoparticles: Common aspects and guidelines for improved nanotoxicity evaluation, Nano Today, 2011, 6, 446 CrossRef CAS.
- A. Ivask,
et al., Toxicity of 11 Metal Oxide Nanoparticles to Three Mammalian Cell Types In Vitro, Curr. Top. Med. Chem., 2015, 15, 1914 CrossRef CAS PubMed.
- M. Carofiglio,
et al., Iron-Doped ZnO Nanoparticles as Multifunctional Nanoplatforms for Theranostics, Nanomaterials, 2021, 11, 2628 CrossRef CAS PubMed.
- Z. Gao,
et al., Sonodynamic therapy inhibits angiogenesis and tumor growth in a xenograft mouse model, Cancer Lett., 2013, 335, 93 CrossRef CAS PubMed.
- W. Xiong,
et al., A new sensitizer DVDMS combined with multiple focused ultrasound treatments: an effective antitumor strategy, Sci. Rep., 2015, 5, 17485 CrossRef PubMed.
- D. Song,
et al., Study of the mechanism of sonodynamic therapy in a rat glioma model, OncoTargets Ther., 2014, 7, 1801 CrossRef PubMed.
- J. Yao,
et al., Sonodynamic Therapy Suppresses Neovascularization in Atherosclerotic Plaques via Macrophage Apoptosis-Induced Endothelial Cell Apoptosis, JACC: Basic Transl. Sci., 2020, 5, 53 Search PubMed.
- K. Zhang,
et al., Extravascular gelation shrinkage-derived internal stress enables tumor starvation therapy with suppressed metastasis and recurrence, Nat. Commun., 2019, 10, 5380 CrossRef PubMed.
- J. H. Hwang,
et al., Vascular effects induced by combined 1-MHz ultrasound and microbubble contrast agent treatments in vivo, Ultrasound Med. Biol., 2005, 31, 553 CrossRef PubMed.
- Y. Zhao,
et al., Tumor Microenvironment‐Responsive Cu/CaCO3 ‐Based Nanoregulator for Mitochondrial Homeostasis Disruption‐Enhanced Chemodynamic/Sonodynamic Therapy, Small, 2022, 18, 2204047 CrossRef CAS PubMed.
- P. Meng,
et al., Hematoporphyrin monomethyl ether mediated photodynamic therapy inhibits oral squamous cell carcinoma by regulating the P53-miR-21-PDCD4 axis via singlet oxygen, J. Lasers Med. Sci., 2022, 37, 1 CrossRef PubMed.
- J. Yao,
et al., Low‐Intensity Focused Ultrasound‐Responsive Ferrite‐Encapsulated Nanoparticles for Atherosclerotic Plaque Neovascularization Theranostics, Adv. Sci., 2021, 8, 2100850 CrossRef CAS PubMed.
- D. Hao, Y. Song, Z. Che and Q. Liu, Calcium overload and in vitro apoptosis of the C6 glioma cells mediated by sonodynamic therapy (hematoporphyrin monomethyl ether and ultrasound), Cell Biochem. Biophys., 2014, 70, 1445 CrossRef CAS PubMed.
- X. Wang, T. J. Lewis and D. Mitchell, The tumoricidal effect of sonodynamic therapy (SDT) on S-180 sarcoma in mice, Integr. Cancer Ther., 2008, 7, 96 CrossRef CAS PubMed.
- W. Yue,
et al., Checkpoint blockade and nanosonosensitizer-augmented noninvasive sonodynamic therapy combination reduces tumour growth and metastases in mice, Nat. Commun., 2019, 10, 2025 CrossRef PubMed.
- X. J. L. S. Yifei Yin, Continuous inertial cavitation evokes massive ROS for reinforcing sonodynamic therapy and immunogenic cell death against breast carcinoma, Nano Today, 2020, 101009 Search PubMed.
- X. Su,
et al., Sonodynamic therapy induces the interplay between apoptosis and autophagy in K562 cells through ROS, Int. J. Biochem. Cell Biol., 2015, 60, 82 CrossRef CAS PubMed.
- Q. Feng,
et al., Cancer Cell Membrane-Biomimetic Nanoplatform for Enhanced Sonodynamic Therapy on Breast Cancer via Autophagy Regulation Strategy, ACS Appl. Mater. Interfaces, 2019, 11, 32729 CrossRef CAS PubMed.
- R. Liu, Q. Zhang, Y. Lang, Z. Peng and L. Li, Sonodynamic therapy, a treatment developing from photodynamic therapy, Photodiagn. Photodyn. Ther., 2017, 19, 159 CrossRef PubMed.
- N. Yumita, R. Nishigaki, K. Umemura and S. Umemura, Hematoporphyrin as a sensitizer of cell-damaging effect of ultrasound, Jpn. J. Cancer Res., 1989, 80, 219 CrossRef CAS PubMed.
- N. Yumita, R. Nishigaki and S. Umemura, Sonodynamically induced antitumor effect of Photofrin II on colon 26 carcinoma, J. Cancer Res. Clin. Oncol., 2000, 126, 601 CrossRef CAS PubMed.
- H. Xu,
et al., Nanoparticles in sonodynamic therapy: state of the art review, RSC Adv., 2016, 10, 50697–50705 RSC.
- N. Yumita,
et al., Involvement of reactive oxygen species in sonodynamically induced apoptosis using a novel porphyrin derivative, Theranostics, 2012, 2, 880 CrossRef CAS PubMed.
- K. Hachimine,
et al., Sonodynamic therapy of cancer using a novel porphyrin derivative, DCPH-P-Na(I), which is devoid of photosensitivity, Cancer Sci., 2007, 98, 916 CrossRef CAS PubMed.
- N. Yumita, N. Okuyama, K. Sasaki and S. Umemura, Sonodynamic therapy on chemically induced mammary tumor: pharmacokinetics, tissue distribution and sonodynamically induced antitumor effect of gallium-porphyrin complex ATX-70, Cancer Chemother. Pharmacol., 2007, 60, 891 CrossRef CAS PubMed.
- N. Miyoshi, V. Misík and P. Riesz, Sonodynamic toxicity of gallium-porphyrin analogue ATX-70 in human leukemia cells, Radiat. Res., 1997, 148, 43 CrossRef CAS PubMed.
- N. Yumita, K. Okudaira, Y. Momose and S. Umemura, Sonodynamically induced apoptosis and active oxygen generation by gallium-porphyrin complex, ATX-70, Cancer Chemother. Pharmacol., 2010, 66, 1071 CrossRef CAS PubMed.
- B. Mai,
et al., Correction to Smart Hydrogel-Based DVDMS/bFGF Nanohybrids for Antibacterial Phototherapy with Multiple Damaging Sites and Accelerated Wound Healing, ACS Appl. Mater. Interfaces, 2021, 13, 24341 CrossRef CAS PubMed.
- B. Mai, X. Wang, Q. Liu, K. Zhang and P. Wang, The Application of DVDMS as a Sensitizing Agent for Sono-/Photo-Therapy, Front. Pharmacol., 2020, 11, 19 CrossRef CAS PubMed.
- H. Sun,
et al., Apoptosis-Promoting Effects of Hematoporphyrin Monomethyl Ether-Sonodynamic Therapy (HMME-SDT) on Endometrial Cancer, PLoS One, 2015, 10, e137980 Search PubMed.
- Z. Gong and Z. Dai, Design and Challenges of Sonodynamic Therapy System for Cancer Theranostics: From Equipment to Sensitizers, Adv. Sci., 2021, 8, 2002178 CrossRef CAS PubMed.
- M. J. Chen,
et al., Ultrasound triggered drug delivery for mitochondria targeted sonodynamic therapy, J. Drug Delivery Sci. Technol., 2017, 501–507 CrossRef.
- H. Leroy,
et al., Is interstitial photodynamic therapy for brain tumors ready for clinical practice? A systematic review, Photodiagn. Photodyn. Ther., 2021, 36, 102492 CrossRef CAS PubMed.
- A. Sazgarnia, A. Shanei, N. T. Meibodi, H. Eshghi and H. Nassirli, A novel nanosonosensitizer for sonodynamic therapy: in vivo study on a colon tumor model, J. Ultrasound Med., 2011, 30, 1321 CrossRef PubMed.
- K. Liang,
et al., Intelligent Nanocomposites with Intrinsic Blood-Brain-Barrier Crossing Ability Designed for Highly Specific MR Imaging and Sonodynamic Therapy of Glioblastoma, Small, 2020, 16, e1906985 CrossRef PubMed.
- L. Li,
et al., Carrier-Free Nanoplatform via Evoking Pyroptosis and Immune Response against Breast Cancer, ACS Appl. Mater. Interfaces, 2022, 452–468 Search PubMed.
- P. Xu,
et al., Therapeutic Effect of Doxorubicin-Chlorin E6-Loaded Mesoporous Silica Nanoparticles Combined with Ultrasound on Triple-Negative Breast Cancer, Int. J. Nanomed., 2020, 15, 2659 CrossRef CAS PubMed.
- S. Chen,
et al., Highly biocompatible chlorin e6-poly(dopamine) core–shell nanoparticles for enhanced cancer phototherapy, Nanoscale Adv., 2022, 4, 4617 RSC.
- X. Sun, Z. Ji and S. He, SHG-enhanced NIR-excitedin vitro photodynamic therapy using composite nanoparticles of barium titanate and rose Bengal, RSC Adv., 2019, 9, 8056 RSC.
- H. J. Chen,
et al., Synthesis and biological characterization of novel rose bengal derivatives with improved amphiphilicity for sono-photodynamic therapy, Eur. J. Med. Chem., 2018, 145, 86 CrossRef CAS PubMed.
- R. Hou,
et al., In situ conversion of rose bengal microbubbles into nanoparticles for ultrasound imaging guided sonodynamic therapy with enhanced antitumor efficacy, Biomater. Sci., 2020, 8, 2526 RSC.
- N. Nomikou,
et al., Microbubble-sonosensitiser conjugates as therapeutics in sonodynamic therapy, Chem. Commun., 2012, 48, 8332 RSC.
- N. Sakusabe,
et al., Enhanced sonodynamic antitumor effect of ultrasound in the presence of nonsteroidal anti-inflammatory drugs, Jpn. J. Cancer Res., 1999, 90, 1146 CrossRef CAS PubMed.
- K. Okada,
et al., Enhanced antitumor effect of ultrasound in the presence of piroxicam in a mouse air pouch model, Jpn. J. Cancer Res., 2002, 93, 216 CrossRef CAS PubMed.
- X. Cao,
et al., Curcumin suppresses tumorigenesis by ferroptosis in breast cancer, PLoS One, 2022, 17, e261370 Search PubMed.
- X. Wang,
et al., Ultrasound induces cellular destruction of nasopharyngeal carcinoma cells in the presence of curcumin, Ultrasonics, 2011, 51, 165 CrossRef CAS PubMed.
- P. Wang, C. S. Xu, J. Xu, X. Wang and A. W. Leung, Hypocrellin B enhances ultrasound-induced cell death of nasopharyngeal carcinoma cells, Ultrasound Med. Biol., 2010, 36, 336 CrossRef PubMed.
- X. Wang,
et al., Hypocrellin B-mediated sonodynamic action induces apoptosis of hepatocellular carcinoma cells, Ultrasonics, 2012, 52, 543 CrossRef CAS PubMed.
- F. Bosca,
et al., Exploiting Lipid and Polymer Nanocarriers to Improve the Anticancer Sonodynamic Activity of Chlorophyll, Pharm., 2020, 12, 605 CAS.
- C. H. Kim,
et al., Self-immolative nanosensitizer for glutathione depletion- assisted sonodynamic therapy, Theranostics, 2022, 12, 7465 CrossRef CAS PubMed.
- S. Yamamoto, E. Yuba, A. Harada and K. Kono, Effective Condensation of Multivalent Anions into Polyion Complex Micelles Prepared from TiO2 Nanoparticles and Polyallylamine Bearing Poly(ethylene glycol) Grafts, Langmuir, 2015, 31, 8583 CrossRef CAS PubMed.
- X. Xu,
et al., TiO2 -based Surface-Enhanced Raman Scattering bio-probe for efficient circulating tumor cell detection on microfilter, Biosens. Bioelectron., 2022, 114305 CrossRef CAS PubMed.
- S. Iqbal,
et al., Mathematical modeling and experimental analysis of the efficacy of photodynamic therapy in conjunction with photo thermal therapy and PEG-coated Au-doped TiO2 nanostructures to target MCF-7 cancerous cells, Saudi J. Biol. Sci., 2021, 28, 1226 CrossRef CAS PubMed.
- X. Wang,
et al., Ultrafine Titanium Monoxide (TiO) Nanorods for Enhanced Sonodynamic Therapy, J. Am. Chem. Soc., 2020, 142, 6527 CrossRef CAS PubMed.
- C. Dai, S. Zhang, Z. Liu, R. Wu and Y. Chen, Two-Dimensional Graphene Augments Nanosonosensitized Sonocatalytic Tumor Eradication, ACS Nano, 2017, 11, 9467 CrossRef CAS PubMed.
- D. G. You,
et al., ROS-generating TiO2 nanoparticles for non-invasive sonodynamic therapy of cancer, Sci. Rep., 2016, 6, 23200 CrossRef CAS PubMed.
- V. G. Deepagan,
et al., Long-Circulating Au-TiO Nanocomposite as a Sonosensitizer for ROS-Mediated Eradication of Cancer, Nano Lett., 2016, 16, 6257 CrossRef CAS PubMed.
- A. P. Sviridov,
et al., Porous silicon nanoparticles as sensitizers for ultrasonic hyperthermia, Appl. Phys. Lett., 2013, 103, 2873 CrossRef.
- S. Alekseev, D. Korytko, M. Iazykov, S. A. Khainakov and V. Lysenko, Electrochemical Synthesis of Carbon Fluorooxide Nanoparticles from 3C-SiC Substrates, J. Phys. Chem. C, 2015, 119, 20503 CrossRef CAS.
- A. P. Sviridov,
et al., Cytotoxicity control of silicon nanoparticles by biopolymer coating and ultrasound irradiation for cancer theranostic applications, Nanotechnology, 2017, 28, 105102 CrossRef CAS PubMed.
- L. A. Osminkina,
et al., Porous silicon nanoparticles as efficient sensitizers for sonodynamic therapy of cancer, Microporous Mesoporous Mater., 2015, 169–175 CrossRef CAS.
- A. Ebrahimi Fard, A. Zarepour, A. Zarrabi, A. Shanei and H. Salehi, Synergistic effect of the combination of triethylene-glycol modified Fe3O4 nanoparticles and ultrasound wave on MCF-7 cells, J. Magn. Magn. Mater., 2015, 394, 44 CrossRef CAS.
- Q. Qian, J. Zhang, Z. Gu and Y. Chen, Nanocatalysts-augmented Fenton chemical reaction for nanocatalytic tumor therapy, Biomater., 2019, 211, 1 CrossRef PubMed.
- H. Xu,
et al., Biocompatible Fe-Hematoporphyrin coordination nanoplatforms with efficient sonodynamic-chemo effects on deep-seated tumors, Biomater., 2020, 257, 120239 CrossRef CAS PubMed.
- J. Chen,
et al., pH-responsive catalytic mesocrystals for chemodynamic therapy via ultrasound-assisted Fenton reaction, Chem. Eng. J, 2019, 369, 394 CrossRef CAS.
- S. Umemura, N. Yumita, R. Nishigaki and K. Umemura, Mechanism of cell damage by ultrasound in combination with hematoporphyrin, Jpn. J. Cancer Res., 1990, 81, 962 CrossRef CAS PubMed.
- C. Lin, C. Fan and C. Yeh, The Impact of Surface Drug Distribution on the Acoustic Behavior of DOX-Loaded Microbubbles, Pharm., 2021, 13, 2080 CAS.
- G. Dimcevski,
et al., A human clinical trial using ultrasound and microbubbles to enhance gemcitabine treatment of inoperable pancreatic cancer, J. Controlled Release, 2016, 243, 172 CrossRef CAS PubMed.
- P. Dwivedi,
et al., Magnetic Targeting and Ultrasound Activation of Liposome–Microbubble Conjugate for Enhanced Delivery of Anticancer Therapies, ACS Appl. Mater. Interfaces, 2020, 12, 23737 CrossRef CAS PubMed.
- S. Kuchler-Bopp, J. P. Delaunoy, J. C. Artault, M. Zaepfel and J. B. Dietrich, Astrocytes induce several blood–brain barrier properties in non-neural endothelial cells, NeuroReport, 1999, 10, 1347 CrossRef CAS PubMed.
- S. Kotopoulis, G. Dimcevski, O. H. Gilja, D. Hoem and M. Postema, Treatment of human pancreatic cancer using combined ultrasound, microbubbles, and gemcitabine: a clinical case study, Med. Phys., 2013, 40, 72902 CrossRef PubMed.
- C. Y. Dong,
et al., Neisseria meningitidis Opca Protein/MnO2 Hybrid Nanoparticles for Overcoming the Blood–Brain Barrier to Treat Glioblastoma, Adv. Mater., 2022, 34, 2109213 CrossRef CAS PubMed.
- H. J. Liu, M. Wang, S. Shi, X. Hu and P. Xu, A Therapeutic Sheep in Metastatic Wolf’s Clothing: Trojan Horse Approach for Cancer Brain Metastases Treatment, Nano-Micro Lett., 2022, 114 CrossRef PubMed.
- C. McEwan,
et al., Polymeric microbubbles as delivery vehicles for sensitizers in sonodynamic therapy, Langmuir, 2014, 30, 14926 CrossRef CAS PubMed.
- Y. Li,
et al., Cell penetrating peptide-modified nanoparticles for tumor targeted imaging and synergistic effect of sonodynamic/HIFU therapy, Int. J. Nanomed., 2019, 14, 5875 CrossRef CAS PubMed.
- R. Abdalkader,
et al., Evaluation of the potential of doxorubicin loaded microbubbles as a theranostic modality using a murine tumor model, Acta Biomater., 2015, 19, 112 CrossRef CAS PubMed.
- S. Kim,
et al., Drug-loaded titanium dioxide nanoparticle coated with tumor targeting polymer as a sonodynamic chemotherapeutic agent for anti-cancer therapy, Nanomed.: Nanotechnol. Biol. Med., 2020, 24, 102110 CrossRef CAS PubMed.
- X. Zhang, T. Zhu, Y. Miao, L. Zhou and W. Zhang, Dual-responsive doxorubicin-loaded nanomicelles for enhanced cancer therapy, J. Nanobiotechnology, 2020, 18, 136 CrossRef CAS PubMed.
- C. Li,
et al., Red blood cell membrane-enveloped O self-supplementing biomimetic nanoparticles for tumor imaging-guided enhanced sonodynamic therapy, Theranostics, 2020, 10, 867 CrossRef CAS PubMed.
- X. Pan,
et al., MOF-Derived Double-Layer Hollow Nanoparticles with Oxygen Generation Ability for Multimodal Imaging-Guided Sonodynamic Therapy, Angew. Chem. Int. Ed., 2020, 59, 13557 CrossRef CAS PubMed.
- J. Chen,
et al., Oxygen-Self-Produced Nanoplatform for Relieving Hypoxia and Breaking Resistance to Sonodynamic Treatment of Pancreatic Cancer, ACS Nano, 2017, 12849–12862 CrossRef CAS PubMed.
- X. Li, X. Geng, Z. Chen and Z. Yuan, Recent advances in glioma microenvironment-response nanoplatforms for phototherapy and sonotherapy, Pharmacol. Res., 2022, 179, 106218 CrossRef CAS PubMed.
- Y. Liu, P. Wang, Q. Liu and X. Wang, Sinoporphyrin sodium triggered sono-photodynamic effects on breast cancer both in vitro and in vivo, Ultrason. Sonochem., 2016, 31, 437 CrossRef CAS PubMed.
- N. Miyoshi,
et al., Combination of Sonodynamic and Photodynamic Therapy against Cancer Would Be Effective through Using a Regulated Size of Nanoparticles, Nanosci. Nanotechnol., 2016, 4, 1 CAS.
- Z. H. Jin,
et al., Combination effect of photodynamic and sonodynamic therapy on experimental skin squamous cell carcinoma in C3H/HeN mice, J. Dermatol., 2000, 27, 294 CrossRef CAS PubMed.
- X. Tian,
et al., New Insight into a Fenton-like Reaction Mechanism over Sulfidated ?-FeOOH: Key Role of Sulfidation in Efficient Iron(III) Reduction and Sulfate Radical Generation, Environ. Sci. Technol., 2022, 56, 5542–5551 CrossRef CAS PubMed.
- K. Zhang, X. Meng, Z. Yang, H. Dong and X. Zhang, Enhanced cancer therapy by hypoxia-responsive copper metal-organic frameworks nanosystem, Biomater., 2020, 258, 120278 CrossRef CAS PubMed.
- X. Lin,
et al., An Ultrasound Activated Vesicle of Janus Au-MnO Nanoparticles for Promoted Tumor Penetration and Sono-Chemodynamic Therapy of Orthotopic Liver Cancer, Angew. Chem. Int. Ed., 2020, 59, 1682 CrossRef CAS PubMed.
- F. L. Lizzi and M. Ostromogilsky, Analytical modelling of ultrasonically induced tissue heating, Ultrasound Med. Biol., 1987, 13, 607 CrossRef CAS PubMed.
- T. Kondo and E. Kano, Enhancement of hyperthermic cell killing by non-thermal effect of ultrasound, Int. J. Radiat. Biol., 1987, 51, 157 CAS.
- W. Yang, H. Xu, Q. Liu, C. Liu and R. Xie, 5-Aminolevulinic acid hydrochloride loaded microbubbles-mediated sonodynamic therapy in pancreatic cancer cells, Artificial Cells, 2020, 48, 1178 CAS.
- F. Gong,
et al., Preparation of TiH nanodots by liquid-phase exfoliation for enhanced sonodynamic cancer therapy, Nat. Commun., 2020, 11, 3712 CrossRef CAS PubMed.
- R. Hou,
et al., In situ conversion of rose bengal microbubbles into nanoparticles for ultrasound imaging guided sonodynamic therapy with enhanced antitumor efficacy, Biomater. Sci., 2020, 8, 2526–2536 RSC.
- L. H. Treat,
et al., Targeted delivery of doxorubicin to the rat brain at therapeutic levels using MRI-guided focused ultrasound, Int. J. Cancer, 2007, 121, 901 CrossRef CAS PubMed.
- L. Yang,
et al., A multifunctional nanoparticle system combines sonodynamic therapy and chemotherapy to treat hepatocellular carcinoma, Nano Res., 2017, 10, 834 CrossRef.
- Y. Ding,
et al., An enhanced chemotherapeutic effect facilitated by sonication of MSN, Dalton Trans., 2017, 46, 11875 RSC.
- J. Shi,
et al., Reactive Oxygen Species-Manipulated Drug Release from a Smart Envelope-Type Mesoporous Titanium Nanovehicle for Tumor Sonodynamic-Chemotherapy, ACS Appl. Mater. Interfaces, 2015, 7, 28554 CrossRef CAS PubMed.
- J. Luo,
et al., Enhancement of antitumor immunotherapy using mitochondria-targeted cancer cell membrane-biomimetic MOF-mediated sonodynamic therapy and checkpoint blockade immunotherapy, J. Nanobiotechnology, 2022, 20, 1 CrossRef PubMed.
- J. Luo,
et al., Enhancement of antitumor immunotherapy using mitochondria-targeted cancer cell membrane-biomimetic MOF-mediated sonodynamic therapy and checkpoint blockade immunotherapy, J. Nanobiotechnology, 2022, 20, 1 CrossRef PubMed.
- G. Jia,
et al., Cytotoxicity of carbon nanomaterials: single-wall nanotube, multi-wall nanotube, and fullerene, Environ. Sci. Technol., 2005, 39, 1378 CrossRef CAS PubMed.
- H. Nishimori,
et al., Silica nanoparticles as hepatotoxicants, Eur. J. Pharm. Biopharm., 2009, 72, 496 CrossRef CAS PubMed.
- D. Wang,
et al., Intraparticle Double‐Scattering‐Decoded Sonogenetics for Augmenting Immune Checkpoint Blockade and CAR‐T Therapy, Adv. Sci., 2022, 9, 2203106 CrossRef CAS PubMed.
Footnote |
† Authors contributed equally to this work and should be considered co-first authors. |
|
This journal is © The Royal Society of Chemistry 2024 |
Click here to see how this site uses Cookies. View our privacy policy here.