DOI:
10.1039/D4TB01706D
(Paper)
J. Mater. Chem. B, 2024,
12, 12232-12238
Development of a small molecule-based two-photon photosensitizer for targeting cancer cells†
Received
1st August 2024
, Accepted 21st October 2024
First published on 22nd October 2024
Abstract
Photodynamic therapy (PDT) employing two-photon (TP) excitation is increasingly recognized to induce cell damage selectively in targeted areas, underscoring the importance of developing TP photosensitizers (TP-PSs). In this study, we developed BSe-B, a novel PS that combines a selenium containing dye with biotin, a cancer-selective ligand, and is optimized for TP excitation. BSe-B demonstrated enhanced cancer selectivity, efficient generation of type-I based reactive oxygen species (ROS), low dark toxicity, and excellent cell-staining capability. Evaluation across diverse cell lines (HeLa, A549, OVCAR-3, WI-38, and L-929) demonstrated that BSe-B differentiated and targeted cancer cells while sparing normal cells. BSe-B displayed excellent in vivo biocompatibility. In cancer models such as three-dimensional spheroids and actual colon cancer tissues, BSe-B selectively induced ROS production and cell death under TP irradiation, demonstrating precise spatial control. These findings highlight the potential of BSe-B for imaging-guided PDT and its capability for micro treatment within tissues. Thus, BSe-B demonstrates robust TP-PDT capabilities, making it a promising dual-purpose tool for cancer diagnosis and treatment.
Introduction
Photodynamic therapy (PDT) for cancer stands out for its reduced side effects, noninvasiveness, and site-specific treatment capabilities, distinguishing it from traditional cancer therapies such as surgery and chemotherapy.1–4 PDT employs photosensitizers (PSs) activated by light, which interact with oxygen or biological substrates to generate cytotoxic singlet oxygen (1O2) or reactive oxygen species (ROS), thereby inducing cell damage and death.5–10 However, clinically approved PSs such as chlorine, porphyrin, and phthalocyanine are effective primarily for superficial diseases, such as skin cancer, due to their limited tissue penetration under ultraviolet-visible (UV-Vis) light (<700 nm).11,12 In addition, they have disadvantages, including limitations in intravenous administration owing to low solubility and lack of cancer-selective ability.13–15 To overcome these limitations, researchers are developing PSs with enhanced cancer-targeting capabilities. Cancer-selective ligands are gaining attention in diagnosis and treatment, playing a critical role in selectively delivering PSs to cancer cells.16,17 Cancer cells often overexpress various tumor-specific receptors, including certain vitamin receptors that promote rapid growth, proliferation, and cell survival.18–20 For example, receptors for biotin (vitamin B7) are notably overexpressed on the surface of cancer cells found in cervical cancer, lung cancer, breast cancer, and colon cancer.19,21–24 Several PSs have significantly improved cancer selectivity by utilizing biotin as a targeting ligand.25–27 Therefore, leveraging biotin as a targeting ligand to deliver PSs to cancer cells represents a promising PDT strategy toward enhancing treatment efficacy while minimizing off-target effects.
Furthermore, PDT can benefit significantly from advancements in imaging technology. Fluorescence imaging plays a crucial role in visualizing cellular and molecular processes within living organisms and offers real-time insights into various physiological and pathological conditions. However, most PDT agents lack imaging capabilities. Combining imaging ability with cancer-targeting PSs could revolutionize imaging-guided therapies, enabling precise treatment guidance and therapeutic outcome assessment. Furthermore, the integration of two-photon excitation (TPE) offers advantages over traditional UV-Vis excitation by employing longer-wavelength near-infrared (NIR) light, which penetrates more deep into tissues with reduced photodamage.28–32 The nonlinear characteristics of TPE confine PS activation to precise locations, minimizing collateral damage to healthy tissues and facilitating micro treatment within tissues.33–36 The development of PSs optimized for TPE conditions (TP-PSs) represents a promising frontier in PDT research, addressing current limitations and expanding the scope of PDT applications in clinical settings.
Small molecule-based dyes (such as fluorescent probes and PS) are easy to synthesize, allowing for various applications and modifications. They are also suitable for application to biological samples owing to their excellent staining ability on cells.37–39 We recently reported on a dimethylaminonaphthalene (DAN) scaffold featuring an electron push–pull system, which has been used to design various two-photon fluorescent probes.40 These dyes exhibit significant two-photon excitation, primarily due to their efficient internal charge transfer (ICT) characteristics. Herein, we performed a simple chemical modification of the DAN structure by incorporating selenium, resulting in BSe-B (Fig. 1a). BSe-B includes a cancer-targeting unit (biotin) known for its high tumor affinity and low molecular weight.41,42 We explored the cancer selectivity and therapeutic efficacy of BSe-B in various cancer models, including different cell lines and tissues. Although several two-photon photosensitizers are based on small molecules (Table S1 and Scheme S2, ESI†), there have been no reports of selenium-containing, small molecule-based two-photon excited photosensitizers that target cancer cells and generate reactive oxygen species through type-I process. Incorporating imaging capabilities into PDT agents, such as BSe-B, not only facilitates precise treatment guidance but also enables real-time monitoring of therapeutic responses. This integration represents a significant advancement, potentially improving PDT treatment outcomes while minimizing off-target effects.
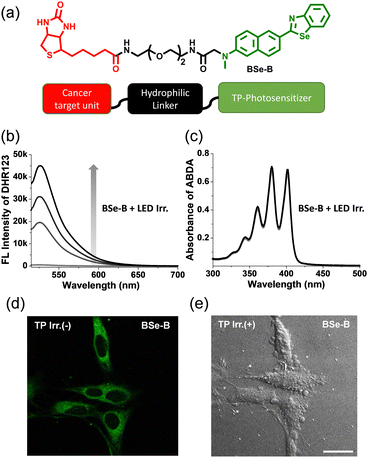 |
| Fig. 1 (a) Chemical structure of BSe-B; (b) fluorescence spectra of DHR123 and (c) absorbance spectra of ABDA containing BSe-B after white LED irradiation (0–30 min); (d) fluorescence and (e) bright field images of HeLa cells; cells were stained with BSe-B (2 μM). After probe staining, cells were irradiated with a two-photon (TP) laser at 730 nm. Excitation: 730 nm; emission: 450–600 nm. Scanning laser: 730 nm; 1.4 mW; 1.3 s per scan; 100 scans. Scale bar: 30 μM. | |
Results and discussion
Synthetic strategy and design of BSe-B
BSe-B is composed of BSe (TP-PS) conjugated to oligomeric ethylene glycol (a hydrophilic linker) and biotin (a cancer-targeting unit) (Fig. 1). We conjugated a hydrophilic linker in the middle to avoid affecting the ROS generation efficiency of BSe and the cancer selectivity of biotin. BSe-B can be easily modified to exploit the advantages of small-molecule dyes. First, a hydrophilic linker and biotin are conjugated to a 6-(methylamino)-2-naphthaldehyde structure using an amide-coupling reagent. Cyclization was then performed using bis(2-aminophenyl)diselenide and an acid catalyst. Consequently, we obtained BSe-B in a few simple steps (Scheme S1, ESI†). The BSe-B structure was confirmed using 1H-NMR, 13C-NMR, and high-resolution mass spectrometry. The detailed synthesis steps are provided in ESI.†
Photophysical characteristics and ROS generation ability of BSe-B
The photophysical characteristics of BSe-B were determined in each solvent (Fig. S1 and Table S2, ESI†). A maximum absorption wavelength of 370 nm and a maximum emission wavelength of 487 nm were observed. The absorption and emission spectra of BSe-B are red-shifted compared to the sulfur-containing BTDAN dye (Table S2, ESI†), which may be attributed to the enhanced internal charge transfer character. The fluorescence quantum yield (Φ) of BSe-B was calculated to be 46% in EtOH/phosphate-buffered saline 1
:
1 (10 mM, pH 7.4). The calculated TP action cross-section (δΦf) was roughly 50 GM at 740 nm (Fig. S2, ESI†). The intensity of the TP excited emission spectra exhibited a linear dependence on the square of the incident laser power, confirming nonlinear absorption (Fig. S2, ESI†). The significant δΦf value of BSe-B highlights its potential for effective imaging and therapy in deeper tissue layers.40 We demonstrated the ability of BSe-B to generate ROS but not singlet oxygen (1O2) in PBS buffer (Fig. 1b and c). Each indicator, DHR123 (ROS) and ABDA (1O2), was mixed with BSe-B and irradiated with white light. Consequently, the fluorescence intensity of DHR123, oxidized by the generated ROS, increased.43 However, since 1O2 was not produced, decomposition of the ABDA absorption spectrum was not observed.44 Under two-photon irradiation, the PL intensity of DHR123 enhanced significantly, whereas the absorbance of ABDA remains unchanged which demonstrating the type-I ROS production of BSe-B (Fig. S3, ESI†). ROS inhibition studies were conducted to confirm the specific ROS produced by BSe-B (Fig. S3, ESI†). The results showed that BSe-B primarily generates the O2˙− radical and partially produces NOO− under both white light and two-photon irradiation.
We confirmed the staining ability of BSe-B and the effect of TP-PDT on HeLa cells. As shown in Fig. 1d, BSe-B is efficiently taken up by HeLa cells, likely due to a specific ligand-receptor-mediated endocytosis process, and it does not form aggregates after being labeled for 30 minutes. Additionally, when the cells were irradiated with a TP laser, the cell-bleb phenomenon was observed, indicating that the cells were damaged (Fig. 1e).45BSe-B also has the advantage that diagnosis and treatment can be performed simultaneously because its fluorescence intensity allows cell imaging. In short, BSe-B, which displayed good ROS generation ability in cuvettes and cells and excellent cell-staining ability, has potential as a PDT tool.
ROS generation and PDT effect of BSe-B in cells
The dark toxicity and phototoxicity of BSe-B were evaluated using the CCK-8 assay (Fig. 2a). After incubating HeLa cells with BSe-B, the cells were divided into groups irradiated with light or kept in the dark. Cells exposed to light showed a marked decrease in viability, particularly evident at concentrations ≥0.2 μM, demonstrating BSe-B's high phototoxicity with minimal dark toxicity. We assessed the ability of BSe-B to induce cell damage via ROS generation after TP laser irradiation. ROS generation was evaluated using the dichlorodihydrofluorescein diacetate (DCHF-DA) assay, in which DCHF-DA, a non-fluorescent compound, enters cells and reacts with ROS to produce fluorescent dichlorofluorescein (DCF).46 Upon irradiation of HeLa cells with a TP laser in the presence of BSe-B and DCHF-DA, a significant increase in DCF fluorescence was observed (Fig. 2b), indicating effective ROS production by BSe-B.
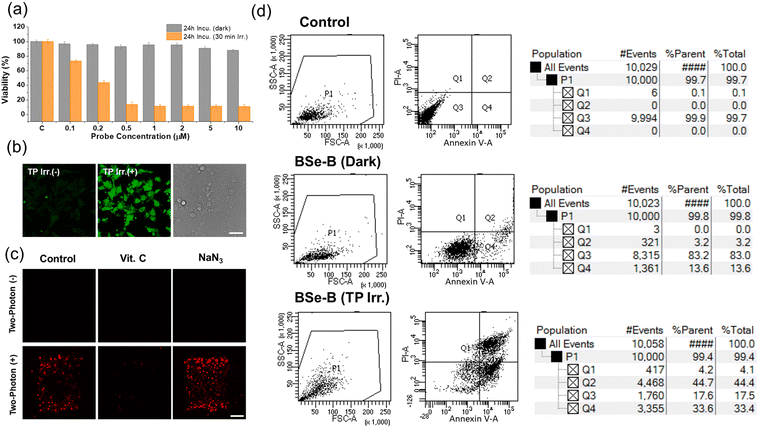 |
| Fig. 2 (a) Viability of HeLa cells after white LED irradiation or non-irradiation; the cells were treated with BSe-B. After irradiation, the cells were incubated for 24 h. The viability was measured using CCK-8. (b) Fluorescence images of HeLa cells co-stained with dichlorodihydrofluorescein diacetate (DCHF-DA) (10 μM) and BSe-B (2 μM); (c) fluorescence images of HeLa cells treated with BSe-B (2 μM), vitamin C (100 μM), or NaN3 (100 μM). Excitation: 488 nm (DCHF-DA, PI). Emission: 500–550 nm (DCHF-DA), 650–700 nm (PI). Scanning laser: 730 nm, 1.4 mW, 1.3 s per scan, 100 scans. Scale bars: (b) 40 μm and (c) 70 μm. (d) Cell viability of BSe-B using FACS analysis in HeLa cells; control (dimethylsulfoxide only), BSe-B (10 μM) staining followed by non-irradiation, and TP-irradiation at 730 nm after BSe-B (10 μM) staining. After all procedures, the cells were stained with Annexin V and PI (mean ± SD, n = 3). | |
Further investigation using ROS and 1O2 scavengers elucidated the mechanism of PDT induced by BSe-B (Fig. 2c). HeLa cells treated with BSe-B were divided into control, vitamin C (ROS scavenger, 100 μM), or NaN3 (1O2 scavenger, 100 μM) groups, followed by propidium iodide (PI) staining to assess cell death.47,48 In the absence of TP laser irradiation, PI fluorescence indicative of cell death was absent across all groups. Conversely, upon TP laser irradiation, PI fluorescence was observed in the control and NaN3 groups, whereas the vitamin C group showed no PI fluorescence. This suggests that during PDT, BSe-B predominantly operates via a type-I mechanism involving ROS generation. These findings underscore BSe-B's efficacy in ROS-mediated PDT and its potential as a targeted cancer therapy.
We conducted cell viability testing using fluorescence-activated cell sorting (Fig. 2d). HeLa cells were divided into a control group stained with dimethylsulfoxide alone or an experimental group stained with BSe-B (10 μM). These groups were further subdivided into non-irradiated and TP-irradiated groups. Apoptosis was assessed using Annexin V and PI staining.49–51 The control group showed high cell viability (over 99%), whereas the group stained with BSe-B and not irradiated with TP exhibited cell viability of more than 83%. In contrast, TP irradiation led to more than 77% of apoptosis in the BSe-B stained group. Additionally, to determine the IC50 of BSe-B under TP conditions, the live/dead cell ratio was calculated using Hoechst 33342 (live cell indicator) and PI (dead cell indicator).36,47,52 HeLa cells stained with BSe-B at concentrations in the range of 0.2–20 μM were irradiated with a TP laser, revealing an IC50 value of approximately 4.9 μM (Fig. S4, ESI†). These findings emphasized the potent apoptotic effect of BSe-B under TP irradiation, highlighting its potential as an effective photosensitizer in targeted cancer therapies.
Cancer cell selectivity experiment of BSe-B
The selectivity of BSe-B for cancer cells was evaluated using HeLa (biotin-positive cervical cancer) and WI-38 (biotin-negative normal lung) cells (Fig. 3). Following staining with BSe-B, real-time monitoring of the two-photon excitation fluorescence (TPEF) intensity was conducted for 60 min in HeLa and WI-38 cells. The fluorescence intensity of BSe-B was approximately ten times higher in HeLa cells (cancer cells) compared with WI-38 cells (normal cells) (Fig. 3a and b).
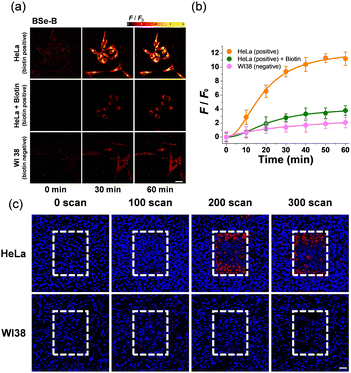 |
| Fig. 3 Biotin-conjugated BSe (BSe-B) targeting HeLa cells: (a) real-time TP images of HeLa (biotin-positive) and WI-38 (biotin-negative) cells; cells were treated with BSe-B (2 μM) with or without free biotin (1 mM). (b) Relative fluorescence intensities were acquired every 10 min. (c) Fluorescence images of HeLa and WI-38 cells; cells were treated with BSe-B (2 μM, 1 h incubation). After probe incubation, TP irradiation was performed within the white-dashed line box areas. Excitation: 488 nm (PI), 730 nm (Hoechst 33342, TP), and 750 nm (BSe-B, TP). Emission: 400–450 nm (Hoechst 33342), 480–520 nm (BSe-B), and 650–700 nm (PI). Scanning laser: 730 nm, 1.4 mW, 1.3 s per scan. Scale bar: (a) 40 μm, (c) 80 μm (mean ± SD, n = 3). | |
To confirm the specificity of BSe-B uptake by cancer cells mediated by the biotin unit, a competition experiment using free biotin was performed.27,53 Cells treated with free biotin exhibited a fluorescence intensity similar to that of WI-38 cells (Fig. 3a and b), indicating that the enhanced uptake of BSe-B in cancer cells could be attributed to the binding between the biotin unit and the overexpressed biotin receptor in the cancer cells.54 In addition, TP-PDT efficacy experiments were conducted on both cancer and normal cells. After incubating each cell type with BSe-B for 1 h, the cells were irradiated with TP according to the number of scans. In HeLa cells, cell death occurred in the TP-irradiated area, whereas no cell death was observed in the WI-38 cells (Fig. 3c). Similar TP-PDT experiments were performed on other cancer cell types, including A549 (lung cancer) and OVCAR-3 (ovarian cancer) cells, where cell death was observed after staining with BSe-B and TP irradiation. In contrast, TP irradiation of L-929 cells (normal connective tissue cells) resulted in the death of only a few cells (Fig. S5, ESI†). These results highlight BSe-B's potential for targeted cancer therapy by leveraging biotin-mediated selectivity and TP excitation for precise imaging and treatment.
Biocompatibility test of BSe-Bin vivo
A biocompatibility experiment was conducted to assess the in vivo safety of BSe-B (Fig. S6, ESI†). Following injection into mice, the organ morphology was examined using hematoxylin and eosin (H&E) staining (Fig. S6a, ESI†). The results indicated no significant morphological changes compared with the control group, confirming the absence of adverse effects in the organs tested. Biochemical blood analysis further confirmed the absence of toxicity, with no notable differences observed compared with the control group (Fig. S6b, ESI†). Overall, these findings demonstrate that BSe-B exhibits excellent biocompatibility, supporting its potential for safe use in vivo without compromising liver or kidney function.
TPM imaging in three-dimensional (3D)-spheroid tumor model and human colon tissue
In our major experimental observations, a 3D-spheroid tumor model was used and evaluated (Fig. 4). The fluorescence intensity of BSe-B in HeLa cell spheroids was 6 times higher than that of WI-38 cells (Fig. 4a and c). Upon TP irradiation, there was a significant increase in the PI signal, specifically in the HeLa spheroids (Fig. 4b and d), indicating BSe-B's efficacy in targeting and inducing cytotoxicity in HeLa cells.
 |
| Fig. 4 Selectivity and toxicity of BSe-B towards HeLa spheroids: (a) 3D-spheroid two-photon fluorescence images of HeLa (biotin positive) and WI-38 (biotin negative) cells. Spheroids were stained with BSe-B (10 μM) for 3 h. (b) One-photon fluorescence image of PI staining in the spheroid. Spheroids stained with BSe-B (10 μM, 3 h incubation) were irradiated with two-photon lasers at 730 nm and then stained with PI (10 μM) for 24 h. (c) Two-photon fluorescence intensity of BSe-B in each spheroid; (d) one-photon fluorescence intensity of PI in each spheroid; excitation: 488 nm (PI) and 730 nm (BSe-B, TP); emission: 480–520 nm (BSe-B) and 650–700 nm (PI); scanning laser: 730 nm, 1.4 mW, 1.3 s per scan. Scale bar: (a) 50 μm, (b) 100 μm (mean ± SD, n = 3), (***p < 0.001). | |
BSe-B was applied to biopsy samples obtained from patients with colorectal cancer at Ajou University Hospital (Fig. 5). Staining under identical conditions revealed that the TPEF intensity in cancerous tissues was approximately 8 times stronger than that in normal tissues (Fig. 5a and c). This selective uptake was evident not only in two-dimensional cell cultures and 3D spheroids but also in patient-derived colorectal cancer tissues. TP excitation allows the precise targeting of specific regions within tissues. Colorectal cancer tissues treated with BSe-B and DHR123 exhibited increased fluorescence (red) of DHR123 solely within the irradiated area marked by a white dotted box (Fig. 5b and d). In contrast, DHR123 fluorescence was not observed in adjacent normal tissues under the same conditions. These results underscore BSe-B's ability to selectively target and induce cytotoxic effects within distinct regions of colorectal cancer tissue ex vivo, highlighting its potential as a promising therapeutic strategy for future applications.
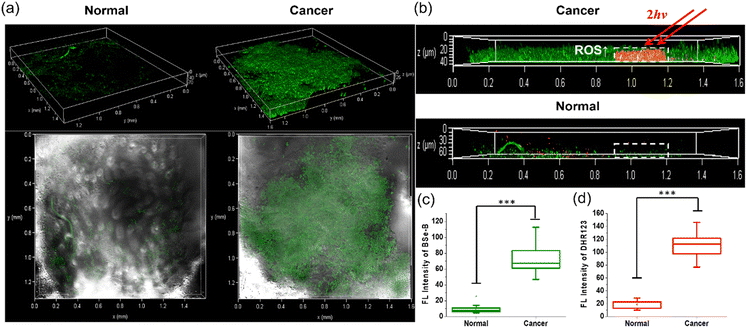 |
| Fig. 5 Selectivity and toxicity of BSe-B towards patient-derived colorectal cancer tissues: (a) two-photon 3D images of colon cancer and normal tissues. The tissues were treated with BSe-B (10 μM, 3 h incubation). (b) Z-section images of colon tissues after two-photon laser irradiation on the white-dashed line box region; the tissues were treated with BSe-B and DHR123. (c) Two-photon fluorescence intensity of BSe-B in colon cancer and normal tissues; (d) one-photon fluorescence intensity of DHR123 in colon cancer and normal tissues. Excitation: 488 nm (DHR123, OP), 730 nm (BSe-B, TP); emission: 480–520 nm (BSe-B), 500–550 nm (DHR123); scanning laser: 730 nm, 1.4 mW, 1.3 s per scan, 100 scans. The images shown in (b) are merges between BSe-B (TP, green) and DHR123 (OP, red) (mean ± SD, n = 3), (***p < 0.001). | |
Conclusions
We developed and synthesized BSe-B, a two-photon photodynamic therapy (TP-PDT) probe engineered for selective cancer targeting. By conjugating a biotin unit to BSe, known for its potent ROS generation, BSe-B was tailored to possess superior ROS generation capability, minimal dark toxicity, excellent cell-staining ability, and remarkable cancer selectivity. This biocompatible probe effectively differentiated cancer cells (HeLa, A549, and OVCAR-3) from normal cells (WI-38, L-929) across diverse cell lines, demonstrating significant therapeutic potential. BSe-Bin vivo revealed no significant morphological changes or biochemical abnormalities in the organs. Furthermore, BSe-B precisely discriminated between cancerous and normal tissues in 3D-spheroid models and live human colon tissue samples. Under TP laser irradiation, which offers spatial selectivity, BSe-B induces cell death and ROS generation deep within the tissues. This capability underscores BSe-B's potential as an advanced TP-PDT tool capable of integrating imaging-guided therapy, enabling precise treatment and facilitating the assessment of therapeutic outcomes.
Author contributions
Dong Joon Lee: investigation, writing – original draft; Vinayak Juvekar: investigation, writing – original draft; Yu Cao: investigation, data curation; Sauraj: investigation, data curation; Choong-Kyun Noh: investigation; Sung Jae Shin: conceptualization; Zhihong Liu: data curation, writing – review, and editing; Hwan Myung Kim: supervision; conceptualization; writing – review & editing.
Data availability
The data supporting this article have been included as part of the ESI.†
Conflicts of interest
There are no conflicts to declare.
Acknowledgements
This study was supported by grants from the National Leading Research Lab Program of the National Research Foundation of Korea (NRF), funded by the Korean government (MSIP) (NRF-2022R1A2B5B03001607), the Center for Convergence Research of Neurological Disorders (NRF-2019R1A5A2026045), and the Ajou University Research Fund.
References
- A. Kawczyk-Krupka, A. M. Bugaj, W. Latos, K. Zaremba, K. Wawrzyniec, M. Kucharzewski and A. Sieron, Photodiagn. Photodyn. Ther., 2016, 13, 158–174 Search PubMed.
- C. Hopper, Lancet Oncol., 2000, 1, 212–219 CrossRef CAS.
- N. P. Brodin, C. Guha and W. A. Tome, Technol. Cancer Res. Treat., 2015, 14, 355–368 CrossRef CAS PubMed.
- P. Agostinis, K. Berg, K. A. Cengel, T. H. Foster, A. W. Girotti, S. O. Gollnick, S. M. Hahn, M. R. Hamblin, A. Juzeniene, D. Kessel, M. Korbelik, J. Moan, P. Mroz, D. Nowis, J. Piette, B. C. Wilson and J. Golab, CA Cancer J. Clin., 2011, 61, 250–281 CrossRef PubMed.
- D. E. Dolmans, D. Fukumura and R. K. Jain, Nat. Rev. Cancer, 2003, 3, 380–387 Search PubMed.
- M. C. DeRosa and R. J. Crutchley, Coord. Chem. Rev., 2002, 233, 351–371 Search PubMed.
- P. Mroz, A. Yaroslavsky, G. B. Kharkwal and M. R. Hamblin, Cancers, 2011, 3, 2516–2539 Search PubMed.
- K. Plaetzer, T. Kiesslich, T. Verwanger and B. Krammer, Med. Laser Appl., 2003, 18, 7–19 Search PubMed.
- D. Van Straten, V. Mashayekhi, H. S. De Bruijn, S. Oliveira and D. J. Robinson, Cancers, 2017, 9, 19 CrossRef PubMed.
- Z. Zhou, J. Song, L. Nie and X. Chen, Chem. Soc. Rev., 2016, 45, 6597–6626 Search PubMed.
- H. Abrahamse and M. R. Hamblin, Biochem. J., 2016, 473, 347–364 Search PubMed.
- F. Hu, S. Xu and B. Liu, Adv. Mater., 2018, 30, e1801350 Search PubMed.
- M. R. Detty, S. L. Gibson and S. J. Wagner, J. Med. Chem., 2004, 47, 3897–3915 CrossRef CAS PubMed.
- J. Chen, T. Fan, Z. Xie, Q. Zeng, P. Xue, T. Zheng, Y. Chen, X. Luo and H. Zhang, Biomaterials, 2020, 237, 119827 CrossRef CAS.
- L. Bretin, A. Pinon, S. Bouramtane, C. Ouk, L. Richard, M. L. Perrin, A. Chaunavel, C. Carrion, F. Bregier, V. Sol, V. Chaleix, D. Y. Leger and B. Liagre, Cancers, 2019, 11, 1474 CrossRef CAS PubMed.
- L. Bildstein, C. Dubernet and P. Couvreur, Adv. Drug Delivery Rev., 2011, 63, 3–23 CrossRef CAS PubMed.
- Y. Singh, M. Palombo and P. J. Sinko, Curr. Med. Chem., 2008, 15, 1802–1826 CrossRef CAS PubMed.
- M. J. Akhtar, M. Ahamed, H. A. Alhadlaq, S. A. Alrokayan and S. Kumar, Clin. Chim. Acta, 2014, 436, 78–92 Search PubMed.
- G. Russell-Jones, K. McTavish, J. McEwan, J. Rice and D. Nowotnik, J. Inorg. Biochem., 2004, 98, 1625–1633 Search PubMed.
- S. Chen, X. Zhao, J. Chen, J. Chen, L. Kuznetsova, S. S. Wong and I. Ojima, Bioconjug. Chem., 2010, 21, 979–987 CrossRef CAS PubMed.
- A. D. Vadlapudi, R. K. Vadlapatla, D. Pal and A. K. Mitra, Int. J. Pharm., 2013, 441, 535–543 CrossRef CAS PubMed.
- K. Li, L. Qiu, Q. Liu, G. Lv, X. Zhao, S. Wang and J. Lin, J. Photochem. Photobiol., B, 2017, 174, 243–250 CrossRef CAS PubMed.
- Y. Singh, K. K. Durga Rao Viswanadham, A. Kumar Jajoriya, J. G. Meher, K. Raval, S. Jaiswal, J. Dewangan, H. K. Bora, S. K. Rath, J. Lal, D. P. Mishra and M. K. Chourasia, Mol. Pharm., 2017, 14, 2749–2765 CrossRef CAS PubMed.
- N. U. Deshpande and M. Jayakannan, Biomacromolecules, 2018, 19, 3572–3585 CrossRef CAS.
- M. F. Isaac-Lam and D. M. Hammonds, Pharmaceuticals, 2017, 10, 41 CrossRef.
- P. Balçik-Erçin, M. Çetin, M. Göksel and M. Durmuş, New J. Chem., 2020, 44, 3392–3401 RSC.
- D. J. Lee, V. Juvekar, H. W. Lee, E. S. Kim, C. K. Noh, S. J. Shin and H. M. Kim, Anal. Chem., 2021, 93, 16821–16827 CrossRef CAS.
- T. Dai, Y. Y. Huang and M. R. Hamblin, Photodiagn. Photodyn. Ther., 2009, 6, 170–188 Search PubMed.
- W. R. Zipfel, R. M. Williams and W. W. Webb, Nat. Biotechnol., 2003, 21, 1369–1377 CrossRef CAS.
- F. Helmchen and W. Denk, Nat. Methods, 2005, 2, 932–940 CrossRef CAS PubMed.
- R. M. Williams, W. R. Zipfel and W. W. Webb, Curr. Opin. Chem. Biol., 2001, 5, 603–608 CrossRef CAS PubMed.
- C. Xu, W. Zipfel, J. B. Shear, R. M. Williams and W. W. Webb, Proc. Natl. Acad. Sci. U. S. A., 1996, 93, 10763–10768 CrossRef CAS.
- M. Göppert-Mayer, Ann. Phys., 1931, 401, 273–294 Search PubMed.
- W. Kaiser and C. G. B. Garrett, Phys. Rev. Lett., 1961, 7, 229–231 CrossRef CAS.
- J. D. Bhawalkar, N. D. Kumar, C. F. Zhao and P. N. Prasad, J. Clin. Laser Med. Surg., 1997, 15, 201–204 CrossRef CAS.
- V. Juvekar, C. S. Lim, D. J. Lee, S. J. Park, G. O. Song, H. Kang and H. M. Kim, Chem. Sci., 2021, 12, 427–434 RSC.
- Y. X. Li, D. T. Xie, Y. X. Yang, Z. Chen, W. Y. Guo and W. C. Yang, Molecules, 2022, 27, 4501 CrossRef CAS.
- R. Tian, W. Sun, M. Li, S. Long, M. Li, J. Fan, L. Guo and X. Peng, Chem. Sci., 2019, 10, 10106–10112 RSC.
- M. Lan, S. Zhao, W. Liu, C. S. Lee, W. Zhang and P. Wang, Adv. Healthcare Mater., 2019, 8, e1900132 CrossRef PubMed.
- V. Juvekar, H. W. Lee, D. J. Lee and H. M. Kim, TrAC-Trends Anal. Chem., 2022, 157, 116787 CrossRef CAS.
- W. X. Ren, J. Y. Han, S. Uhm, Y. J. Jang, C. Kang, J. H. Kim and J. S. Kim, Chem. Commun., 2015, 51, 10403–10418 RSC.
- S. Maiti, N. Park, J. H. Han, H. M. Jeon, J. H. Lee, S. Bhuniya, C. Kang and J. S. Kim, J. Am. Chem. Soc., 2013, 135, 4567–4572 CrossRef CAS.
-
F. A. Villamena, Fluorescence Technique, in Reactive Species Detection in Biology, ed. F. A. Villamena, Elsevier, 2017, pp. 87–162 Search PubMed.
- T. Entradas, S. Waldron and M. Volk, J. Photochem. Photobiol., B, 2020, 204, 111787 CrossRef CAS PubMed.
- J. W. Mills, M. Horster and P. Wilson, Cell Biol. Int. Rep., 1986, 10, 11–17 Search PubMed.
- D. Yu, Y. Zha, Z. Zhong, Y. Ruan, Z. Li, L. Sun and S. Hou, Sens. Actuators, B, 2021, 339, 129878 CrossRef CAS.
- I. Nicoletti, G. Migliorati, M. C. Pagliacci, F. Grignani and C. Riccardi, J. Immunol. Methods, 1991, 139, 271–279 CrossRef CAS PubMed.
- S. Wang, W. Wu, P. Manghnani, S. Xu, Y. Wang, C. C. Goh, L. G. Ng and B. Liu, ACS Nano, 2019, 13, 3095–3105 CrossRef CAS.
- M. Van Engeland, L. J. Nieland, F. C. Ramaekers, B. Schutte and C. P. Reutelingsperger, Cytometry, 1998, 31, 1–9 CrossRef CAS.
- A. P. Demchenko, Cytotechnology, 2013, 65, 157–172 CrossRef CAS.
- A. M. Rieger, K. L. Nelson, J. D. Konowalchuk and D. R. Barreda, J. Vis. Exp., 2011, 50, e2597 Search PubMed.
- B. Chazotte, Cold Spring Harb. Protoc., 2011, pdbprot5557 CrossRef.
- M. Ren, Q. Xu, S. Wang, L. Liu and F. Kong, Chem. Commun., 2020, 56, 13351–13354 RSC.
- O. Tredan, C. M. Galmarini, K. Patel and I. F. Tannock, J. Nat. Cancer Inst., 2007, 99, 1441–1454 CrossRef CAS PubMed.
|
This journal is © The Royal Society of Chemistry 2024 |
Click here to see how this site uses Cookies. View our privacy policy here.