DOI:
10.1039/D4BM01257G
(Review Article)
Biomater. Sci., 2025,
13, 161-178
Nanotechnology at the crossroads of stem cell medicine
Received
21st September 2024
, Accepted 16th November 2024
First published on 18th November 2024
Abstract
Nanotechnology in stem cell medicine is an interdisciplinary field which has gained a lot of interest recently. This domain addresses key challenges associated with stem cell medicine such as cell isolation, targeted delivery, and tracking. Nanotechnology-based approaches, including magnetic cell sorting, fluorescent tagging, and drug or biomolecule conjugation for delivery, have enhanced precision in stem cell isolation and guided cell migration, increasing the therapeutic potential. Recent studies have focused on using nanomaterials and scaffolds to drive stem cell differentiation by activating specific molecular pathways, achieved through embedding biomolecules within the scaffold or through the scaffold's material composition and structure alone. These innovations hold promise in therapeutic applications across various diseases, including cancer stem cell targeting, neurodegenerative disorders, pre-eclampsia, cardiovascular conditions, and organoid development. This review examines recent advancements in the field, explores potential applications like biosensors and nanochips, and highlights the challenges and research gaps.
1. Introduction
Stem cells are a class of cells which are undifferentiated, have self-renewal capability and have the ability to differentiate into numerous types of cell lineages.1 They are vital in two major fields of science, developmental biology and regenerative medicine.2 These cells play a pivotal role in the early development,3 tissue repair,4 and maintenance throughout an organism's life.5 There are two primary types of stem cells: first being embryonic stem cells, which originate from early-stage embryos and can become any cell kind in the body, and adult stem cells, which are found in several tissues and usually generate cell types specific to their tissue of origin.6 The remarkable adaptability and regenerative potential of stem cells have made them a focal point of medical research, paving a path for innovative treatments for several diseases and injuries by the replacement of damaged or malfunctioning cells with healthy ones.5,7
Stem cells can be categorized into several types based on their origin and differentiation potential, each with unique characteristics and applications. Potency refers to the differentiation potential of stem cells.8 Totipotent stem cells, present in the earliest embryonic stages, can differentiate into all cell types, including embryonic and extraembryonic tissues (example: zygote). As development progresses, stem cells become more specialized: multipotent stem cells can differentiate into several cell types in a specific lineage (example: hematopoietic stem cells), oligopotent stem cells into a few closely related types (example: myeloid progenitor cells), and unipotent stem cells into a single cell type, with the ability of self-renewal (example: muscle stem cells). These categories reflect the decreasing potency and increasing specialization of stem cells as they develop.9,10
Nanotechnology is an interdisciplinary field that encompasses the manipulation of matter at the nanoscale, typically defined as dimensions ranging from 1 to 100 nanometres (nm). At this scale, materials display distinct physical and chemical properties compared to their bulk forms, primarily due to the enhanced surface area and quantum effects.11,12 There are various types of nanoparticles based on the composition like organic nanoparticles (proteins, lipids, polymers, and other organic compounds),13,14 carbon-based nanoparticles (solely made up of carbon atoms),15–17 inorganic nanoparticles18–24 (metals, semiconductors, etc.), etc. Based on the composition of the nanoparticle, each nanoparticle has certain mechanical, chemical and optical properties associated with it.25
Nanotechnology and stem cell biology have converged to create an exciting interdisciplinary frontier in regenerative medicine. At this intersection, nanoscale devices, particles and materials are being leveraged to precisely control and study stem cell behaviour.26 Nanoparticles like quantum dots,27–29 carbon nanotubes,30,31–33 and magnetic nanoparticles34 enable novel approaches for stem cell imaging,35 tracking, and delivery of genes/drugs.36–38 Engineered nanostructured surfaces and scaffolds can recapitulate the stem cell niche, providing topographical and mechanical cues that direct lineage commitment.12,30,39–41 By elucidating the molecular mechanisms underlying stem cell fate decisions, these nanotechnology tools are accelerating the development therapies based on stem cells for regenerating damaged tissues and treating diseases like heart disease, stroke, and neurodegenerative disorders.42,43
Recent research has advanced beyond merely differentiating stem cells into specific cell types, focusing also on methods for precise isolation and real-time monitoring through the use of biocompatible nanoparticles.44–46 These nanoparticles enable tracking of stem cell migration within the body and their interactions with tissue at the target site, providing insights into the stem cell fate at a molecular level.46,47,47,48,49–53 By studying these interactions, researchers can better understand cellular behaviour and mitigate off-target effects. Functionalizing nanoparticles or tagging them with specific ligands enhances targeting accuracy by promoting adhesion at the intended site.40,54 This approach allows quantification of the proportion of cells reaching the target and the duration required, thereby facilitating the optimization of dosing and dosing intervals for therapeutic efficacy. However, challenges remain in fully understanding the interactions between nanomaterials and stem cells, as well as translating these advances to the clinic. Nonetheless, the convergence of nanotechnology and stem cell biology holds great promise for realizing the therapeutic potential of stem cells as shown in Fig. 1.55,56
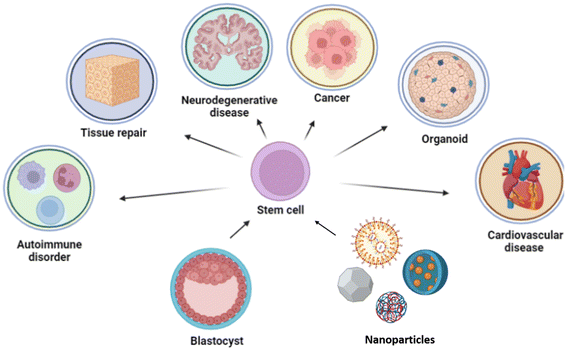 |
| Fig. 1 Therapeutic applications of blastocyst-derived stem cells modulated by nanoparticles for targeted differentiation into specific cell lineages. Nanoparticle-guided stem cells can regenerate healthy immune cells, offering potential treatments for autoimmune disorders. In tissue repair, these cells enhance regeneration by differentiating into the required tissue types. For neurodegenerative diseases, they can be directed to form neuronal cells, aiding in neuroregeneration. In cancer therapy, nanoparticle-modulated stem cells provide targeted treatment by generating specialized therapeutic cells. Additionally, this approach enhances the formation of organoids for disease modelling and drug testing. Lastly, in cardiovascular diseases, stem cells are directed to regenerate heart tissue by forming cardiomyocytes and endothelial cells. | |
In this review, we examine the synergistic potential of interdisciplinary approaches in advancing stem cell research, particularly through the use of nanoparticles, nanomaterials, and nanodevices. We focus on how recently these nanotechnologies have been applied in the isolation and differentiation of stem cells, and their significant contributions to therapeutic advancements in the treatment of various diseases, including cancer, neurodegenerative disorders, cardiovascular diseases, and preeclampsia, as well as in immunomodulation. By integrating nanotechnology with stem cell biology, we highlight the potential for developing more effective and targeted therapies across a broad spectrum of medical conditions and the gaps in the current research domain.
2. Isolation of stem cells
Stem cell isolation is a critical process in regenerative medicine and research, involving the extraction and purification of stem cells from different sources, including umbilical cord blood and adult tissues.57 The methods of isolation vary significantly depending on the types of stem embryos, cells being targeted. Nanoparticles are increasingly utilized in the isolation and manipulation of stem cells, enhancing the efficiency and specificity of these processes in regenerative medicine.58,59 Researchers have explored magnetic-activated cell sorting (MACS) which can efficiently and rapidly isolate single cells by leveraging the immunoreactivity of cell membrane antigens. This technique involves binding antibodies to magnetic particles, enabling cell separation through magnetic field manipulation as shown in Fig. 2.60 This technique leverages magnetic nanoparticles to isolate specific cell populations by recognizing surface antigens. The process initiates when superparamagnetic nanoparticles, coated with antibodies against specific cell surface markers, bind to the cells of interest. Once these magnetic beads attach to the target cells, the cell mixture is passed through a column placed in a strong magnetic field. The column's ferromagnetic matrix intensifies the magnetic field gradient, enabling the labeled cells to remain in the column while unlabeled cells are flushed out. Separation can occur through either positive selection, isolating target cells, or negative selection, removing non-target cells. When the column is removed from the magnetic field, the retained cells can be collected for further study or application.61–63
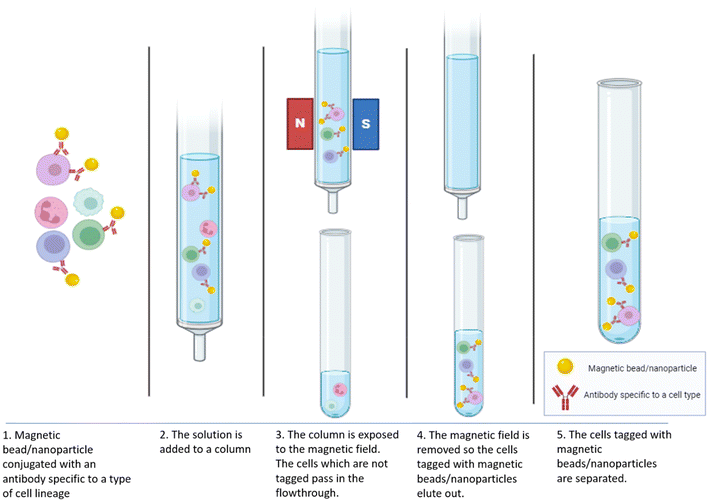 |
| Fig. 2 Magnetic-activated cell sorting (MACS). It is a method to isolate specific cells from a mixture using magnetic beads bound to antibodies. Labelled cells are captured in a magnetic column, while unlabelled cells pass through. The target cells are then released by removing the magnetic field, enabling precise and efficient cell separation. | |
A study explored the use of a high-specificity aptamer, Apt-W2, to isolate mouse bone marrow mesenchymal stem cells (mBMSCs) using magnetic beads (Apt-W2-MBs). The advantage of this method is that it ensures the isolated MSCs remain intact, retain their stem cell phenotype, and demonstrate high pluripotency, differentiating into osteoblasts, chondrocytes, and adipocytes. It has displayed a capture efficiency of approximately 88.33%.44 Furthermore, another study showed that conjugated MNPs with anti-CD34 antibodies effectively label and isolate peripheral blood progenitor cells from whole blood with 60–96% purity, 18–60% recovery, and a throughput of 1.7–9.3 × 104 cells per s.35 A group led by Gonçalo M. C. devised a 12-day protocol for efficiently differentiating human induced pluripotent stem cells (hiPSCs) into neural precursors (NPs) and removing Tra-1-60+ PSCs using MACS, resulting in a nearly pure NP population. They tried to achieve homogeneous NP populations by eliminating the residual pluripotent stem cells (PSCs) after differentiation. This method of isolating stem cells and NP cells derived from hiPSCs are crucial for drug screening, disease modeling, CNS development studies, and regenerative medicine.59
3. Tracking of stem cells
One of the major concerns associated with stem cells is the fact that the fate of the injected stem cells is yet not completely understood, like the time the cells will take to reach the target organ and the path of reaching to the target site. Hence, it is necessary to monitor the movement and path of stem cells in the body after administration. It will also help one understand what is the therapeutic efficacy and the risk associated as the cell passes through the body and hence help in upgrading the treatment approach as shown in Fig. 3. A group of researchers produced a magnetic particle imaging tracer of cubic iron oxide magnetic nanoparticles tailored with a shape and size of about 22 nm. They enabled real time and prolonged monitoring of stem cells with efficient cellular uptake and high sensitivity and resolution when transplanted in hindlimbs of ischemic mice.64 Another group aimed to track MSC-derived extracellular vesicles (MSC-EVs), which have great regeneration capacity in ischemic acute kidney injury by tagging EVs with DPA-SCP. They observed that MSC-EVs gathered in the injured kidney and were taken up by the renal tubular cells. These EVs also boosted mitochondrial defence and energy production by activating the Keap1-Nrf-2 pathway, protecting the cells from oxidative damage. Additionally, they triggered a rise in microRNA-200a-3p levels, aiding in recovery. This tracking method offered superior accuracy, spatiotemporal resolution and non-invasive visualization over commercially available options.65
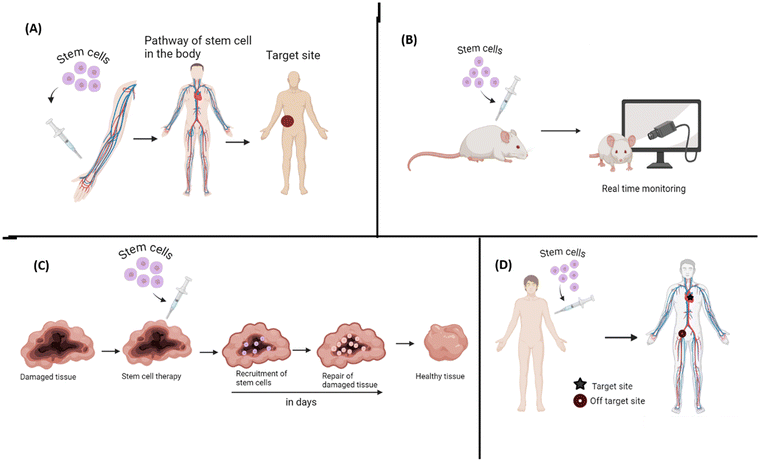 |
| Fig. 3 Tracking stem cells enables precise monitoring and analysis. (A) Studying the stem cell behaviour and migration pathways after administration, helping to assess whether the cells reach target sites. (B) Real-time monitoring of stem cell treatment, allowing for dosage optimization based on observed cell activity and therapeutic response. (C) Tracking at the target site to understand the timing, behaviour, and persistence of stem cells at the intended location, with follow-up assessments for sustained efficacy. (D) Detection of off-target sites where stem cells may migrate unintentionally, providing essential data to minimize and address undesired effects. | |
It is also important to understand stem cell-based therapies, such as the migration and biodistribution of human mesenchymal stem cells (hMSCs) in cancer treatments. Gao et al. proposed nanoparticle-based contrast agents – particularly promising due to their customizable imaging capabilities, yet most are still under development with challenges in retention time and safety, limiting access for researchers. They explored the application of FDA-approved core–shell fluorescent silica nanoparticles (C dots) for stem cell tracking. Their findings showed that 500 nm C dots offer extended cellular retention (up to one month), limited transfer between cells (lasting three weeks in co-culture systems), and minimal impact on hMSC properties, including viability, proliferation, differentiation, and tumor-targeting ability, making them promising tools for clinical research.
Since a lot of nanoparticles which are used in stem cell tracking are magnetic in nature, a study was conducted to check the biosafety of these molecules by using different forms of iron oxides (Fe2O3, Fe3O4, and CoxNi1−x Fe2O4 NPs), which were further compared by coating them with starch (ST-coated). They labelled adipose derived stem cells (ASCs) and found that CoxNi1−x Fe2O4 NPs significantly reduced cell proliferation. Interestingly, they found that starch-coated nanoparticles showed migration of cells as well as higher angiogenic potential in comparison with uncoated NPs. Hence, they concluded that ST-Fe2O3 NPs can provide effective labelling of cells without affecting the tracked cells with enhanced regeneration capacity.66
4. Nanoparticle-mediated gene delivery in stem cells
Gene delivery involves introducing therapeutic genes into cells to modify or correct genetic information, which can be critical in treating a wide range of diseases, including cancer, genetic disorders, and degenerative diseases. This process enables cells to produce specific proteins or induce pathways that can repair, replace, or inhibit dysfunctional genes, providing targeted and sustained therapeutic effects. However, efficient gene delivery remains challenging due to the need for high specificity, cellular uptake, and minimal toxicity. In this study, mesenchymal stem cells (MSCs) hold promise as vehicles in cell-based gene therapy due to their tumor-tropic properties and migration abilities, offering targeted delivery and immune protection of therapeutic agents. However, efficient gene transfer to MSCs is limited by cytotoxicity and low transfection efficiency. This study introduces a novel polyethylenimine (PEI25)-based delivery system designed to transfect MSCs with TRAIL-encoding plasmids, where TRAIL selectively induces apoptosis in cancer cells. Results showed that a heterocyclic amine-modified PEI25 enhanced MSC viability, transfection efficiency, and TRAIL expression. Engineered MSCs effectively migrated to and induced cell death in melanoma (B16F0) cells in vitro and, following single administration, delayed tumor growth and reduced tumor mass in vivo. Histological analysis confirmed extensive necrosis within treated tumors, suggesting modified PEI25/TRAIL–MSC complexes as a promising approach for targeted cancer therapy.67 Another project focused on cancer cell death through a targeted delivery system combining Bcl-xL-specific shRNA and a minimal dose of DOX to activate the intrinsic apoptotic pathway in gastric cancer cells. A modified branched PEI (10 kDa), linked to carboxylated single-walled carbon nanotubes (SWCNTs) via PEG, served as the shRNA carrier. To enhance targeting, an AS1411 aptamer was conjugated to the SWCNT–PEG–PEI complex, specifically directing it to nucleolin-overexpressing tumor cells. The final delivery vehicle, loaded with DOX and pBcl-xL shRNA, significantly inhibited growth in nucleolin-rich gastric cancer cells, as confirmed through cell viability and transfection assays. This combination of shRNA and a low DOX dose (about 58 times lower than IC50) demonstrated potent tumoricidal effects with reduced toxicity, suggesting a promising and safe approach for cancer therapy.68
In the case of bone repair, non-viral gene therapy has shown potential, but faces challenges with low transfection efficiency and uncontrolled expression, limiting clinical applications. This study introduces a new gene vector created by layering polyethyleneimine (PEI) with exosomes (Exos) derived from mesenchymal stem cells (MSCs) to enhance large plasmid delivery. A hypoxia-responsive plasmid was designed with an RTP801 promoter and miR-877 sponge to target expressions under low-oxygen conditions. Findings showed that this Exos-based system provides high transfection efficiency and low toxicity in MSCs, while also boosting BMP2 expression and neoangiogenesis under hypoxia, offering a promising strategy to advance bone tissue engineering.69 Hematopoietic stem cell (HSC) gene editing faces logistical challenges due to its reliance on ex vivo electroporation. This study assessed polymer-based nanoparticles (NPs) for delivering mRNA and nucleases to GCSF-mobilized human CD34+ cells, with potential for in vivo applications. NP-mediated delivery showed no toxicity, and the transfection efficiency correlated with the NP charge. Compared to electroporation, NP delivery achieved similar gene-editing efficiency with a 3-fold reduction in reagent use and improved HSC engraftment in NSG mouse models. Additionally, lyophilized mRNA- and nuclease-loaded NPs maintained transfection potential post-rehydration, highlighting a promising and portable platform for HSC gene therapy.70
5. Differentiation of stem cells
There are various types of nanoparticles and materials which have been used to differentiate stem cells into a desired type of cell in a lineage as shown in Fig. 4. This phenomenon of differentiation of stem cells has been explored in regenerative therapies which aim to repair damaged tissues or organs, including bone injuries, which are complex to treat.9
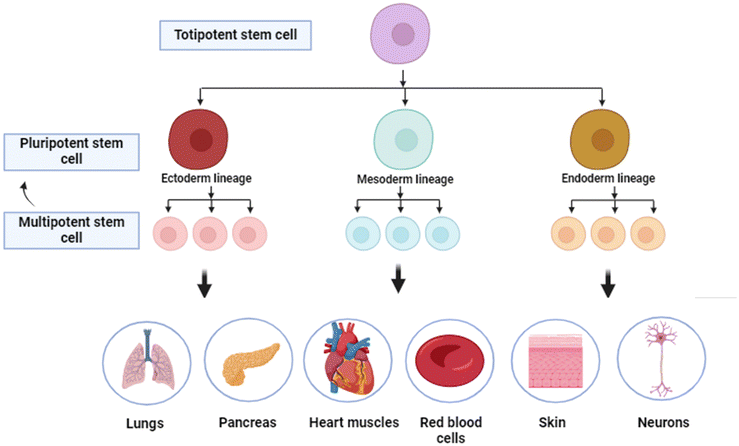 |
| Fig. 4 Different types of stem cells and their lineages. Stem cells are classified by their differentiation potential. Totipotent stem cells can develop into any cell type, including embryonic and extra-embryonic tissues. Pluripotent stem cells can become almost any cell type within the body. Multipotent stem cells are restricted to specific lineages, like blood cells. These cells then differentiate into various types of cells based on the external and internal signals. | |
5.1 Heart
A study addressing heart failure treatment investigated the effect of silver nanoparticles (Ag-NPs) on the process through which bone marrow-derived MSCs (BM-MSCs) develop into cardiomyocytes and they found the role of elongated telomeres and altered expression of key cardiac markers and proteins. Notably, Ag-NPs enhanced the Wnt3/β-catenin signaling pathway, suggesting a promising nanotechnology-based approach.50
5.2 Bone
There has been research exploring nanoparticles conjugated with various molecules which act as inducers for stem cell differentiation or bone cell differentiation. Curcumin nanocrystals have been investigated for the biocompatibility and osteogenic potential in human dental pulp stem cells (DPSCs). These nanocrystals were about 128 nm in size and spherical morphology and showed minimal toxicity at most concentrations, with significant inhibition of cell growth observed at 2.5–25 μM after 7 days. Alkaline phosphatase (ALP) activity increased significantly after two weeks, suggesting enhanced osteogenic differentiation compared to the controls (p < 0.05).47
There are various studies exploring various types of bionanomaterials or scaffolds for bone regeneration. A study by Ishmukhametov et al. used a bionanomaterial created by magnetic iron oxide nanoparticles (MNPs) and DNA and examined the process of differentiating hTERT-transduced adipose-derived MSCs into osteoblasts, adipocytes and chondrocytes using substrates with nanotopography. Citrate-stabilized MNPs that were approximately 10 nm in size were synthesized, and were used to form MNPs@DNA coatings which promoted chondrogenesis and osteogenesis, with cells maintaining a normal morphology and actin filament distribution. Higher concentrations of MNPs increased surface roughness and decreased cell adhesion. Notably, MNPs@DNA-coated glass substrates induced active chondrogenesis in a diluted differentiation medium, indicating the potential of these nanostructured coatings to facilitate cell differentiation with reduced growth factor levels.52 Another study explored the use of a polycaprolactone/gelatin/hydroxyapatite (PGH) scaffold which exhibited bead-free uniform fibres with a diameter of 616 nm, and porosity of 86%. Human exfoliated deciduous teeth-derived stem cells were cultured on the scaffolds. In vivo studies in Wistar rats demonstrated that the stem cell-seeded PGH scaffolds significantly enhanced cranial bone defect regeneration, indicating the scaffold's biocompatibility and efficacy in promoting stem cell adhesion, proliferation, and differentiation.71 In another study, they developed a composite piezoelectric fibrous membrane using titanium dioxide nanoparticles (TiO2) and polyvinylidene fluoride (PVDF), termed TiO2 @PVDF, to enhance cellular osteogenic differentiation. Comparing pure PVDF and control groups to the TiO2 @PVDF membrane, significantly upregulated osteocalcin (OCN) gene expressions by 49-fold and 6-fold, respectively, were observed. Increased surface potential of the membrane enhanced early cell attachment and proliferation by delivering electromechanical stimulation throughout osteogenic differentiation. Furthermore, the membrane enhanced alkaline phosphatase (ALP) activity up to 2-fold by utilizing electrical and mechanical signals from piezoelectric fibers, effectively triggering osteogenic differentiation. This study proposed a mechanism where surface potential of TiO2 @PVDF composite membranes regulates osteogenic behavior, offering insights to promote bone regeneration and remodeling using designing electroactive materials.51
5.3 Liver
Research on hepatoprotective effects has gained importance due to the rising incidence of liver diseases, which are often challenging to treat with conventional therapies. Understanding and developing effective hepatoprotective agents is essential for preventing liver damage, improving patient outcomes, and ultimately reducing the global burden of hepatic disorders.72,73 In this context, recent studies have explored the impact of silica magnetic graphene oxide (SMGO) on enhancing the effects of MSC-conditioned medium (MSC-CM) in protecting hepatocytes and promoting liver regeneration. Rats were treated with CCl4 causing acute liver failure (ALF), and were then introduced to the MSC-CM, SMGO, or a combination of both. Four days post-injection, histological and biochemical analyses revealed that SMGO significantly enhanced the therapeutic effects of the MSC-CM. The combined treatment reduced necrosis, inflammation, and liver enzyme levels (AST, ALT and ALP) while upregulating vascular endothelial growth factor (VEGF) and matrix metalloproteinase-9 (MMP-9) expression, indicating improved liver regeneration.34
5.4 Brain
A study explored the use of engineered nanomaterials, particularly vertically aligned nanostructured (VA-NS) arrays, to create specialized microenvironments that allow precise control and study of human neural stem cells. These arrays can influence cell fate, improve cell reprogramming, and serve as sensitive platforms for monitoring neural activity in vivo.42 Another study produced a novel nanostructure which is a bioresorbable elastomeric scaffold made from poly(L-lactide-co-ε-caprolactone) (PLCL) with ordered nanopatterns and graphene oxide (GO) and polydopamine surface functionalization. These scaffolds facilitated the attachment, alignment, and differentiation of murine neural stem cells (NSCs) into neurons and glial cells without additional extracellular matrix proteins. The nanostructured topography guided NSC migration and promoted neuronal and glial cell growth, indicating their potential for nerve tissue regeneration and cellular therapy.39
Another study investigated the biocompatibility of chemical vapor deposited (CVD) nanocrystalline diamond (NCD), particularly when doped with boron and nanostructured using an innovative approach that involves growing NCD over carbon nanotubes (CNTs) to create a 3D scaffold. This nanostructuring increases the material's capacitance, making boron-doped NCD (BNCD) ideal for neural interfaces. The biocompatibility was evaluated by examining how human neural stem cells (hNSCs) interacted with different NCD substrates, including both undoped and boron-doped, in planar and nanostructured forms. The results showed that boron doping did not negatively impact hNSC attachment, proliferation, or viability, and that nanostructuring further enhanced BNCD's biocompatibility, likely due to the increased surface area. These findings suggest that diamond, particularly when doped with boron, is a highly biocompatible material that can support cell growth and has potential for direct electrical interfacing in neural applications.30
5.5 Retina
Vision loss is caused by retinal diseases, such as age-related macular degeneration and diabetic retinopathy. Traditional treatments often fail to restore lost sight, making new approaches essential. Stem cells have the ability to regenerate damaged retinal tissue, while nanotechnology enhances their delivery and effectiveness by ensuring targeted, controlled release.74 The role of self-assembling peptide nanofiber scaffolds (SAPNs) in triggering the growth, proliferation, and retinal neuronal differentiation of progenitor cells derived from the ciliary pigment epithelium (CPE) of human cadaveric eyes was investigated by a group of researchers. CPE cells were encapsulated within the scaffolds, leading to the formation of proliferative clusters that expressed neural progenitor markers, including β-III tubulin, Pax6, Nestin, and Musashi1. Upon differentiation, the SCs displayed retinal neuronal markers such as rhodopsin, s-Opsin, and Recoverin. These findings underscore the potential of combining stem cells with nanotechnology to create advanced therapies for retinal regeneration and vision restoration (Table 1).49
Table 1 Various nanoparticles and nanomaterials used for differentiation of stem cells into different cell types
Sl. No. |
Type of nanoparticle/nanomaterial |
Type of stem cell used |
Differentiated cell type |
Reference |
1 |
Silver nanoparticles (Ag-NPs) |
Bone marrow-derived MSCs (BM-MSCs) |
Cardiomyocytes |
50
|
2 |
Curcumin nanocrystals |
Human dental pulp stem cells (DPSCs) |
Osteogenic differentiation |
47
|
3 |
Magnetic iron oxide nanoparticles (MNPs) and DNA |
hTERT-transduced adipose-derived MSCs |
Osteoblasts, adipocytes and chondrocytes |
52
|
4 |
Titanium dioxide nanoparticles (TiO2) and polyvinylidene fluoride (PVDF) membrane |
Mesenchymal stem cells |
Osteogenic differentiation |
51
|
5 |
Silica magnetic graphene oxide (SMGO) |
Hepatocytes in mesenchymal stem cell conditioned media |
Hepatocyte regeneration |
34
|
6 |
Vertically aligned nanostructured (VA-NS) arrays |
Human neuronal stem cells |
Neurons |
42
|
7 |
Poly(L-lactide-co-ε-caprolactone) (PLCL) with ordered nanopatterns and graphene oxide (GO) and polydopamine scaffolds |
Murine neural stem cells (NSCs) |
Neurons and glial cells |
39
|
8 |
Chemical vapor deposited (CVD) nanocrystalline diamond (NCD) doped with boron over carbon nanotubes (3D scaffold) |
Human neural stem cells (hNSCs) |
Neurons |
30
|
9 |
Self-assembling peptide nanofiber scaffolds (SAPNs) |
Retinal neuronal differentiation of progenitor cells |
Retinal cells |
49
|
10 |
Gold nanoparticles (AuNPs) |
Periodontal ligament stem cell (PDLSC) sheets |
Osteogenic differentiation |
75
|
6. Cancer stem cell therapy (drug delivery)
Cancer stem cells (CSCs) are subpopulations within tumors that possess the ability to self-renew, differentiate, and drive tumor progression, often causing drug resistance, recurrence, and metastasis.45,76
6.1 Oral cancer
This study examines the apoptotic effects of quinacrine-gold hybrid nanoparticles (QAuNP) on oral squamous cell carcinoma (OSCC) CSCs, both in vitro and in vivo, with near infrared (NIR) irradiation. The QAuNP and NIR treatment induced DNA damage and apoptosis in SCC-9-CSCs by disrupting mitochondrial membrane potential and increasing ROS. It upregulated CASPASE-3 and DR-5/DR-4 (critical executioner protease in the extrinsic apoptosis pathway) while reducing HSP-70. In vivo, this treatment increased DR-5 and CASPASE-3 expressions and decreased HSP-70, Ki-67 and CD-44 levels in xenograft mice, suggesting that QAuNP + NIR promotes apoptosis by modulating HSP-70 in OSCC-CSCs.53
6.2 Brain
A study explored the use of nanostructured lipid carriers (NLCs) as a promising agent for delivering drugs specifically to glioma cells. It investigated the mechanisms and therapeutic effects of doxorubicin (DOX) and paclitaxel (PTX) loaded NLCs (PTX-DOX-NLC) on glioma stem cells (GSCs). In a mouse xenograft glioma model, PTX-DOX-NLC showed superior inhibition of GSC proliferation and promotion of apoptosis compared to other treatments. Mechanistic studies revealed that PTX-DOX-NLC suppressed the PI3K/AKT/mTOR signaling pathway, highlighting its potential as a therapeutic option against glioma.77 Another study presents a method to culture glioblastoma (GBM) patient tissue (1 mm in diameter) in a microchannel network chip, preserving key tumor characteristics through vasculature-mimetic perfusion. Administration of temozolomide and radiation within 1 day on the chip mirrored clinical outcomes, bypassing the lengthy processes of cell and organoid culture, was carried out. Prolonged culture led to a loss of GBM signatures and increased migration of stem cells, indicating a “leaving-home” effect. Nanovesicles produced from GBM stem cells, loaded with an anti-PTPRZ1 peptide and temozolomide, reactivated quiescent cancer stem cells, leading to their death. This study proposes a GBM mimicking platform and mechanism-based nanotherapy for clinical translation.78
6.3 Blood
Acute myeloid leukemia (AML) is a hematologic malignancy distinguished by the uncontrolled proliferation of abnormal myeloid blasts within the bone marrow, which impairs normal blood cell production and leads to various clinical complications.79 It has high relapse and drug resistance because of leukemic stem cells (LSCs). Targeting LSCs, specifically marked by CD123, is crucial for effective treatment. Curcumin, a natural compound with low toxicity, inhibits leukemic cell growth but suffers from poor water solubility and bioavailability. This study formulated curcumin nanoparticles (Cur-NPs) and conjugated them with anti-CD123 antibodies to enhance solubility and target LSCs. The cytotoxicity of Cur-NPs and anti-CD123-Cur-NPs was evaluated in KG-1a cells, showing IC50 values of 74.20 ± 6.71 μM and 41.45 ± 5.49 μM, respectively. In conclusion, anti-CD123-Cur-NPs triggered higher apoptosis and showed greater uptake in KG-1a cells. These findings suggest that anti-CD123-Cur-NPs improve curcumin's targeting of LSCs and bioavailability, offering a promising therapeutic strategy for AML.80
6.4 Colon cancer
Exosomes, natural nanovesicles, are increasingly recognized as biocompatible carriers for drug delivery, enhancing drug effectiveness and safety.81 In this study, exosomes were isolated from adipose-derived stem cells (ADSCs) and used to encapsulate the hydrophobic drug SN38 via a combination of incubation, freeze–thaw, and surfactant methods (SN38/Exo). The exosomes were further conjugated with an anti-MUC1 aptamer (SN38/Exo-Apt) to target Mucin 1-overexpressing cancer cells. The novel method achieved a 58% encapsulation efficiency of SN38. In vitro, SN38/Exo-Apt demonstrated high cellular uptake and significant cytotoxicity against Mucin 1-overexpressing C26 cancer cells, compared to normal CHO cells. This approach offers a promising platform for targeted colorectal cancer therapy.54
6.5 Breast cancer
One of the approaches to address stem cells in cancer is via photodynamic therapy (PDT), a non-invasive treatment using light activation of photosensitizers, and it shows potential for targeting drug-resistant CSCs. However, PDT alone is often insufficient in eliminating CSCs effectively, necessitating targeted strategies. Gold nanoparticle bioconjugates combined with PDT offer a promising approach for specialised therapy by enhancing drug delivery, selectively binding to tumor cells/CSCs, and effectively generating reactive oxygen species (ROS).82,83 A smart theranostic platform was developed using MSC membrane (MSCM)-coated doxorubicin-loaded hollow gold nanoparticles functionalized with a MUC1 aptamer for targeted delivery. The nanoparticles exhibited a spherical morphology with a diameter of 118 nm. Doxorubicin was efficiently loaded into the hollow gold nanoparticles. In vitro release studies demonstrated a pH-responsive behavior, with 50% release of doxorubicin at pH 5.5 over 48 hours, compared to 14% at pH 7.4. Cytotoxicity experiments on MUC1-positive 4T1 cells showed significantly enhanced cell mortality at 0.468 and 0.23 μg ml−1 equivalent DOX concentrations compared to non-targeted formulations, while no cytotoxicity was observed in MUC1-negative CHO cells. In vivo studies revealed high tumor growth of the targeted formulation, leading to effectiveness of curbing tumor growth in 4T1 tumor-bearing mice. Additionally, the hollow gold nanoparticles enabled CT scan imaging of tumor tissue for up to 24 hours after administration. These discoveries highlight the potential of this theranostic system as a promising and safe approach for combating metastatic breast cancer (Fig. 5).84
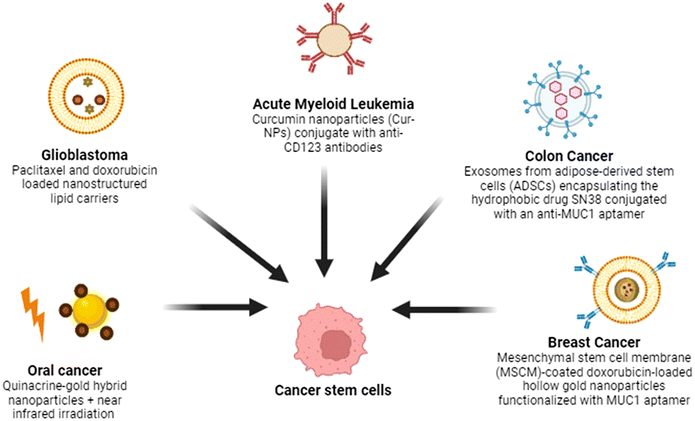 |
| Fig. 5 Different approaches to target cancer stem cells. Quinacrine conjugated gold nanoparticles were used along with near infrared irradiation for oral cancer stem cells, Paclitaxel and doxorubicin loaded lipid carriers were used for glioblastoma cancer stem cells; anti CD123 antibody conjugated curcumin nanoparticles were used to efficiently target stem cells in acute myeloid leukaemia. In the case of stem cells of colon cancer, exosomes were derived from adipose tissue and were conjugated to an anti MUC-1 aptamer and the encapsulated SN38 drug. In the case of breast cancer stem cells, doxorubicin loaded hollow gold nanoparticle were encapsulated in the MSC membrane and the membrane was further functionalised with a MUC-1 aptamer for better targeting. | |
One of the after effects associated with cancer is that, the male survivors of childhood cancer often suffer from azoospermia, highlighting the need for effective spermatogonial stem cell (SSC) isolation and purification. A study evaluated the efficacy of nanocomposite scaffolds made from chitosan, alginate and graphene oxide (GO) in promoting SSC proliferation. Cytotoxicity assay revealed that the scaffold with 30 μg mL−1 GO (ALGCS/GO30) had the best performance. Proliferation of SSCs showed that ALGCS/GO30 significantly enhanced SSC proliferation compared to the control. Immunocytochemistry confirmed increased PLZF protein expression in ALGCS/GO30 scaffolds, which is a transcription factor involved in cell proliferation differentiation and development. The ALGCS/GO30 scaffold shows potential for SSC proliferation in vitro.41
7. Neurodegenerative diseases
Neurodegenerative diseases are those diseases in which there is loss of neurons due to various causes like aging and genetic and environmental reasons.85 A study used adult stem cell therapies for regeneration of nerves at injury sites for therapeutic efficacy through magnetic targeted therapy in a Wallerian degeneration model. Adipose-derived MSCs (AdMSCs) loaded with citric acid-coated superparamagnetic iron oxide nanoparticles (SPIONs) were systemically transplanted and magnetically directed to the damaged nerve. Magnetic targeting significantly increased the AdMSC influx at the injury site, surpassing the efficacy of standalone cell therapies. AdMSC-SPIONs facilitated partially preserved nerve structure with intact myelinated axons and notable restoration of myelin basic protein organization, indicating remyelination and improved nerve conduction. This non-invasive, non-traumatic approach demonstrates promising potential for enhancing sciatic nerve regeneration post-trauma, like traumatic peripheral nerve injuries often leading to mobility impairment, validating magnetic targeting efficacy in complex in vivo models.86
Another approach utilised neural stem cell (NSC) therapy for neuron replacement. However, limited NSC differentiation into neurons hampers efficacy. To address this, neuron-specific miR-124 promotes NSC maturation but faces challenges like nuclease degradation and poor uptake. Here, they proposed a Ca-MOF@miR-124 nanodelivery system to stabilize and enhance miR-124 uptake by NSCs. In vitro experiments confirmed accelerated neuronal differentiation with Ca-MOF@miR-124, while in vivo studies combining NSC therapy with Ca-MOF@miR-124 nanoparticles reduced the ischemic area significantly within 7 days. This innovative approach shows promise for treating ischemic stroke and other neurodegenerative conditions by promoting rapid NSC differentiation and functional neuronal recovery in cases of strokes, which is a principal source of disability worldwide (Fig. 6).87
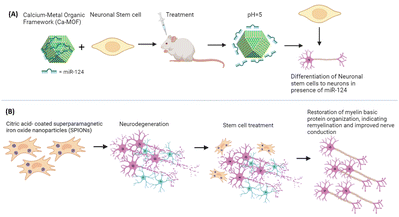 |
| Fig. 6 Approaches to use nanoparticle mediated stem cell therapy. (A) Ca-MOF is used to deliver miR-124 in vivo to increase its stability. This miR dissociates from the Ca-MOF at pH 5, which then helps the neuronal stem cells to differentiate into neurons. (B) Citric acid coated iron oxide nanoparticles were internalized into adipose derived mesenchymal stem cells, which are then magnetically directed to the site of injury. This treatment helps in restoring myelin organisation which indicates improved nerve conduction. | |
Another study on neuronal stem cells explored neural tissue engineering, using materials that support stem cell adhesion, directed movement, and differentiation into neurons and glial cells. They developed bioresorbable elastomeric scaffolds with structured nanopatterned topography and graphene oxide (GO) surface functionalization under mild conditions. These scaffolds enabled murine neural stem cells (NSCs) to adhere and differentiate into immature neurons and glial cells without additional extracellular matrix proteins. NSCs migrated along nanostructured grooves, promoting spatially oriented cell connectivity.48 Researchers have worked with olibanum–collagen–gelatin scaffolds built using freeze-cast technology to aid nerve regeneration. Olibanum and collagen, sourced from natural materials, were utilized. Scanning electron microscopy revealed a lamellar microstructure, with smaller pore sizes associated with higher freezing rates. Scaffold strength decreased slightly at lower freezing rates, while swelling and biodegradation rates increased, attributed to bigger pores and unidirectional channels. The scaffolds’ constitution and aligned microstructure enhanced cellular interactions, particularly adhesion, spreading, and proliferation, indicating potential for further in vitro and in vivo studies on neural defect regeneration.40
8. Cardiovascular diseases
Myocardial damage leads to loss of the cardiac tissue, which poses as a major problem worldwide.88 The protective effects were evaluated for human amniotic membrane-derived MSCs (hAMSCs) labeled with superparamagnetic iron oxide nanoparticles (SPIONs) for isoproterenol (ISO)-induced myocardial injury, with or without a magnetic field. hAMSCs loaded with 100 μg ml−1 SPIONs were administered intravenously, either with or without magnetic guidance. SPION-labeled MSCs with magnetic guidance significantly enhanced cardiac function, reduced fibrosis, and attenuated tissue damage by controlling inflammation through NF-κB/MAPK pathways. These results suggest SPION-labeled MSCs guided magnetically could be promising for managing inflammation following myocardial injury.89
9. Preeclampsia
Early-onset preeclampsia (EOPE) is a serious pregnancy complication linked to abnormal trophoblast differentiation and function, with symptoms appearing in late pregnancy. There is currently lack of a solid method for early diagnosis and therapy. Adrenomedullin (ADM), a peptide abundantly expressed in the placenta during early pregnancy, was found to be lower in chorionic villi and serum of initial three-month pregnant women who subsequently developed EOPE. In-vitro studies revealed that ADM promotes differentiation of stem cells of trophoblasts and organoids. In pregnant mice, placental ADM repression caused EOPE-like symptoms, which were mitigated by placenta-specific lipid polymer nanoparticle-based ADM expression.90
10. Organoids
Organoids are 3-dimensional structures derived from stem cells that mirror the architecture and functionality of biological systems. They are created by culturing tissue-resident stem cells in a supportive extracellular matrix, allowing them to self-organize into complex structures that resemble specific organ tissues as shown in Fig. 7.91,92 They have applications in studying organ development, disease modelling, drug screening and personalized medicine and in understanding disease mechanisms, including cancer and genetic disorders, and hold promise for regenerative medicine by providing a source of cells for transplantation or tissue engineering.93,94
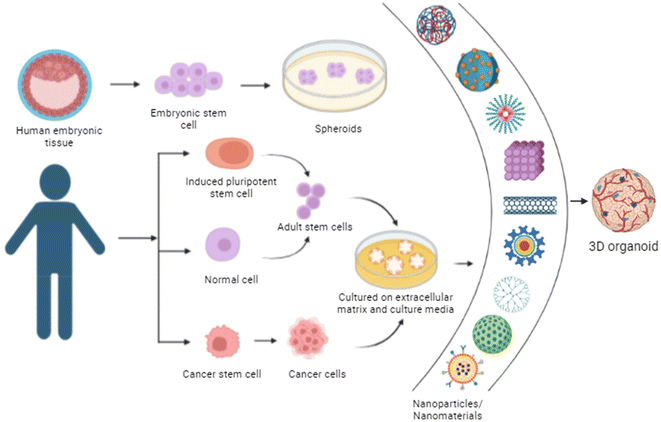 |
| Fig. 7 Organoid formation using stem cells and nanoparticles/nanomaterials. Stem cells can be derived from various sources: embryonic stem cells (ESCs) are obtained from blastocysts and can form spheroids; induced pluripotent stem cells (iPSCs) are generated by reprogramming somatic cells to a pluripotent state; adult stem cells are sourced from specific tissues within organs; and cancer stem cells exhibit stem-like properties within tumors. When cultured with the extracellular matrix in appropriate media, these stem cells proliferate and grow. Nanoparticles and nanomaterials can then guide their differentiation into specific cell lineages and facilitate the formation of organoids. | |
Nanomaterials combined with biological components can be used as scaffolds. Among many other molecules, peptides are particularly attractive due to their ability to self-assemble with target materials with high specificity, without varying the inherent characteristics of nanomaterials. A group studied a 1D hybrid nanomaterial which was created through the self-assembly of a tailored peptide. This peptide featured a hexagonal coiled-coil motif, which matched geometrically to the diameter of an inorganic-based nanomaterial, with a hydrophobic surface encircling the core's axis. Structural and spectroscopic studies revealed a homogeneous, rod-like complex of a peptide–inorganic nanomaterial. When embryonic stem cells were cultured on surfaces treated with this hybrid material, cell growth and adhesion were enhanced. Additionally, the hybrid nanomaterial acted as an extracellular matrix for brain organoids, increasing neuron maturation. This method of fabricating hybrid materials through peptide assembly has promising applications.95–97
11. Nanochips and biosensors
A group of researchers presented a novel chip-supported approach for in situ analysis of patient-derived glioblastoma stem cells (GSCs), utilizing single-clone biomimetic motility assays and nanochannel-based electroporation (NEP). Unlike previous methods such as microelectroporation (MEP) or fluidic delivery,98 NEP minimizes cell damage by targeting nanometer-sized membrane regions and enables rapid, controlled cargo delivery. This advanced platform not only allows real-time monitoring of single-cell movement but also links migratory behaviour to intracellular molecular profiles and therapy resistance, offering new insights for therapeutic development and tumor biology. This on-chip analysis has uncovered notable variations in GSC motility between tumors, which are consistent with findings from tumor xenograft studies and gene expression profiles. In the more aggressive GSC population, the chip detected notable interclonal variations in motility and gene expression. Additionally, chip-based resistance studies with temozolomide and anti-miR363 identified a CD44-high GSC subpopulation with strong antiapoptotic behaviour and enhanced motility. This chip platform offers a unique capability for large-scale, single-cell resolution analysis of heterogeneous cancer cell populations, providing valuable insights into GSC dissemination and therapy resistance mechanisms.99 A different study presented a nanosensor platform for non-invasive, real-time tracking of embryonic stem cells (ESCs) which are vital for studying early embryonic development and tissue regeneration therapies. Unlike traditional cell trackers like DiO or GFP, nanosensors provide uniform and efficient labeling of ESCs. Once internalized, the nanosensors release non-fluorescent molecules which fluoresce only in viable cells, enabling constant monitoring of ESCs within embryoid bodies (EBs). Importantly, nanosensor labeling does not significantly affect the biological properties of ESCs or EBs.46 Tracking how viable ESCs are distributed within EBs plays a key role in the maturation process of EBs. Non-invasive, real-time tracking of ESC distribution allows for the optimization of culture conditions and selection of suitable EBs for further applications.
12. Applications
There have been various applications of nanoparticles and nanomaterials in stem cell engineering. An interesting work introduced an innovative bioswitchable miR inhibitor delivery system (BiRDS) using tetrahedral framework DNA (tFNA). This system incorporates three miR inhibitors (representing immortal birds) fused within a nucleic acid core (symbolizing the central sun), maximizing loading capacity while ensuring stability and minimizing RNA exposure. Each miR inhibitor within BiRDS features an RNase H-responsive sequence that induces a conversion from 3D to 2D upon cellular entry, facilitating efficient delivery. Upon application to deliver the miR-31 inhibitor in a skin aging model, BiRDS demonstrated superior skin penetration and RNA delivery, resulting in significant anti-aging effects on hair follicle stem cells. This study underscored BiRDS’ potential to enhance miR therapy efficacy through improved stability and delivery precision.100
Another study explored human induced pluripotent cells (hiPSCs); the neural crest is a source of multipotent stem cells which have the ability to differentiate into diverse types of cells. SOX10, a transcription factor, is expressed in early neural crest progenitors (NCPs) and stem cells. They generated SOX10-Nano-lantern (NL) reporter hiPSCs using CRISPR/Cas9 to study NCPs. SOX10-NL-positive cells were transiently induced from hiPSCs using the GSK3 inhibitor CHIR99021 and the TGFβ inhibitor SB431542. These cells expressed the early neural crest marker NGFR, but their NL expression decreased over time in culture. Efforts were made to sustain SOX10-NL-positive cells under different culture conditions, facilitating large-scale neural crest cell research.101 Another concept of nanoghosts came to the surface where they targeted osteoarthritis (OA), which is a chronic degenerative joint disease marked by cartilage loss, inflammation and bone changes, with current therapies only addressing symptoms. They explored a novel treatment using mesenchymal stem cell (MSC) cytoplasmic membrane-based nanoparticles, termed nanoghosts (NGs). In this technique, NGs retain MSC surface properties, allowing immunomodulatory capacity and immune evasion without the internal cellular machinery. In vitro and in vivo experiments showed the ability of NGs to target cartilage tissue and control inflammation, slowing cartilage degeneration. NGs also showed potential as versatile nanocarriers for therapeutics targeting both healthy and inflamed cartilage cells, highlighting their promise for treating inflammation-related diseases.102 Furthermore, in dentistry, new barrier membranes with enhanced biomechanical properties are critical for periodontal tissue regeneration. A group of researchers developed electrospun polycaprolactone (PCL)/gelatin (Gel) membranes incorporating bioglass (BG) and copper-doped bioglass (CuBG), and assessed their performance with alveolar bone marrow-derived MSCs (aBMSCs). Incorporation of gelatin and CuBG improved membrane properties, including wettability and mechanical strength. While PCL/Gel/CuBG showed least initial cell attachment, cells proliferated effectively and attained confluence within seven days. All modified membranes demonstrated good biocompatibility and bioactivity, with Cu-doped membranes showing superior potential for periodontal tissue regeneration.103 While working with nanoparticles and nanomaterials, it is also important to understand the mechanisms in which the body reacts to the treatment, hence one study assessed the impact of chitosan films on MSC immunomodulation. Current research suggests that mesenchymal stem cells (MSCs) exert paracrine immunomodulatory effects through the release of nitric oxide (NO), interleukin-10 (IL-10) and transforming growth factor-beta (TGF-β). LPS (lipopolysaccharide) treatment and spheroid formation significantly increased IL-10 and TGF-β levels, and spheroids showed a 7.13-fold increase in arginase activity. LPS treatment significantly elevated NO levels, while chitosan films alone did not affect the NO levels. The results suggest that chitosan films enhance MSC synthesis of immunosuppressive factors in vitro, specifically IL-10, TGF-β, and arginase activities.104
13 Challenges and future perspectives
Nanotechnology presents both significant opportunities and notable challenges in stem cell therapy. There are certain issues associated with it like cytotoxicity, where nanoparticles can trigger oxidative stress, inflammation, and apoptosis in stem cells, varying based on nanoparticle type, size, and surface properties. Additionally, the interaction between nanoparticles and stem cells can disrupt normal differentiation and function, potentially leading to adverse effects such as aberrant cell behaviour or tumorigenesis. Manufacturing and quality control also pose challenges, as ensuring consistent production and characterization of nanomaterials is crucial but difficult. Variability in nanoparticle properties can result in inconsistent therapeutic outcomes, further complicated by a lack of standardized protocols for regulatory approval and clinical application.105–109 The current market for nanoparticles utilized in stem cell isolation, tracking, and differentiation is limited, with only a select few nanoparticle types in active use, such as quantum dots, silica nanoparticles, carbon nanotubes, and gold nanoparticles. A primary challenge associated with these nanoparticles is their high cost, which restricts accessibility and scalability in research and therapeutic applications.110
Another major concern is the potential for SCs to migrate to unintended tissues after intravenous injection, which can lead to variable differentiation signals and potential embolism. Additionally, the immune system may rapidly clear MSCs from circulation, and cell heterogeneity can result in inconsistent therapeutic outcomes. Strategies to improve SC targeting include targeted drug delivery, magnetic guidance, gene modification, and surface modification. However, preparing MSCs to meet therapeutic standards can be time-consuming and costly, especially in urgent situations.111–114
Despite these challenges, nanotechnology holds promise for advancing stem cell therapies. It offers the potential to enhance stem cell functionality, including improved proliferation, differentiation, and treatment of diseases like cancer and neurodegenerative disorders. Furthermore, nanomaterials can facilitate innovative drug delivery systems that precisely target therapeutic agents, potentially increasing efficacy and minimizing side effects. Overcoming the current challenges will require continued interdisciplinary research and collaboration to ensure safety, efficacy, and regulatory compliance, ultimately advancing regenerative medicine and therapeutic strategies.
14. Conclusions
In conclusion, nanotechnology has emerged as a transformative tool in stem cell medicine, offering novel solutions to critical challenges in cell isolation, targeted delivery, tracking, and differentiation. By enhancing precision through techniques like magnetic cell sorting, fluorescent tagging, and controlled delivery systems, nanotechnology significantly improves the therapeutic efficacy and specificity of stem cell-based treatments. The integration of nanomaterials and scaffolds has further enabled controlled stem cell differentiation, activating molecular pathways that support tissue-specific development. These advancements hold potential in addressing complex medical conditions, such as cancer stem cell targeting, neurodegenerative diseases, preeclampsia, cardiovascular disorders, and tissue engineering through organoid development. However, the field still faces considerable challenges, including high costs, biocompatibility, and scalability of nanoparticle applications, as well as the need for regulatory standardization to facilitate clinical translation. Future research focused on these limitations will be crucial to fully harness nanotechnology's potential in advancing stem cell therapies across a wider range of clinical applications.
Data availability
No new data were generated for this manuscript.
Conflicts of interest
There are no conflicts to declare.
Acknowledgements
We thank all the members of SG and DDB labs for reading the manuscript and for their valuable feedback. The work in host labs is funded by GSBTM, Gujcost-DST, SERB-ANRF and STARS-MoES, GoI.
References
- W. Zakrzewski, M. Dobrzyński, M. Szymonowicz and Z. Rybak, Stem cells: past, present, and future, Stem Cell Res. Ther., 2019, 10(1), 68, DOI:10.1186/s13287-019-1165-5.
- J. Vasanthan, N. Gurusamy, S. Rajasingh, V. Sigamani, S. Kirankumar and E. L. Thomas,
et al., Role of Human Mesenchymal Stem Cells in Regenerative Therapy, Cells, 2021, 10(1), 54 CrossRef PubMed , Available from: https://www.mdpi.com/2073-4409/10/1/54.
- T. T. K. K. Tam, S. Xu, P. Liu and A. De Los Angeles, Dawn of development: Exploring early human embryogenesis using stem cells, Cell Stem Cell, 2023, 30(8), 1006–1007 CrossRef PubMed . Available from: https://www.sciencedirect.com/science/article/pii/S1934590923002540.
- E. Coalson, E. Bishop, W. Liu, Y. Feng, M. Spezia and B. Liu,
et al., Stem cell therapy for chronic skin wounds in the era of personalized medicine: From bench to bedside, Genes Dis., 2019, 6(4), 342–358 CrossRef . Available from: https://www.sciencedirect.com/science/article/pii/S2352304219300790.
- Z. Tian, T. Yu, J. Liu, T. Wang and A. Higuchi, Introduction to stem cells, Prog. Mol. Biol. Transl. Sci., 2023, 199, 3–32 Search PubMed.
- G. Kolios and Y. Moodley, Introduction to stem cells and regenerative medicine, Respiration, 2013, 85(1), 3–10 CrossRef PubMed.
- H. F. Bahmad, M. K. Elajami, R. Daouk, H. Jalloul, B. Darwish and R. M. Chalhoub,
et al., Stem Cells: In Sickness and in Health, Curr. Stem Cell Res. Ther., 2021, 16(3), 262–276 Search PubMed.
-
J.M. Riveiro and A. R. Brickman, From pluripotency to totipotency: an experimentalist‘s guide to cellular potency, 2020 Search PubMed.
- L. Bacakova, J. Zarubova, M. Travnickova, J. Musilkova, J. Pajorova and P. Slepicka,
et al., Stem cells: their source, potency and use in regenerative therapies with focus on adipose-derived stem cells - a review, Biotechnol. Adv., 2018, 36(4), 1111–1126 CrossRef PubMed.
- V. K. Singh, A. Saini, M. Kalsan, N. Kumar and R. Chandra, Describing the Stem Cell Potency: The Various Methods of Functional Assessment and In silico Diagnostics, Front. Cell Dev. Biol., 2016, 4, 134 Search PubMed.
-
D. Suhag, P. Thakur and A. Thakur, Introduction to Nanotechnology, in Integrated Nanomaterials and their Applications, ed. D. Suhag, A. Thakur and P. Thakur, Springer Nature Singapore, Singapore, 2023, p. 1–17. DOI:10.1007/978-981-99-6105-4_1.
- C. Salvador-Morales and P. Grodzinski, Nanotechnology Tools Enabling Biological Discovery, ACS Nano, 2022, 16(4), 5062–5084 CrossRef.
- M. J. R. Virlan, D. Miricescu, R. Radulescu, C. M. Sabliov, A. Totan and B. Calenic,
et al., Organic Nanomaterials and Their Applications in the Treatment of Oral Diseases, Molecules, 2016, 21(2), 207 CrossRef.
- B. H. Alshammari, M. M. A. Lashin, M. A. Mahmood, F. S. Al-Mubaddel, N. Ilyas and N. Rahman,
et al., Organic and inorganic nanomaterials: fabrication, properties and applications, RSC Adv., 2023, 13(20), 13735–13785 RSC.
- J. Lu, Z. Jiang, J. Ren, W. Zhang, P. Li and Z. Chen,
et al., One-Pot Synthesis of Multifunctional Carbon-Based Nanoparticle-Supported Dispersed Cu2+ Disrupts Redox Homeostasis to Enhance CDT, Angew. Chem., Int. Ed., 2022, 61(4), e202114373, DOI:10.1002/anie.202114373.
- K. Buskaran, M. Z. Hussein, M. A. M. Moklas, M. J. Masarudin and S. Fakurazi, Graphene Oxide Loaded with Protocatechuic Acid and Chlorogenic Acid Dual Drug Nanodelivery System for Human Hepatocellular Carcinoma Therapeutic Application, Int. J. Mol. Sci., 2021, 22(11), 5786 CrossRef PubMed , Available from: https://www.mdpi.com/1422-0067/22/11/5786.
- P. Yadav, D. Benner, R. Varshney, K. Kansara, K. Shah and L. Dahle,
et al., Dopamine-Functionalized, Red Carbon Quantum Dots for In Vivo Bioimaging, Cancer Therapeutics, and Neuronal Differentiation, ACS Appl. Bio Mater., 2024, 7(6), 3915–3931, DOI:10.1021/acsabm.4c00249.
- Z. Yi, Z. Luo, N. D. Barth, X. Meng, H. Liu and W. Bu,
et al., In Vivo Tumor Visualization through MRI Off-On Switching of NaGdF4−CaCO3 Nanoconjugates, Adv. Mater., 2019, 31(37), 1901851, DOI:10.1002/adma.201901851.
- Y. Li, J. Liu, Z. Wang, J. Jin, Y. Liu and C. Chen,
et al., Optimizing Energy Transfer in Nanostructures Enables In Vivo Cancer Lesion Tracking via Near-Infrared Excited Hypoxia Imaging, Adv. Mater., 2020, 32(14), 1907718, DOI:10.1002/adma.201907718.
- M. Zhao, B. Li, P. Wang, L. Lu, Z. Zhang and L. Liu,
et al., Supramolecularly Engineered NIR-II and Upconversion Nanoparticles In Vivo Assembly and Disassembly to Improve Bioimaging, Adv. Mater., 2018, 30(52), 1804982, DOI:10.1002/adma.201804982.
- T. Liu, L. Tong, N. Lv, X. Ge, Q. Fu and S. Gao,
et al., Two-Stage Size Decrease and Enhanced Photoacoustic Performance of Stimuli-Responsive Polymer-Gold Nanorod Assembly for Increased Tumor Penetration, Adv. Funct. Mater., 2019, 29(16), 1806429, DOI:10.1002/adfm.201806429.
- A. Buonerba, R. Lapenta, A. Donniacuo, M. Licasale, E. Vezzoli and S. Milione,
et al., NIR multiphoton ablation of cancer cells, fluorescence quenching and cellular uptake of dansyl-glutathione-coated gold nanoparticles, Sci. Rep., 2020, 10(1), 11380, DOI:10.1038/s41598-020-68397-1.
- T. Park, S. Lee, R. Amatya, H. Cheong, C. Moon, H. D. Kwak, K. A. Min and M. C. Shin, ICG-Loaded PEGylated BSA-Silver Nanoparticles for Effective Photothermal Cancer Therapy, Int. J. Nanomed., 2020, 15, 5459–5471, DOI:10.2147/IJN.S255874.
- R. R. Silva, D. V. Freitas, F. L. N. Sousa, A. C. Jesus, S. E. Silva and A. A. P. Mansur,
et al., Synthesis of CuInS2 and CuInS2@ZnX (X = S, Se) nanoparticles for bioimaging of cancer cells using electrochemically generated S2- and Se2, J. Alloys Compd., 2021, 853, 156926 CrossRef . Available from: https://www.sciencedirect.com/science/article/pii/S0925838820332904.
- N. Joudeh and D. Linke, Nanoparticle classification, physicochemical properties, characterization, and applications: a comprehensive review for biologists, J. Nanobiotechnol., 2022, 20(1), 262, DOI:10.1186/s12951-022-01477-8.
- D. Alzate-Correa, W. R. Lawrence, A. Salazar-Puerta, N. Higuita-Castro and D. Gallego-Perez, Nanotechnology-Driven Cell-Based Therapies in Regenerative Medicine, AAPS J., 2022, 24(2), 43 CrossRef PubMed.
- R. Atchudan, S. Chandra Kishore, P. Gangadaran, T. N. Jebakumar Immanuel Edison, S. Perumal and R. L. Rajendran,
et al., Tunable fluorescent carbon dots from biowaste as fluorescence ink and imaging human normal and cancer cells, Environ. Res., 2022, 204, 112365 CrossRef PubMed . Available from: https://www.sciencedirect.com/science/article/pii/S0013935121016662.
- S. Li, W. Su, H. Wu, T. Yuan, C. Yuan and J. Liu,
et al., Targeted tumour theranostics in mice via carbon quantum dots structurally mimicking large amino acids, Nat. Biomed. Eng., 2020, 4(7), 704–716, DOI:10.1038/s41551-020-0540-y.
- W. Su, R. Guo, F. Yuan, Y. Li, X. Li and Y. Zhang,
et al., Red-Emissive Carbon Quantum Dots for Nuclear Drug Delivery in Cancer Stem Cells, J. Phys. Chem. Lett., 2020, 11(4), 1357–1363, DOI:10.1021/acs.jpclett.9b03891.
- A. C. Taylor, B. Vagaska, R. Edgington, C. Hébert, P. Ferretti and P. Bergonzo,
et al., Biocompatibility of nanostructured boron doped diamond for the attachment and proliferation of human neural stem cells, J. Neural Eng., 2015, 12(6), 066016 CrossRef PubMed.
- Z. Du, X. Feng, G. Cao, Z. She, R. Tan and K. E. Aifantis,
et al., The effect of carbon nanotubes on osteogenic functions of adipose-derived mesenchymal stem cells in vitro and bone formation in vivo compared with that of nano-hydroxyapatite and the possible mechanism, Bioact. Mater., 2021, 6(2), 333–345 Search PubMed . Available from: https://www.sciencedirect.com/science/article/pii/S2452199X20301717.
- A. Shafiee, M. Kehtari, Z. Zarei, M. Soleimani, R. Varshochian and A. Ahmadi,
et al., An in situ hydrogel-forming scaffold loaded by PLGA microspheres containing carbon nanotube as a suitable niche for neural differentiation, Mater. Sci. Eng., C, 2021, 120, 111739 CrossRef PubMed . Available from: https://www.sciencedirect.com/science/article/pii/S0928493120336584.
- K. D. Patel, T.-H. Kim, N. Mandakhbayar, R. K. Singh, J.-H. Jang and J.-H. Lee,
et al., Coating biopolymer nanofibers with carbon nanotubes accelerates tissue healing and bone regeneration through orchestrated cell- and tissue-regulatory responses, Acta Biomater., 2020, 108, 97–110 CrossRef PubMed . Available from: https://www.sciencedirect.com/science/article/pii/S1742706120301446.
- T. Foroutan, F. Kabiri and E. Motamedi, Silica Magnetic Graphene Oxide Improves the Effects of Stem Cell-Conditioned Medium on Acute Liver Failure, ACS Omega, 2021, 6(33), 21194–21206, DOI:10.1021/acsomega.0c05395.
- G. A. Martín-Henao, M. Picón, F. Rueda, B. Amill, S. Querol and E. Gonzalez-Barca,
et al., Combined isolation of CD34+ progenitor cells and reduction of B cells from peripheral blood by use of immunomagnetic methods, Transfusion, 2002, 42(7), 912–920 CrossRef PubMed.
- A. Haleem, M. Javaid, R. P. Singh, S. Rab and R. Suman, Applications of nanotechnology in medical field: a brief review, Glob. Health J., 2023, 7(2), 70–77 CrossRef . Available from: https://www.sciencedirect.com/science/article/pii/S2414644723000337.
- V. Alimardani, Z. Rahiminezhad, M. DehghanKhold, G. Farahavar, M. Jafari and M. Abedi,
et al., Nanotechnology-based cell-mediated delivery systems for cancer therapy and diagnosis, Drug Delivery Transl. Res., 2023, 13(1), 189–221 CrossRef PubMed.
- . Nano Select - 2021 - Kumar - The convergence of nanotechnology–stem cell nanotopography–mechanobiology and biotic–abiotic.pdf.
- Y. Polo Arroyabe, J. Luzuriaga, J. Iturri, J. L. Toca-Herrera, G. Ibarretxe and F. Unda,
et al., Nanostructured scaffolds based on Bioresorbable polymers and Graphene oxide induce the aligned migration and accelerate the neuronal differentiation of neural stem cells, Nanomedicine, 2020, 31, 102314 CrossRef.
- F. Ghorbani, A. Zamanian, F. Kermanian and A. Shamoosi, A bioinspired 3D shape olibanum-collagen-gelatin scaffolds with tunable porous microstructure for efficient neural tissue regeneration, Biotechnol. Prog., 2020, 36(1), e2918, DOI:10.1002/btpr.2918.
- A. Moeinzadeh, B. Ashtari, H. Garcia, M. Koruji, C. A. Velazquez and Z. Bagher,
et al., The Effect of Chitosan/Alginate/Graphene Oxide Nanocomposites on Proliferation of Mouse Spermatogonial Stem Cells, J. Funct. Biomater., 2023, 14(12), 556 CrossRef.
- E. Lestrell, C. M. O'Brien, R. Elnathan and N. H. Voelcker, Vertically Aligned Nanostructured Topographies for Human Neural Stem Cell Differentiation and Neuronal Cell Interrogation, Adv. Ther., 2021, 4(9), 2100061, DOI:10.1002/adtp.202100061.
-
P. Samuel, S. Sundarraj and D. Sudarmani, Nanotechnology-Based Stem Cell Therapy: Current Status and Perspectives, 2023 Search PubMed.
- X. Wen, Y. Zou, L. Li, H. Nie, K. Wang and J. Wang,
et al., Nondestructive isolation of mesenchymal stem cells from bone marrow using
DNA aptamers, Analyst, 2022, 147(22), 5203–5209, 10.1039/D2AN01086K.
- J. Han, M. Won, J. H. Kim, E. Jung, K. Min and P. Jangili,
et al., Cancer stem cell-targeted bio-imaging and chemotherapeutic perspective, Chem. Soc. Rev., 2020, 49(22), 7856–7878 RSC.
- J. Fu, C. Wiraja, R. Chong, C. Xu and D.-A. Wang, Real-time and non-invasive monitoring of embryonic stem cell survival during the development of embryoid bodies with smart nanosensor, Acta Biomater., 2017, 49, 358–367 CrossRef.
- M. Samiei, F. Arablouye Moghaddam, E. Dalir Abdolahinia, E. Ahmadian, S. Sharifi and S. Maleki Dizaj, Influence of Curcumin Nanocrystals on the Early Osteogenic Differentiation and Proliferation of Dental Pulp Stem Cells, J. Nanomater., 2022, 2022(1) DOI:10.1155/2022/8517543.
- Y. Polo, J. Luzuriaga, J. Iturri, I. Irastorza, J. L. Toca-Herrera and G. Ibarretxe,
et al., Nanostructured scaffolds based on bioresorbable polymers and graphene oxide induce the aligned migration and accelerate the neuronal differentiation of neural stem cells, Nanomedicine, 2021, 31, 102314 CrossRef PubMed . Available from: https://www.sciencedirect.com/science/article/pii/S1549963420301684.
- S. Jasty, S. Suriyanarayanan and S. Krishnakumar, Influence of self-assembling peptide nanofibre scaffolds on retinal differentiation potential of stem/progenitor cells derived from ciliary pigment epithelial cells, J. Tissue Eng. Regener. Med., 2017, 11(2), 509–518 CrossRef PubMed.
- K. Adibkia, A. Ehsani, A. Jodaei, E. Fathi, R. Farahzadi and M. Barzegar-Jalali, Silver nanoparticles induce the cardiomyogenic differentiation of bone marrow derived mesenchymal stem cells via telomere length extension, Beilstein J. Nanotechnol., 2021, 12, 786–797, DOI:10.3762/bjnano.12.62.
- J. Liu, Y. Cheng, H. Wang, D. Yang, C. Liu and W. Dou,
et al., Regulation of TiO2@PVDF piezoelectric nanofiber membranes on osteogenic differentiation of mesenchymal stem cells, Nano Energy, 2023, 115, 108742 CrossRef . Available from: https://www.sciencedirect.com/science/article/pii/S2211285523005797.
- I. Ishmukhametov, S. Batasheva, E. Rozhina, F. Akhatova, R. Mingaleeva and A. Rozhin,
et al., DNA/Magnetic Nanoparticles Composite to Attenuate Glass Surface Nanotopography for Enhanced Mesenchymal Stem Cell Differentiation, Polymers, 2022, 14(2), 344 CrossRef PubMed , Available from: https://www.mdpi.com/2073-4360/14/2/344.
- S. R. Dash, S. Chatterjee, S. Sinha, B. Das, S. Paul and R. Pradhan,
et al., NIR irradiation enhances the apoptotic potentiality of quinacrine-gold hybrid nanoparticles by modulation of HSP-70 in oral cancer stem cells, Nanomedicine, 2022, 40, 102502 CrossRef PubMed . Available from: https://www.sciencedirect.com/science/article/pii/S1549963421001453.
- E. Pishavar, R. Yazdian-Robati, K. Abnous, M. Hashemi, M. Ebrahimian and R. Feizpour,
et al., Aptamer-functionalized mesenchymal stem cells-derived exosomes for targeted delivery of SN38 to colon cancer cells, Iran. J. Basic Med. Sci., 2023, 26(4), 388–394 Search PubMed.
- Z. Wang, J. Ruan and D. Cui, Advances and prospect of nanotechnology in stem cells, Nanoscale Res. Lett., 2009, 4(7), 593–605 CrossRef.
- Y. Dong, X. Wu, X. Chen, P. Zhou, F. Xu and W. Liang, Nanotechnology shaping stem cell therapy: Recent advances, application, challenges, and future outlook, Biomed. Pharmacother., 2021, 137, 111236 CrossRef PubMed . Available from: https://www.sciencedirect.com/science/article/pii/S0753332221000214.
- M. Maqsood, M. Kang, X. Wu, J. Chen, L. Teng and L. Qiu, Adult mesenchymal stem cells and their exosomes: Sources, characteristics, and application in regenerative medicine, Life Sci., 2020, 256, 118002 CrossRef PubMed . Available from: https://www.sciencedirect.com/science/article/pii/S0024320520307529.
- J. K. Biehl and B. Russell, Introduction to stem cell therapy, J. Cardiovasc. Nurs., 2009, 24(2), 95–98 CrossRef.
-
G. M. C. Rodrigues, T. G. Fernandes, C. A. V. Rodrigues, J. M. S. Cabral and M. M. Diogo, Purification of Human Induced Pluripotent Stem Cell-Derived Neural Precursors Using Magnetic Activated Cell Sorting, in Stem Cells and Good Manufacturing Practices: Methods, Protocols, and Regulations, ed. K. Turksen, Springer New York, New York, NY, 2015, pp. 137–145. DOI:10.1007/7651_2014_115.
- H. Sun, J. Chen, B. Ni, X. Yang and Y. Wu, Recent advances and current issues in single-cell sequencing of tumors, Cancer Lett., 2015, 365(1), 1–10 CrossRef . Available from: https://www.sciencedirect.com/science/article/pii/S0304383515002906.
- C. Vissers, G.-L. Ming and H. Song, Nanoparticle technology and stem cell therapy team up against neurodegenerative disorders, Adv. Drug Delivery Rev., 2019 Ag, 148, 239–251 Search PubMed.
- A. Grützkau and A. Radbruch, Small but mighty: How the MACS®-technology based on nanosized superparamagnetic particles has helped to analyze the immune system within the last 20 years, Cytometry, Part A, 2010, 77A(7), 643–647, DOI:10.1002/cyto.a.20918.
- M. Frenea-Robin and J. Marchalot, Basic Principles and Recent Advances in Magnetic Cell Separation, Magnetochemistry, 2022, 8(1), 11 CrossRef , Available from: https://www.mdpi.com/2312-7481/8/1/11.
- Q. Wang, X. Ma, H. Liao, Z. Liang, F. Li and J. Tian,
et al., Artificially Engineered Cubic Iron Oxide Nanoparticle as a High-Performance Magnetic Particle Imaging Tracer for Stem Cell Tracking, ACS Nano, 2020, 14(2), 2053–2062, DOI:10.1021/acsnano.9b08660.
- H. Cao, Y. Cheng, H. Gao, J. Zhuang, W. Zhang and Q. Bian,
et al., In Vivo Tracking of Mesenchymal Stem Cell-Derived Extracellular Vesicles Improving Mitochondrial Function in Renal Ischemia–Reperfusion Injury, ACS Nano, 2020, 14(4), 4014–4026, DOI:10.1021/acsnano.9b08207.
- H. Elkhenany, M. Abd Elkodous, N. I. Ghoneim, T. A. Ahmed, S. M. Ahmed and I. K. Mohamed,
et al., Comparison of different uncoated and starch-coated superparamagnetic iron oxide nanoparticles: Implications for stem cell tracking, Int. J. Biol. Macromol., 2020, 143, 763–774 CrossRef PubMed . Available from: https://www.sciencedirect.com/science/article/pii/S0141813019343107.
- Z. Salmasi, M. Hashemi, E. Mahdipour, H. Nourani, K. Abnous and M. Ramezani, Mesenchymal stem cells engineered by modified polyethylenimine polymer for targeted cancer gene therapy, in vitro and in vivo, Biotechnol. Prog., 2020, 36(6), e3025, DOI:10.1002/btpr.3025.
- S. Taghavi, A. H. Nia, K. Abnous and M. Ramezani, Polyethylenimine-functionalized carbon nanotubes tagged with AS1411 aptamer for combination gene and drug delivery into human gastric cancer cells, Int. J. Pharm., 2017, 516(1–2), 301–312 CrossRef.
- Z. Liang, L. Yang and Y. Lv, Exosome derived from mesenchymal stem cells mediates hypoxia-specific BMP2 gene delivery and enhances bone regeneration, Chem. Eng. J., 2021, 422, 130084 CrossRef . Available from: https://www.sciencedirect.com/science/article/pii/S1385894721016697.
- R. El-Kharrag, K. E. Berckmueller, R. Madhu, M. Cui, G. Campoy and H. M. Mack,
et al., Efficient polymer nanoparticle-mediated delivery of gene editing reagents into human hematopoietic stem and progenitor cells, Mol. Ther., 2022, 30(6), 2186–2198 CrossRef.
- M. Sattary, A. B. Amirhosein Kefayat and M. Rafienia, Polycaprolactone/Gelatin/Hydroxyapatite nanocomposite scaffold seeded with Stem cells from human exfoliated deciduous teeth to enhance bone repair: in vitro and in vivo studies, Mater. Technol., 2022, 37(5), 302–315, DOI:10.1080/10667857.2020.1837488.
- S. Cheemerla and M. Balakrishnan, Global Epidemiology of Chronic Liver Disease, Clin. Liver Dis., 2021, 17(5), 365–370 CrossRef.
- H. Devarbhavi, S. K. Asrani, J. P. Arab, Y. A. Nartey, E. Pose and P. S. Kamath, Global burden of liver disease: 2023 update, J. Hepatol., 2023, 79(2), 516–537 CrossRef PubMed . Available from: https://www.sciencedirect.com/science/article/pii/S0168827823001940.
- A. Sharma and B. G. Jaganathan, Stem Cell Therapy for Retinal Degeneration: The Evidence to Date, Biologics, 2021, 15, 299–306 Search PubMed.
- Y. Zhang, P. Wang, Y. Wang, J. Li, D. Qiao, R. Chen, W. Yang and F. Yan, Gold Nanoparticles Promote the Bone Regeneration of Periodontal Ligament Stem Cell Sheets Through Activation of Autophagy, Int. J. Nanomed., 2021, 16, 61–73, DOI:10.2147/IJN.S282246.
- Z. Zeng, M. Fu, Y. Hu, Y. Wei, X. Wei and M. Luo, Regulation and signaling pathways in cancer stem cells: implications for targeted therapy for cancer, Mol. Cancer, 2023, 22(1), 172, DOI:10.1186/s12943-023-01877-w.
- L. Chang, Y. Zhang, M. Li, X. Zhao, D. Wang and J. Liu,
et al., Nanostructured lipid carrier co-delivering paclitaxel and doxorubicin restrains the proliferation and promotes apoptosis of glioma stem cells via regulating PI3K/Akt/mTOR signaling, Nanotechnology, 2021, 32(22), 225101, DOI:10.1088/1361-6528/abd439.
- S.-J. Yoon, S. Baek, S. E. Yu, E. Jo, D. Lee and J.-K. Shim,
et al., Tissue Niche Miniature of Glioblastoma Patient Treated with Nano-Awakeners to Induce Suicide of Cancer Stem Cells, Adv. Healthc. Mater., 2022, 11(21), 2201586, DOI:10.1002/adhm.202201586.
- H. Kantarjian, T. Kadia, C. DiNardo, N. Daver, G. Borthakur and E. Jabbour,
et al., Acute myeloid leukemia: current progress and future directions, Blood Cancer J., 2021, 11(2), 41, DOI:10.1038/s41408-021-00425-3.
- W. Nirachonkul, S. Ogonoki, T. Thumvijit, S. Chiampanichayakul, P. Panyajai and S. Anuchapreeda,
et al., CD123-Targeted Nano-Curcumin Molecule Enhances Cytotoxic Efficacy in Leukemic Stem Cells, Nanomaterials, 2021, 11(11), 2974 CrossRef PubMed , Available from: https://www.mdpi.com/2079-4991/11/11/2974.
- S. Wadhwa, V. Garg, M. Gulati, B. Kapoor, S. K. Singh and N. Mittal, Nanovesicles for Nanomedicine: Theory and Practices, Methods Mol. Biol., 2019, 2000, 1–17 CrossRef PubMed.
- T. E. Kim and J.-E. Chang, Recent Studies in Photodynamic Therapy for Cancer Treatment: From Basic Research to Clinical Trials, Pharmaceutics, 2023, 15(9), 2257 CrossRef CAS PubMed , Available from: https://www.mdpi.com/1999-4923/15/9/2257.
- A. M. Itoo, M. Paul, S. G. Padaga, B. Ghosh and S. Biswas, Nanotherapeutic Intervention in Photodynamic Therapy for Cancer, ACS Omega, 2022, 7(50), 45882–45909, DOI:10.1021/acsomega.2c05852.
- S. Taghavi, H. Tabasi, M. Zahiri, K. Abnous, S. Mohammad Taghdisi and S. Nekooei,
et al., Surface engineering of hollow gold nanoparticle with mesenchymal stem cell membrane and MUC-1 aptamer for targeted theranostic application against metastatic breast cancer, Eur. J. Pharm. Biopharm., 2023, 187, 76–86 CrossRef CAS . Available from: https://www.sciencedirect.com/science/article/pii/S0939641123001005.
- R. N. L. Lamptey, B. Chaulagain, R. Trivedi, A. Gothwal, B. Layek and J. Singh, A Review of the Common Neurodegenerative Disorders: Current Therapeutic Approaches and the Potential Role of Nanotherapeutics, Int. J. Mol. Sci., 2022, 23(3), 1851 CrossRef CAS PubMed.
- P. A. Soto, M. Vence, G. M. Piñero, D. F. Coral, V. Usach and D. Muraca,
et al., Sciatic nerve regeneration after traumatic injury using magnetic targeted adipose-derived mesenchymal stem cells, Acta Biomater., 2021, 130, 234–247 CrossRef CAS PubMed . Available from: https://www.sciencedirect.com/science/article/pii/S1742706121003603.
- H. Yang, M. Han, J. Li, H. Ke, Y. Kong and W. Wang,
et al., Delivery of miRNAs through Metal–Organic Framework Nanoparticles for Assisting Neural Stem Cell Therapy for Ischemic Stroke, ACS Nano, 2022, 16(9), 14503–14516, DOI:10.1021/acsnano.2c04886.
-
N. S. Fathima, Current Research and Trends in Medical Science and Technology-An update on myocardial infarction, 2021, pp. 1–2 Search PubMed.
- M. Naseroleslami, N. Aboutaleb and B. Mokhtari, Amniotic membrane mesenchymal stem cells labeled by iron oxide nanoparticles exert cardioprotective effects against isoproterenol (ISO)-induced myocardial damage by targeting inflammatory MAPK/NF-κB pathway, Drug Delivery Transl. Res., 2021, 11(1), 242–254, DOI:10.1007/s13346-020-00788-3.
- Q. Zhang, C.-L. Lee, T. Yang, J. Li, Q. Zeng and X. Liu,
et al., Adrenomedullin has a pivotal role in trophoblast differentiation: A promising nanotechnology-based therapeutic target for early-onset preeclampsia, Sci. Adv., 2023, 9(44), eadi4777 CrossRef PubMed.
- X.-Y. Tang, S. Wu, D. Wang, C. Chu, Y. Hong and M. Tao,
et al., Human organoids in basic research and clinical applications, Signal Transduction Targeted Ther., 2022, 7(1), 168, DOI:10.1038/s41392-022-01024-9.
- D. de Jongh, E. K. Massey, E. Berishvili, L. M. Fonseca, F. Lebreton and K. Bellofatto,
et al., Organoids: a systematic review of ethical issues, Stem Cell Res. Ther., 2022, 13(1), 337, DOI:10.1186/s13287-022-02950-9.
- J. Clinton and P. McWilliams-Koeppen, Initiation, Expansion, and Cryopreservation of Human Primary Tissue-Derived Normal and Diseased Organoids in Embedded Three-Dimensional Culture, Curr. Protoc. Cell Biol., 2019, 82(1), e66 CrossRef PubMed.
- Y. Takahashi, Y. Inoue, S. Sato, T. Okabe, H. Kojima and H. Kiyono,
et al., Drug cytotoxicity screening using human intestinal organoids propagated with extensive cost-reduction strategies, Sci. Rep., 2023, 13(1), 5407, DOI:10.1038/s41598-023-32438-2.
- E. Parigoris, J.-H. Lee, A. Y. Liu, X. Zhao and S. Takayama, Extended longevity geometrically-inverted proximal tubule organoids, Biomaterials, 2022, 290, 121828 CrossRef PubMed . Available from: https://www.sciencedirect.com/science/article/pii/S0142961222004689.
- E. Driehuis, A. Gracanin, R. G. J. Vries, H. Clevers and S. F. Boj, Establishment of Pancreatic Organoids from Normal Tissue and Tumors, STAR Protoc., 2020, 1(3), 100192 CrossRef PubMed.
- N. H. Kim, S. Chae, S. A. Yi, D. H. Sa, S. Oh and E. S. Kang,
et al., Peptide-Assembled Single-Chain Atomic Crystal Enhances Pluripotent Stem Cell Differentiation to Neurons, Nano Lett., 2023, 23(15), 6859–6867, DOI:10.1021/acs.nanolett.3c00966.
- R. Ziv, Y. Steinhardt, G. Pelled, D. Gazit and B. Rubinsky, Micro-electroporation of mesenchymal stem cells with alternating electrical current pulses, Biomed. Microdevices, 2009, 11(1), 95–101 CrossRef PubMed.
- D. Gallego-Perez, L. Chang, J. Shi, J. Ma, S.-H. Kim and X. Zhao,
et al., On-Chip Clonal Analysis of Glioma-Stem-Cell Motility and Therapy Resistance, Nano Lett., 2016 Sp, 16(9), 5326–5332 Search PubMed.
- S. Li, Y. Liu, T. Zhang, S. Lin, S. Shi and J. He,
et al., A Tetrahedral Framework DNA-Based Bioswitchable miRNA Inhibitor Delivery System: Application to Skin Anti-Aging, Adv. Mater., 2022, 34(46), 2204287, DOI:10.1002/adma.202204287.
- T. Horikiri, H. Ohi, M. Shibata, M. Ikeya, M. Ueno and C. Sotozono,
et al., SOX10-Nano-Lantern Reporter Human iPS Cells; A Versatile Tool for Neural Crest Research, PLoS One, 2017, 12(1), e0170342 CrossRef.
- D. D'Atri, L. Zerrillo, J. Garcia, J. Oieni, Y. Lupu-Haber and T. Schomann,
et al., Nanoghosts: Mesenchymal Stem cells derived nanoparticles as a unique approach for cartilage regeneration, J. Controlled Release, 2021, 337, 472–481 CrossRef PubMed . Available from: https://www.sciencedirect.com/science/article/pii/S0168365921002315.
- B. Malekahmadi, V. Esfahanian, F. Ejeian, M. E. Dastgurdi, M. Agheb and F. Kaveian,
et al., In Vitro Study of the Recruitment and Expansion of Mesenchymal Stem Cells at the Interface of a Cu-Doped PCL-Bioglass Scaffold, Biomimetics, 2022, 7(1), 19 CrossRef PubMed , Available from: https://www.mdpi.com/2313-7673/7/1/19.
- S. Farrokhi, F. Sotoodehnejadnematalahi, A. Fathollahi, M. Haji Molla Hoseini, S. M. Hashemi and F. Yeganeh, The immunomodulatory potential of murine adipose-derived mesenchymal stem cells is enhanced following culture on chitosan film, Tissue Cell, 2022, 74, 101709 CrossRef . Available from: https://www.sciencedirect.com/science/article/pii/S0040816621002251.
- J. Wolfram, M. Zhu, Y. Yang, J. Shen, E. Gentile and D. Paolino,
et al., Safety of Nanoparticles in Medicine, Curr. Drug Targets, 2015, 16(14), 1671–1681 CrossRef.
- A. Abdal Dayem, S. B. Lee and S.-G. Cho, The Impact of Metallic Nanoparticles on Stem Cell Proliferation and Differentiation, Nanomaterials, 2018, 8(10), 761 CrossRef . Available from: https://www.mdpi.com/2079-4991/8/10/761.
- J. M. Köhler, Challenges for Nanotechnology, Encyclopedia, 2021, 1(3), 618–631 CrossRef . Available from: https://www.mdpi.com/2673-8392/1/3/51.
- G. V. Lowry, A. Avellan and L. M. Gilbertson, Opportunities and challenges for nanotechnology in the agri-tech revolution, Nat. Nanotechnol., 2019, 14(6), 517–522, DOI:10.1038/s41565-019-0461-7.
- F. Zhang, Grand Challenges for Nanoscience and Nanotechnology in Energy and Health, Front. Chem., 2017, 5, 80, DOI:10.3389/fchem.2017.00080.
- Y. Wang, C. Xu and H. Ow, Commercial nanoparticles for stem cell labeling and tracking, Theranostics, 2013, 3(8), 544–560 CrossRef.
- S. Ikehara, Grand challenges in stem cell treatments, Front. Cell Dev. Biol., 2013, 1, 2 Search PubMed.
- L. Wei, W. Yan, W. Shah, Z. Zhang, M. Wang and B. Liu,
et al., Advancements and challenges in stem cell transplantation for regenerative medicine, Heliyon, 2024, 10(16), e35836 CrossRef PubMed . Available from: https://www.sciencedirect.com/science/article/pii/S2405844024118671.
- E. Apostolou, H. Blau, K. Chien, M. A. Lancaster, P. R. Tata and E. Trompouki,
et al., Progress and challenges in stem cell biology, Nat. Cell Biol., 2023, 25(2), 203–206, DOI:10.1038/s41556-023-01087-y.
-
W. Tang, Challenges and advances in stem cell therapy, Bioscience trends, Japan, 2019, vol. 13, pp. 286 Search PubMed.
Footnote |
† These authors contributed equally to this work. |
|
This journal is © The Royal Society of Chemistry 2025 |
Click here to see how this site uses Cookies. View our privacy policy here.