DOI:
10.1039/D4CC05280C
(Highlight)
Chem. Commun., 2025,
61, 4586-4594
Unnatural foldamers as inhibitors of Aβ aggregation via stabilizing the Aβ helix
Received
7th October 2024
, Accepted 24th February 2025
First published on 24th February 2025
Abstract
Protein aggregation is a critical factor in the development and progression of several human diseases, including Alzheimer's disease (AD), Huntington's disease, Parkinson's disease, and type 2 diabetes. Among these conditions, AD is recognized as the most prevalent progressive neurodegenerative disorder, characterized by the accumulation of amyloid-beta (Aβ) peptides. Neuronal toxicity is likely driven by soluble oligomeric intermediates of the Aβ peptide, which are thought to play a central role in the cascade leading to neuronal dysfunction and cognitive decline. In response, numerous therapeutic strategies have been developed to inhibit Aβ oligomerization, as this is believed to delay the formation of Aβ protofibrils. Traditional research has focused on discovering small molecules or peptides that antagonize Aβ oligomerization. However, recent studies have explored an alternative approach—developing ligands that stabilize the Aβ peptide in its α-helical conformation. This stabilization is thought to alter the peptide's natural aggregation kinetics, shifting it away from toxic oligomer formation and toward less harmful states. Crucially, by maintaining Aβ in this α-helical form, these ligands have been shown to rescue the peptide's associated cytotoxicity, offering a promising mechanism to mitigate the detrimental effects of Aβ in AD. While challenges remain, including treatment costs and side effects like ARIA (amyloid-related imaging abnormalities), anti-Aβ drug development represents a major advancement in Alzheimer's research and therapeutic options. This brief review aims to highlight the development and potential of these α-helix-stabilizing ligands as antagonists of Aβ aggregation, focusing on their interactions with Aβ and how these compounds induce and maintain secondary structural changes in the Aβ peptide. Notably, this innovative strategy holds promise beyond Aβ-related pathology, as the fundamental principles could be applied to other amyloidogenic proteins implicated in various amyloid-related diseases, potentially broadening the scope of therapeutic intervention for multiple neurodegenerative conditions.
Introduction
The self-assembly of peptides or proteins into fibril species is implicated in a wide range of degenerative diseases, collectively known as protein misfolding disorders.1,2 These diseases are characterized by the transition of specific peptides or proteins from their normal, functional, and soluble forms into highly ordered aggregates or fibrils. This structural transformation is associated with the loss of native function and the gain of toxic properties, contributing to disease progression. Pathological aggregation examples include the formation of amyloid fibrils by amyloid β (Aβ) peptides in Alzheimer's disease (AD), α-synuclein in Parkinson's disease, the polyglutamine (polyQ) expansion of huntingtin in Huntington's disease, β2-microglobulin in hemodialysis-related amyloidosis, and the islet amyloid polypeptide (IAPP) in type-2 diabetes.3,4 AD, the most common form of neurodegenerative disorder, is particularly associated with the Aβ peptide accumulation. These peptides initially exist in soluble forms but ultimately undergo structural reorganization into highly stable amyloid fibrils that deposit in the brain. Aβ peptides, derived from the transmembrane region of the amyloid precursor protein (APP), are generated through enzymatic cleavage, with Aβ40 and Aβ42 being the most prevalent isoforms.5 In aqueous solutions, both peptides predominantly adopt random coil conformations.6 In the presence of agents such as hexafluoroisopropanol (HFIP) or sodium dodecyl sulfate (SDS), both peptides adopt an α-helical structure spanning residues 15–24 and 29–35.7–10 Both Aβ40 and Aβ42 are found in amyloid plaques, a hallmark of Alzheimer's disease. Additionally, both peptides interact with cellular components to cause oxidative stress, inflammation and synaptic dysfunction.10 Despite their similarities, the secondary structures of Aβ40 and Aβ42 differ slightly, as Aβ42 adopts a more rigid conformation at its C-terminus.6,11 Under identical conditions, Aβ42 exhibits a greater tendency to aggregate compared to Aβ40, making it the principal component of amyloid plaques and more neurotoxic.12 Despite the presence of these fibrils in AD pathology, it is now widely accepted that the oligomeric intermediates of Aβ, particularly Aβ42, play a more critical role in neurotoxicity.13 Studies have demonstrated that soluble Aβ oligomers, rather than mature fibrils, are the key drivers of synaptic dysfunction and neuronal death, making them the primary neurotoxic species involved in the onset and progression of AD.14,15 Aβ oligomers contribute to both synaptic dysfunction and neurotoxicity, two distinct yet interconnected processes in Alzheimer's disease. The Aβ concentration required for synaptic dysfunction is in the nanomolar range, leading to the impairment of synaptic signaling and plasticity.16,17 Multiple mechanisms might be involved, including receptor dysfunction, LTP inhibition, spine loss and so on.18,19 The Aβ concentration required for neurotoxicity is in the micromolar range, which involves multiple potential mechanisms behind it.20–22 Membrane disruption plays a critical role in neurotoxicity where Aβ oligomers interact with lipid bilayers, forming pore-like structures.23 These pores would disrupt ion homeostasis by allowing uncontrolled calcium and other ion flux. Besides, Aβ oligomers would generate reactive oxygen species, causing lipid peroxidation, protein oxidation, DNA damage and so on. Some other factors are also included, like mitochondrial dysfunction and neuroinflammation.24 As the understanding of Aβ oligomerization has evolved, therapeutic strategies have shifted toward targeting these early-stage aggregates. Inhibiting the formation of toxic Aβ oligomers offers a potential approach to slowing or halting disease progression before irreversible neuronal damage occurs. This has led to the development of various ligand-based strategies designed to interfere with the oligomerization process. These ligands aim to either prevent Aβ peptides from assembling into oligomeric species or destabilize pre-existing oligomers, thereby reducing their neurotoxic effects. Given the complexity of Aβ aggregation and its central role in AD pathology, further research is crucial to fully elucidate the mechanisms driving oligomerization and to optimize therapeutic approaches. Ongoing efforts continue to explore the structural and kinetic properties of Aβ aggregation, aiming to identify more potent and selective inhibitors capable of mitigating the toxic effects of Aβ oligomers and offer a new hope for patients with Alzheimer's disease and other amyloid-related disorders.
One promising approach in combating Alzheimer's disease involves the use of small molecules, which present several distinct advantages. First, small molecules exhibit excellent stability in biological fluids and tissues, maintaining their functional integrity in complex physiological environments. Additionally, they possess the crucial ability to penetrate the blood–brain barrier (BBB), a significant challenge in developing neurodegenerative treatments. This capability makes small molecules particularly attractive for targeting brain-associated diseases such as Alzheimer's.25 The initial breakthrough in small molecule inhibitors of amyloid formation was inspired by the discovery that Congo red and Thioflavin-T (ThT) could effectively block amyloid assembly in various proteins, including Aβ40/42 and IAPP.26,27 Up to now, a wide range of small molecule inhibitors of amyloid aggregates, including polyphenols, have been reported.28–31 However, a notable disadvantage of polyphenol compounds is their lack of specificity as aggregation inhibitors,32,33 which hinders their progression to clinical trials.
One potential method for preventing Aβ oligomerization leverages the precise targeting abilities of antibodies. By designing antibodies that bind selectively to monomeric amyloid precursors, the formation of oligomeric structures can be halted. This inhibition of Aβ aggregation has shown considerable promise in reducing neurotoxic effects and slowing the advancement of Alzheimer's disease.34–36 However these antibodies could have nonspecific binding with some antigens, leading to potential adverse effects.37 Aducanumab, lecanemab, and donanemab are monoclonal antibodies recently approved for AD treatment. They target Aβ aggregates, aiming to mitigate AD progression. Among these, aducanumab selectively binds to Aβ aggregated species, demonstrating significant reduction in amyloid plaques.38 Lecanemab would bind selectively to soluble Aβ protofibrils and aggregation intermediates, promoting the clearance of protofibrils.39 Donanemab binds specifically to N-terminal pyroglutamate Aβ, which is prevalent in deposited plaques, aiming to slow down disease progression.40 Amyloid-related imaging abnormalities (ARIAs) are common adverse effects of amyloid-clearing therapies, particularly monoclonal antibodies like aducanumab, lecanemab, and donanemab.41 The two main types are ARIA-E and ARIA-H, where ARIA-E is suggestive of vasogenic edema or sulcal effusion, caused by fluid leakage into the brain parenchyma, and ARIA-H is suggestive of microhemorrhages or superficial siderosis, resulting from vascular damage and deposition of blood breakdown products.42 After amyloid-clearing antibodies target Aβ within cerebral vessels, the vessel walls might be destabilized and more permeable, leading to leakage of fluid into the brain parenchyma, microvascular damage and bleeding.43 The binding of monoclonal antibodies to Aβ deposits might also trigger an immune response, leading to local inflammation in the blood vessel walls. Such an inflammatory response exacerbates endothelial dysfunction and contributes to ARIA-E and ARIA-H. Besides, the amyloid removal could disrupt the structural integrity of blood vessels and the blood–brain barrier, leadind to fluid leakage and hemosiderin deposition.40,44,45
Molecular chaperones have been employed as another approach to counteract amyloid formation. Among them, the BRICHOS chaperone has been shown to inhibit Aβ oligomerization by specifically interacting with the second-nucleation region, a key site responsible for generating neurotoxic oligomeric species.46 A solvent-exposed β-strand spanning residues 26–28 on Aβ42 was specifically recognized by a Bri2 BRICHOS variant. Notably, even at a low BRICHOS ratio, fibril-catalyzed nucleation was effectively blocked, indicating that the recognized site serves as a catalytic aggregation hotspot.47 The success of BRICHOS underscores the crucial role of chaperones in mitigating amyloid aggregation-induced toxicity by specifically targeting the surface of amyloid fibrils, thereby disrupting the catalytic cycles involved in aggregate formation. Building on this, researchers discovered that heat shock protein 70 (Hsp70) also inhibits Aβ fibril growth by blocking the fibril ends.48 Additionally, other chaperone systems have been identified as effective anti-aggregation agents,49,50 making it possible to reverse amyloid fibrils despite the thermostability of those rigid β-sheet-rich structures.51,52 Taken together, these findings highlight a promising future for the use of natural or engineered proteins in combating aggregation-induced diseases, while also offering valuable insights into the underlying mechanisms of aggregation-related pathologies.
Synthetic β-sheet mimics have been widely studied for their ability to inhibit amyloid formation by specifically recognizing amyloid peptide species.53–55 The inhibition mechanism depends on the high specificity and strong affinity for the aggregation core region, which would otherwise progress into oligomers and fibrils.53,56–59 Using a similar approach, incorporating N-methyl or N-amino modified amino acids into the core sequence enables precise hydrogen bonding with the target regions while preventing further amyloid peptide oligomerization.60,61
Hamilton and colleagues recently developed synthetic foldamers as inhibitors of amyloid formation in Aβ42, IAPP, and other proteins.62–66 Foldamers adopt distinct conformations due to interactions between their subunits. By varying the backbone and functional groups, foldamers can access a wide range of molecular architectures, allowing them to interact with numerous biomolecules in a structure- and sequence-specific manner, making foldamers highly promising for the discovery of novel agents in biomolecular targeting.
This brief review highlights a promising approach for inhibiting Aβ oligomerization, which involves unnatural foldamers that interact with Aβ to prevent further aggregation (Fig. 1). This strategy has been shown to redirect Aβ into nonamyloid structures, thus preventing Aβ neurotoxic oligomeric formation. As mentioned above about the nearly identical secondary structures of Aβ40 and Aβ42 under solution conditions like SDS or HFIP, the inhibition strategy present in the review is applicable to both Aβ40 and Aβ42.
 |
| Fig. 1 The schematic illustration of the helical Aβ peptide stabilized by the foldamer. | |
Oligoquinoline-based foldamer attenuates Aβ oligomerization and cytotoxicity
Foldamers are molecules synthesized to replicate the structural and functional properties of natural polymers, such as proteins, polysaccharides, and nucleic acids.67–70 These structures are usually stabilized through non-covalent interactions. Despite significant advancements in foldamer research, recent studies have focused on those employing amide bonds to form intramolecular hydrogen bonds that constitute their structural frameworks.64,71,72 As a class of foldamers, oligoarylamides can adopt diverse secondary structures, enabling interactions with various biomolecules, notably in preventing the aggregation of human islet amyloid polypeptide (IAPP).73–76 Particularly, Hamilton's group has leveraged the quinoline framework to modulate Aβ aggregation and its related cytotoxicity.
NMR-based investigation into the structure of Aβ40 revealed several key domains within the peptide, primarily between residues His13 and Val24.8 Given the well-established role of the 16KLVFF20 region in initiating Aβ aggregation, the researchers synthesized a library of oligoquinolines comprising four subunits, aiming to disrupt and stabilize the central region through protein–protein interactions (Fig. 2a). Among these compounds, molecule 5 emerged as the most potent antagonist of Aβ aggregation, completely suppressing aggregation at an equimolar ratio. Structure–activity relationship (SAR) studies demonstrated that quinoline 5 binds specifically to the central region of Aβ in a sequence-dependent manner, emphasizing the critical role of foldamer side chains in inhibiting Aβ aggregation. Following the identification of the lead compound, the researchers explored whether compound 5 induced an α-helical conformation in Aβ. Typically, Aβ transitions from a random coil to β-sheet structures, however, in the presence of compound 5, as observed in Fig. 2f and g, the double minimum peaks were detected at 208 nm and 222 nm, indicating that it adopted and maintained an α-helical conformation throughout the assay. A 2D NMR spectrum was utilized to identify the binding regions between compound 5 and Aβ, (Fig. 2h) revealing significant chemical shifts in residues from His13 to Val24, particularly in His13–Lys16 and Leu17–Phe20 segments, which delineate the binding region of quinoline 5. Biophysical assays, including negative staining transmission electron microscopy (TEM) and enzyme-linked immunosorbent assay (ELISA), confirmed the effectiveness of compound 5 in inhibiting Aβ oligomerization in vitro. Subsequently, the researchers assessed whether compound 5 could mitigate Aβ aggregation-induced cytotoxicity by preventing its aggregation. Using the N2a cell line, cell viability improved significantly from 45% to 89% in the presence of compound 5. Confocal assays further showed that compound 5 co-translocated with Aβ to the mitochondria, indicating strong binding affinity even under cellular conditions.
 |
| Fig. 2 (a) Generic scheme for the chemical structures of quinoline foldamers. (b) Chemical structure of quinoline 5. (c) 3D model of quinoline 5.77 (d) The partially folded NMR structure of Aβ40 used to highlight the binding region of quinoline 5 (turquoise labeled). (e) Top view of the oligoquinoline foldamer. (f) Circular dichroism of 20 μM Aβ40 at 0 h and 24 h. (g) Circular dichroism of Aβ40 at multiple time points in the presence of an equimolar amount of 5. (h) Overlay of the 1H–15N HSQC NMR spectrum of 60 μM Aβ40 in the absence and presence of 5(15N-Aβ40 : 5 = 1 : 2). | |
In summary, the authors successfully optimized compound 5, which interacted with Aβ with high specificity, as confirmed by both biophysical and cell-based assays. Presumably, there have been multiple interactions between compound 5 and the Aβ peptide, including salt bridges, hydrophobic interactions, and other non-covalent forces.
Oligopyridylamide-based α-helical mimetics modulated Aβ self-oligomerization
Hamilton and colleagues have also explored the potential of a pyridylcarboxamide scaffold as an inhibitor of Aβ aggregation (Fig. 3).78 Through library screening, two lead candidates, ADH-31 (Fig. 3a) and ADH-41 (Fig. 3b), were identified as effective inhibitors. These compounds exhibited strong antagonistic activity against Aβ aggregation, potentially targeting distinct regions. Subdomain 1 consists of a positively charged region (His13–His14–Lys16) and a hydrophobic segment (Leu17–Val18–Phe19–Phe20), while subdomain 2 encompasses a negatively charged region (Glu22–Asp23) alongside a hydrophobic area (Leu17–Val18–Phe19–Phe20). To interact directly with domain 1, the authors designed oligopyridylamides featuring hydrophobic groups and negatively charged side chains.65 It was discovered that Aβ aggregation was fully inhibited at an equimolar concentration of ADH-31. A variety of biophysical and biochemical techniques, including Dot blot, circular dichroism (CD), TEM, 2D NMR, and cell-based assays, were employed to evaluate ADH-31's bioactivity. The results indicated that ADH-31 likely binds to subdomain 1, stabilizing a partial helix conformation of the peptide, aligning with the initial objective of developing agents to stabilize the central region of Aβ.
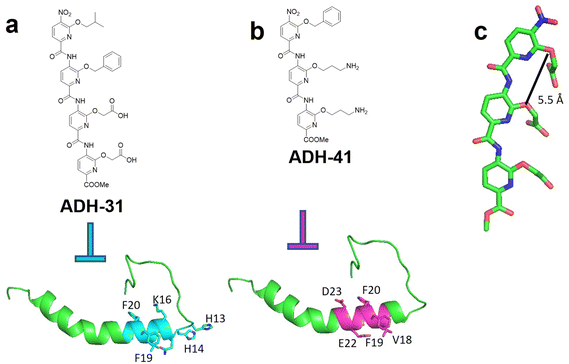 |
| Fig. 3 (a) Chemical structure of ADH-31 and the potential binding region from Aβ with side chains being labeled. (b) Chemical structure of ADH-41 and its potential binding region from Aβ with side chains being labeled. (c) Trispyridylamide scaffold.79 | |
Conversely, the positively charged tripyridylamide ADH-41 was specifically designed to target domain 2 and was identified as a highly effective inhibitor of Aβ aggregation.64 Unlike ADH-31, ADH-41 was shown, through 2D NMR studies, to interact with subdomain 2. This domain contains two negatively charged residues, Glu22 and Asp23, forming a central negatively charged region. The negatively charged region composing Glu22 and Asp23, through forming toxic turn, has been shown to play a critical role in Aβ42-mediated cytotoxicity. In addition, multiple mutation sites related to familiar Alzheimer's disease are distributed at Glu22 and Asp23, indicating the important role of this region in AD development.21,22,80,81 Accordingly, by incorporating primary amines, ADH-41 could form electrostatic interactions with Glu22 and Asp23, while also engaging in hydrophobic–hydrophobic interactions with adjacent hydrophobic residues. These combined interactions facilitated ADH-41 efficient inhibition of Aβ oligomerization.
Helical γ-peptide foldamers trap the monomeric Aβ42 peptide
Over the last few years, researchers have developed a class of constrained heterocyclic γ-amino acids, composed of 4-amino(methyl)-1,3-thiazole-5-carboxylic acids, termed ATC. ATC foldamers would adopt a 9-helix scaffold resembling a 310 helix, with a helical radius being 2.0 Å and helical pitch reaching 12.0 Å. The backbone exhibits low dependency on the nature of side chains. The projection of residues in the ATC foldamer is rigid and predictable,82 rendering ATC an ideal scaffold for interactions with potential biomolecules through PPIs. The 310 helix has been proposed to be an intermediate in Aβ transition from the α-helix to β-sheet. Consequently, researchers selectively chose the side chains to target the transient helix domain within Aβ, preventing its conversion into cross-β-sheet rich structures.
The researchers prepared two series of compounds for inhibition testing. Hydrophobic residues, including isobutyl and benzyl groups, were introduced to form a hydrophobic interaction specifically with amyloid sequence 16KLVFF20. Cationic aminobutyl substituents were proposed to interact with negatively charged residues of Aβ42 (Asp1, Glu3, Asp7. Glu11, Glu22, Asp23 and A42). NMR and circular dichroism (CD) analyses corroborated the C9 helical pattern model. Ultimately, compound 6 was identified as completely inhibiting Aβ42 aggregation at a 10
:
1 ratio.83 Additionally, compound 6 demonstrated effectiveness in hIAPP inhibition, reducing the maximum fluorescence intensity to 40% of the control group in which no inhibitors were added. Multiple assays, including TEM, mass spectrometry, and capillary electrophoresis, supported the efficacy of foldamer 6 as a potential inhibitor of Aβ aggregation (Fig. 4).
 |
| Fig. 4 (a) Chemical structures of ATC foldamers showing the organization of thiazole residues and γ-side chains. (b) Side view and top view of the ATC foldamer single crystal structure.84 (c) Chemical structure of compound 6. | |
Sulfonyl-γ-AApeptides inhibit Aβ42 aggregation
Our group has focused on the development of a novel class of foldamers termed sulfonyl-γ-AApeptides over the past decade. Single crystal structures indicated that such a foldamer adopts a rigid 414-helix scaffold with minimum dependence on the nature of side chains. Unlike the natural helix domain, both sulfonamido moieties' structure and intramolecular hydrogen bonds confer robust helical stability of the foldamers, as well as the proteolytic resistance and high diversity for potential pharmaceutical and material applications.85–88 Thus, it was speculated that stabilizing the partial helix domain in Aβ through recognition of its surface could prevent Aβ aggregation.
By closely examining the α-helical structure of Aβ40 (PDB: 1BA4), we identified three potential domains that could be crucial for designing ligands to recognize and stabilize the Aβ helix: cationic domain 1, comprising primarily positively charged or polar residues (H13, H14, Q15, and K16); hydrophobic domain 2, containing the aggregation-prone hydrophobic fragment (L17, V18, F19, F20, and A21); and anionic domain 3, composed of negatively charged residues (E22 and D23). We hypothesized that ligands with complementary hydrophobic and charged groups capable of simultaneously targeting multiple domains of the Aβ helix could bind and stabilize it through both hydrophobic and electrostatic interactions.
To investigate this, we designed a series of sulfonyl-γ-AApeptides, based on the helical folding pattern of sulfonyl-γ-AApeptides, incorporating a variety of side functional groups, and assessed their potential to target the helical structure of Aβ. After several rounds of optimizations, we identified lead compound Ab-6 as the most potent inhibitor of Aβ aggregation.89 Thioflavin T (ThT) assays revealed no visible fibril positive signal, corroborated by TEM analysis and dynamic light scattering (DLS) results. Circular dichroism (CD) and NMR spectra indicated that Ab-6 effectively induced the helix subdomain within Aβ, with pronounced shifts in the targeted residue regions. Cell based assays demonstrated that Ab-6 could interact with Aβ under cellular conditions and co-localize onto the mitochondrial membrane (Fig. 5).
 |
| Fig. 5 (a) Chemical structures of sulfonyl-γ-AApeptides: “a” and “b” denote the chiral and sulfonyl side chains. (b) Side and top views of sulfonyl-γ-AApeptides. (c) Chemical structure of Ab-6. (d) Helical wheel of sulfonyl-γ-AApeptide illustrating side chain distribution. (e) and (f) Hypothesized interactions between Ab-6 and Aβ side chains. | |
Conclusion
The accumulation of protein aggregations species, characterized as the histopathological signature of amyloid diseases, results from the formation of cross-β-sheet amyloid fibril deposits both intracellularly and extracellularly, has become a defining feature of various brain disorders.90–98 Consequently, the accumulation of these protein aggregations has been proposed as the driving force in their pathogenesis. Therapeutic options targeting different stages in neurodegenerative diseases, such as protein production, chaperone-mediated refolding, and degradation via autophagy or a proteasome pathway, are under extensive investigation. Apart from recently FDA-approved monoclonal antibodies, like aducanumab, lecanemab, and donanemab, research into other therapeutic agents for Alzheimer's disease is continuing robustly. As of January 2024, the Alzheimer's disease drug development pipeline included 164 clinical trials assessing 127 unique drugs. Of these, 48 trials were in Phase 3, evaluating 32 drugs; 90 trials were in Phase 2, assessing 81 drugs; and 26 trials were in Phase 1, testing 25 agents. These trials encompass a range of therapeutic approaches, including disease-modifying biologics and small molecules, cognitive enhancers, and treatments targeting neuropsychiatric symptoms.99 This work will focus on the highlights of the recent development of de novo foldamers as antagonists of Aβ aggregation through recognition and stabilization of the Aβ helix domain.
Employing diverse scaffolds to interfere with proteins has been utilized across various disciplines, and the modulation of the structure and function of amyloid proteins through artificial foldamers represents a recent innovation. Over the past few years, Hamilton and their colleagues have pioneered approaches elucidating pathways for designing potent inhibitors of Aβ and IAPP. By employing different scaffolds, the researchers successfully identified oligoquinoline and oligopyridylamide-based foldamers as effective antagonists toward Aβ oligomerization. The stabilization of helix domains by foldamers occurs through various interactions, including electrostatic interaction, hydrogen bonding, hydrophobic effects, and some other forces. The Ongeri group has concentrated on the development and potential application of de novo γ-peptides. By recognizing the advantageous effect of cationic amines in antagonizing Aβ amyloidogenesis independently of Hamilton's group, they identified lead compounds as Aβ inhibitors. By leveraging these previous reports on de novo foldamers' design principles for inhibiting Aβ amyloidogenesis, along with the unique characteristics of sulfonyl-γ-AApeptides, our group has also optimized lead compound Ab-6 as an Aβ antagonist. CD spectra indicated that the helix conformation was induced within Aβ throughout the assays, while 2D NMR spectra confirmed the conformation change and potential binding regions. Cell-based assays provided insight into how these foldamers enhance cell viability by binding to Aβ in a cellular environment.
As suggested by Teplow et al., certain strategies may lead to ideal therapeutics agents for targeting Aβ oligomerization.100 One approach focuses on modulating the conformational arrangement of the monomeric Aβ to influence its downstream functions and prevent aggregation, while another seeks to destabilize oligomeric species to inhibit the fibril growth. Foldamer-based intervention in Aβ oligomerization is particularly promising from both perspectives, as misfolded Aβ peptides may either be rearranged into partially helix conformation or their oligomerization can be inhibited, thereby reducing toxicities. This work highlights several recent studies employing the former strategy, wherein researchers utilized foldamers to modulate Aβ oligomerization into Aβ helical forms. Overall, foldamers hold considerable promise in advancing our understanding and development of therapeutic agents for the treatment of Alzheimer's disease.
Data availability
No primary research data have been included or analyzed in this review.
Conflicts of interest
There are no conflicts to declare.
Acknowledgements
This work was supported by NIH 2R01AG056569-06 and 1R01GM150196-01.
References
- T. P. Knowles, M. Vendruscolo and C. M. Dobson, Nat. Rev. Mol. Cell Biol., 2014, 15, 384–396 CrossRef CAS PubMed.
- M. G. Iadanza, M. P. Jackson, E. W. Hewitt, N. A. Ranson and S. E. Radford, Nat. Rev. Mol. Cell Biol., 2018, 19, 755–773 CrossRef CAS PubMed.
- K. Lundmark, G. T. Westermark, S. Nystrom, C. L. Murphy, A. Solomon and P. Westermark, Proc. Natl. Acad. Sci. U. S. A., 2002, 99, 6979–6984 CrossRef CAS PubMed.
- K. Lundmark, G. T. Westermark, A. Olsen and P. Westermark, Proc. Natl. Acad. Sci. U. S. A., 2005, 102, 6098–6102 CrossRef CAS PubMed.
- D. J. Selkoe and J. Hardy, EMBO Mol. Med., 2016, 8, 595–608 CrossRef CAS PubMed.
- R. Riek, P. Guntert, H. Dobeli, B. Wipf and K. Wuthrich, Eur. J. Biochem., 2001, 268, 5930–5936 CrossRef CAS PubMed.
- J. Jarvet, J. Danielsson, P. Damberg, M. Oleszczuk and A. Graslund, J. Biomol. NMR, 2007, 39, 63–72 CrossRef CAS PubMed.
- M. Coles, W. Bicknell, A. A. Watson, D. P. Fairlie and D. J. Craik, Biochemistry, 1998, 37, 11064–11077 CrossRef CAS PubMed.
- L. C. Serpell, Biochim. Biophys. Acta., 2000, 1502, 16–30 CrossRef CAS PubMed.
- C. L. Masters, R. Bateman, K. Blennow, C. C. Rowe, R. A. Sperling and J. L. Cummings, Nat. Rev. Dis. Primers, 2015, 1, 15056 CrossRef PubMed.
- Y. Yan and C. Wang, J. Mol. Biol., 2006, 364, 853–862 CrossRef CAS PubMed.
- D. M. Walsh and D. J. Selkoe, J. Neurochem., 2007, 101, 1172–1184 CrossRef CAS PubMed.
- J. T. Jarrett, E. P. Berger and P. T. Lansbury, Jr., Biochemistry, 1993, 32, 4693–4697 CrossRef CAS PubMed.
- R. Kayed, E. Head, J. L. Thompson, T. M. McIntire, S. C. Milton, C. W. Cotman and C. G. Glabe, Science, 2003, 300, 486–489 CrossRef CAS PubMed.
- J. Hardy and D. J. Selkoe, Science, 2002, 297, 353–356 CrossRef CAS PubMed.
- G. M. Shankar, S. Li, T. H. Mehta, A. Garcia-Munoz, N. E. Shepardson, I. Smith, F. M. Brett, M. A. Farrell, M. J. Rowan, C. A. Lemere, C. M. Regan, D. M. Walsh, B. L. Sabatini and D. J. Selkoe, Nat. Med., 2008, 14, 837–842 CrossRef CAS PubMed.
- A. Chikugo, Y. Irie, C. Tsukano, A. Uchino, T. Maki, T. Kume, T. Kawase, K. Hirose, Y. Kageyama, I. Tooyama and K. Irie, ACS Chem. Neurosci., 2022, 13, 2913–2923 CrossRef CAS PubMed.
- D. M. Walsh, I. Klyubin, J. V. Fadeeva, W. K. Cullen, R. Anwyl, M. S. Wolfe, M. J. Rowan and D. J. Selkoe, Nature, 2002, 416, 535–539 CrossRef CAS PubMed.
- M. Townsend, G. M. Shankar, T. Mehta, D. M. Walsh and D. J. Selkoe, J. Physiol., 2006, 572, 477–492 CrossRef CAS PubMed.
- A. R. Foley, H. W. Lee and J. A. Raskatov, J. Org. Chem., 2020, 85, 1385–1391 CrossRef CAS PubMed.
- K. Irie, Biosci., Biotechnol., Biochem., 2020, 84, 1–16 CrossRef CAS PubMed.
- Y. Masuda, S. Uemura, R. Ohashi, A. Nakanishi, K. Takegoshi, T. Shimizu, T. Shirasawa and K. Irie, ChemBioChem, 2009, 10, 287–295 CrossRef CAS PubMed.
- N. Arispe, H. B. Pollard and E. Rojas, Proc. Natl. Acad. Sci. U. S. A., 1993, 90, 10573–10577 CrossRef CAS PubMed.
- V. L. Villemagne, S. Burnham, P. Bourgeat, B. Brown, K. A. Ellis, O. Salvado, C. Szoeke, S. L. Macaulay, R. Martins, P. Maruff, D. Ames, C. C. Rowe and C. L. Masters, AIBL Research Group, Lancet Neurol., 2013, 12, 357–367 CrossRef CAS PubMed.
- A. J. Doig and P. Derreumaux, Curr. Opin. Struct. Biol., 2015, 30, 50–56 CrossRef CAS PubMed.
- Y. Porat, A. Abramowitz and E. Gazit, Chem. Biol. Drug Des., 2006, 67, 27–37 CrossRef CAS PubMed.
- V. M. Lee, Neurobiol. Aging, 2002, 23, 1039–1042 CrossRef CAS PubMed.
- B. Cheng, H. Gong, H. Xiao, R. B. Petersen, L. Zheng and K. Huang, Biochim. Biophys. Acta., 2013, 1830, 4860–4871 CrossRef CAS PubMed.
- N. Ferreira, M. J. Saraiva and M. R. Almeida, FEBS Lett., 2011, 585, 2424–2430 CrossRef CAS PubMed.
- L. M. Young, P. Cao, D. P. Raleigh, A. E. Ashcroft and S. E. Radford, J. Am. Chem. Soc., 2014, 136, 660–670 CrossRef CAS PubMed.
- J. Bieschke, J. Russ, R. P. Friedrich, D. E. Ehrnhoefer, H. Wobst, K. Neugebauer and E. E. Wanker, Proc. Natl. Acad. Sci. U. S. A., 2010, 107, 7710–7715 CrossRef CAS PubMed.
- F. Chiti and C. M. Dobson, Annu. Rev. Biochem., 2006, 75, 333–366 CrossRef CAS PubMed.
- K. K. Turoverov, I. M. Kuznetsova and V. N. Uversky, Prog. Biophys. Mol. Biol., 2010, 102, 73–84 CrossRef CAS PubMed.
- J. Liu, B. Yang, J. Ke, W. Li and W. C. Suen, Drugs Aging, 2016, 33, 685–697 CrossRef CAS PubMed.
- E. Karran and J. Hardy, Ann. Neurol., 2014, 76, 185–205 CrossRef CAS PubMed.
- P. Lichtlen and M. H. Mohajeri, J. Neurochem., 2008, 104, 859–874 CrossRef CAS PubMed.
- G. A. Kerchner and A. L. Boxer, Expert Opin. Biol. Ther., 2010, 10, 1121–1130 CrossRef CAS PubMed.
- J. Sevigny, P. Chiao, T. Bussiere, P. H. Weinreb, L. Williams, M. Maier, R. Dunstan, S. Salloway, T. Chen, Y. Ling, J. O’Gorman, F. Qian, M. Arastu, M. Li, S. Chollate, M. S. Brennan, O. Quintero-Monzon, R. H. Scannevin, H. M. Arnold, T. Engber, K. Rhodes, J. Ferrero, Y. Hang, A. Mikulskis, J. Grimm, C. Hock, R. M. Nitsch and A. Sandrock, Nature, 2016, 537, 50–56 CrossRef CAS PubMed.
- C. H. van Dyck, C. J. Swanson, P. Aisen, R. J. Bateman, C. Chen, M. Gee, M. Kanekiyo, D. Li, L. Reyderman, S. Cohen, L. Froelich, S. Katayama, M. Sabbagh, B. Vellas, D. Watson, S. Dhadda, M. Irizarry, L. D. Kramer and T. Iwatsubo, N. Engl. J. Med., 2023, 388, 9–21 CrossRef CAS PubMed.
- M. A. Mintun, A. C. Lo, C. Duggan Evans, A. M. Wessels, P. A. Ardayfio, S. W. Andersen, S. Shcherbinin, J. Sparks, J. R. Sims, M. Brys, L. G. Apostolova, S. P. Salloway and D. M. Skovronsky, N. Engl. J. Med., 2021, 384, 1691–1704 CrossRef CAS PubMed.
- R. L. Gottlieb, A. Nirula, P. Chen, J. Boscia, B. Heller, J. Morris, G. Huhn, J. Cardona, B. Mocherla, V. Stosor, I. Shawa, P. Kumar, A. C. Adams, J. Van Naarden, K. L. Custer, M. Durante, G. Oakley, A. E. Schade, T. R. Holzer, P. J. Ebert, R. E. Higgs, N. L. Kallewaard, J. Sabo, D. R. Patel, P. Klekotka, L. Shen and D. M. Skovronsky, JAMA, 2021, 325, 632–644 CrossRef CAS PubMed.
- A. Krzyzanowska, I. Garcia-Consuegra, C. Pascual, D. Antequera, I. Ferrer and E. Carro, J. Neuropathol. Exp. Neurol., 2015, 74, 359–369 CrossRef CAS PubMed.
- S. M. Greenberg, B. J. Bacskai, M. Hernandez-Guillamon, J. Pruzin, R. Sperling and S. J. van Veluw, Nat. Rev. Neurol., 2020, 16, 30–42 CrossRef CAS PubMed.
- S. Salloway, S. Chalkias, F. Barkhof, P. Burkett, J. Barakos, D. Purcell, J. Suhy, F. Forrestal, Y. Tian, K. Umans, G. Wang, P. Singhal, S. Budd Haeberlein and K. Smirnakis, JAMA Neurol., 2022, 79, 13–21 CrossRef PubMed.
- N. Suzuki, T. Hatta, M. Ito and K. I. Kusakabe, Chem. Pharm. Bull., 2024, 72, 602–609 CrossRef CAS PubMed.
- S. I. A. Cohen, P. Arosio, J. Presto, F. R. Kurudenkandy, H. Biverstal, L. Dolfe, C. Dunning, X. Yang, B. Frohm, M. Vendruscolo, J. Johansson, C. M. Dobson, A. Fisahn, T. P. J. Knowles and S. Linse, Nat. Struct. Mol. Biol., 2015, 22, 207–213 CrossRef CAS PubMed.
- R. Kumar, T. Le Marchand, L. Adam, R. Bobrovs, G. Chen, J. Fridmanis, N. Kronqvist, H. Biverstal, K. Jaudzems, J. Johansson, G. Pintacuda and A. Abelein, Nat. Commun., 2024, 15, 965 CrossRef CAS PubMed.
- P. Arosio, T. C. Michaels, S. Linse, C. Mansson, C. Emanuelsson, J. Presto, J. Johansson, M. Vendruscolo, C. M. Dobson and T. P. Knowles, Nat. Commun., 2016, 7, 10948 CrossRef CAS PubMed.
- N. B. Nillegoda, J. Kirstein, A. Szlachcic, M. Berynskyy, A. Stank, F. Stengel, K. Arnsburg, X. Gao, A. Scior, R. Aebersold, D. L. Guilbride, R. C. Wade, R. I. Morimoto, M. P. Mayer and B. Bukau, Nature, 2015, 524, 247–251 CrossRef CAS PubMed.
- A. Mogk, E. Kummer and B. Bukau, Front. Mol. Biosci., 2015, 2, 22 Search PubMed.
- A. J. Baldwin, T. P. Knowles, G. G. Tartaglia, A. W. Fitzpatrick, G. L. Devlin, S. L. Shammas, C. A. Waudby, M. F. Mossuto, S. Meehan, S. L. Gras, J. Christodoulou, S. J. Anthony-Cahill, P. D. Barker, M. Vendruscolo and C. M. Dobson, J. Am. Chem. Soc., 2011, 133, 14160–14163 CrossRef CAS PubMed.
- T. R. Jahn and S. E. Radford, Arch. Biochem. Biophys., 2008, 469, 100–117 CrossRef CAS PubMed.
- S. A. Sievers, J. Karanicolas, H. W. Chang, A. Zhao, L. Jiang, O. Zirafi, J. T. Stevens, J. Munch, D. Baker and D. Eisenberg, Nature, 2011, 475, 96–100 CrossRef CAS PubMed.
- E. Andreetto, E. Malideli, L. M. Yan, M. Kracklauer, K. Farbiarz, M. Tatarek-Nossol, G. Rammes, E. Prade, T. Neumuller, A. Caporale, A. Spanopoulou, M. Bakou, B. Reif and A. Kapurniotu, Angew. Chem., Int. Ed., 2015, 54, 13095–13100 CrossRef CAS PubMed.
- L. M. Yan, A. Velkova, M. Tatarek-Nossol, G. Rammes, A. Sibaev, E. Andreetto, M. Kracklauer, M. Bakou, E. Malideli, B. Goke, J. Schirra, M. Storr and A. Kapurniotu, Angew. Chem., Int. Ed., 2013, 52, 10378–10383 CrossRef CAS PubMed.
- P. N. Cheng, C. Liu, M. Zhao, D. Eisenberg and J. S. Nowick, Nat. Chem., 2012, 4, 927–933 CrossRef CAS PubMed.
- P. N. Cheng, R. Spencer, R. J. Woods, C. G. Glabe and J. S. Nowick, J. Am. Chem. Soc., 2012, 134, 14179–14184 CrossRef CAS PubMed.
- I. L. Karle, C. Das and P. Balaram, Proc. Natl. Acad. Sci. U. S. A., 2000, 97, 3034–3037 CrossRef CAS PubMed.
- J. F. Espinosa and S. H. Gellman, Angew. Chem., Int. Ed., 2000, 39, 2330–2333 CrossRef CAS.
- X. L. Bu, P. P. N. Rao and Y. J. Wang, Mol. Neurobiol., 2016, 53, 3565–3575 CrossRef CAS PubMed.
- K. C. Tillett and J. R. Del Valle, RSC Adv., 2020, 10, 14331–14336 RSC.
- S. Kumar, M. Birol, D. E. Schlamadinger, S. P. Wojcik, E. Rhoades and A. D. Miranker, Nat. Commun., 2016, 7, 11412 CrossRef CAS PubMed.
- M. Birol, S. Kumar, E. Rhoades and A. D. Miranker, Nat. Commun., 2018, 9, 1312 CrossRef PubMed.
- S. Kumar and A. D. Hamilton, J. Am. Chem. Soc., 2017, 139, 5744–5755 CrossRef CAS PubMed.
- S. Kumar, A. Henning-Knechtel, M. Magzoub and A. D. Hamilton, J. Am. Chem. Soc., 2018, 140, 6562–6574 CrossRef CAS PubMed.
- S. Kumar, A. Henning-Knechtel, I. Chehade, M. Magzoub and A. D. Hamilton, J. Am. Chem. Soc., 2017, 139, 17098–17108 CrossRef CAS PubMed.
- C. M. Goodman, S. Choi, S. Shandler and W. F. DeGrado, Nat. Chem. Biol., 2007, 3, 252–262 CrossRef CAS PubMed.
- N. Chandramouli, Y. Ferrand, G. Lautrette, B. Kauffmann, C. D. Mackereth, M. Laguerre, D. Dubreuil and I. Huc, Nat. Chem., 2015, 7, 334–341 CrossRef CAS PubMed.
- G. W. Collie, K. Pulka-Ziach, C. M. Lombardo, J. Fremaux, F. Rosu, M. Decossas, L. Mauran, O. Lambert, V. Gabelica, C. D. Mackereth and G. Guichard, Nat. Chem., 2015, 7, 871–878 CrossRef CAS PubMed.
- D. J. Hill, M. J. Mio, R. B. Prince, T. S. Hughes and J. S. Moore, Chem. Rev., 2001, 101, 3893–4012 CrossRef CAS PubMed.
- B. Gong, Acc. Chem. Res., 2008, 41, 1376–1386 CrossRef CAS PubMed.
- H. Yin and A. D. Hamilton, Angew. Chem., Int. Ed., 2005, 44, 4130–4163 CrossRef CAS PubMed.
- C. J. Gerry and S. L. Schreiber, Nat. Chem. Biol., 2020, 16, 369–378 CrossRef CAS PubMed.
- S. Van Mileghem, B. Egle, P. Gilles, C. Veryser, L. Van Meervelt and W. M. De Borggraeve, Org. Biomol. Chem., 2017, 15, 373–378 RSC.
- T. L. May, J. A. Dabrowski and A. H. Hoveyda, J. Am. Chem. Soc., 2011, 133, 736–739 CrossRef CAS PubMed.
- J. A. Hebda, I. Saraogi, M. Magzoub, A. D. Hamilton and A. D. Miranker, Chem. Biol., 2009, 16, 943–950 CrossRef CAS PubMed.
- Q. Gan, C. Bao, B. Kauffmann, A. Grelard, J. Xiang, S. Liu, I. Huc and H. Jiang, Angew. Chem., Int. Ed., 2008, 47, 1715–1718 CrossRef CAS PubMed.
- I. Saraogi, J. A. Hebda, J. Becerril, L. A. Estroff, A. D. Miranker and A. D. Hamilton, Angew. Chem., Int. Ed., 2010, 49, 736–739 CrossRef CAS PubMed.
- L. A. Estroff, C. D. Incarvito and A. D. Hamilton, J. Am. Chem. Soc., 2004, 126, 2–3 CrossRef CAS PubMed.
- Y. Xiao, B. Ma, D. McElheny, S. Parthasarathy, F. Long, M. Hoshi, R. Nussinov and Y. Ishii, Nat. Struct. Mol. Biol., 2015, 22, 499–505 CrossRef CAS PubMed.
- Y. Yang, D. Arseni, W. Zhang, M. Huang, S. Lovestam, M. Schweighauser, A. Kotecha, A. G. Murzin, S. Y. Peak-Chew, J. Macdonald, I. Lavenir, H. J. Garringer, E. Gelpi, K. L. Newell, G. G. Kovacs, R. Vidal, B. Ghetti, B. Ryskeldi-Falcon, S. H. W. Scheres and M. Goedert, Science, 2022, 375, 167–172 CrossRef CAS PubMed.
- M. Simon, L. M. A. Ali, K. El Cheikh, J. Aguesseau, M. Gary-Bobo, M. Garcia, A. Morere and L. T. Maillard, Chemistry, 2018, 24, 11426–11432 CrossRef CAS PubMed.
- J. Kaffy, C. Berardet, L. Mathieu, B. Legrand, M. Taverna, F. Halgand, G. Van Der Rest, L. T. Maillard and S. Ongeri, Chemistry, 2020, 26, 14612–14622 CrossRef CAS PubMed.
- L. Mathieu, B. Legrand, C. Deng, L. Vezenkov, E. Wenger, C. Didierjean, M. Amblard, M. C. Averlant-Petit, N. Masurier, V. Lisowski, J. Martinez and L. T. Maillard, Angew. Chem., Int. Ed., 2013, 52, 6006–6010 CrossRef CAS PubMed.
- Y. Shi, G. Yin, Z. Yan, P. Sang, M. Wang, R. Brzozowski, P. Eswara, L. Wojtas, Y. Zheng, X. Li and J. Cai, J. Am. Chem. Soc., 2019, 141, 12697–12706 CrossRef CAS PubMed.
- P. Sang, Y. Shi, J. Lu, L. Chen, L. Yang, W. Borcherds, S. Abdulkadir, Q. Li, G. Daughdrill, J. Chen and J. Cai, J. Med. Chem., 2020, 63, 975–986 CrossRef CAS PubMed.
- S. Abdulkadir, C. Li, W. Jiang, X. Zhao, P. Sang, L. Wei, Y. Hu, Q. Li and J. Cai, J. Am. Chem. Soc., 2022, 144, 270–281 CrossRef CAS PubMed.
- S. Xue, W. Xu, L. Wang, X. Wang, Q. Duan, L. Calcul, S. Wang, W. Liu, X. Sun, L. Lu, S. Jiang and J. Cai, ACS Cent. Sci., 2023, 9, 1046–1058 CrossRef CAS PubMed.
- H. Liu, Y. Cui, X. Zhao, L. Wei, X. Wang, N. Shen, T. Odom, X. Li, W. Lawless, K. Karunarathne, M. Muschol, W. Guida, C. Cao, L. Ye and J. Cai, Proc. Natl. Acad. Sci. U. S. A., 2024, 121, e2311733121 CrossRef CAS.
- C. M. Dobson, Nature, 2003, 426, 884–890 CrossRef CAS PubMed.
- D. J. Selkoe, Nature, 2003, 426, 900–904 CrossRef CAS PubMed.
- C. Haass and D. J. Selkoe, Nat. Rev. Mol. Cell Biol., 2007, 8, 101–112 CrossRef CAS PubMed.
- Y. C. Wong and D. Krainc, Nat. Med., 2017, 23, 1–13 CrossRef CAS PubMed.
- S. Horowitz, P. Koldewey, F. Stull and J. C. Bardwell, Curr. Opin. Struct. Biol., 2018, 48, 1–5 CrossRef CAS PubMed.
- A. Mukherjee, D. Morales-Scheihing, P. C. Butler and C. Soto, Trends Mol. Med., 2015, 21, 439–449 CrossRef CAS PubMed.
- T. Coelho, G. Merlini, C. E. Bulawa, J. A. Fleming, D. P. Judge, J. W. Kelly, M. S. Maurer, V. Plante-Bordeneuve, R. Labaudiniere, R. Mundayat, S. Riley, I. Lombardo and P. Huertas, Neurol. Ther., 2016, 5, 1–25 CrossRef PubMed.
- J. Munch, E. Rucker, L. Standker, K. Adermann, C. Goffinet, M. Schindler, S. Wildum, R. Chinnadurai, D. Rajan, A. Specht, G. Gimenez-Gallego, P. C. Sanchez, D. M. Fowler, A. Koulov, J. W. Kelly, W. Mothes, J. C. Grivel, L. Margolis, O. T. Keppler, W. G. Forssmann and F. Kirchhoff, Cell, 2007, 131, 1059–1071 CrossRef PubMed.
- J. Xu, J. Reumers, J. R. Couceiro, F. De Smet, R. Gallardo, S. Rudyak, A. Cornelis, J. Rozenski, A. Zwolinska, J. C. Marine, D. Lambrechts, Y. A. Suh, F. Rousseau and J. Schymkowitz, Nat. Chem. Biol., 2011, 7, 285–295 CrossRef CAS PubMed.
- J. Cummings, Y. Zhou, G. Lee, K. Zhong, J. Fonseca and F. Cheng, Alzheimers Dement, 2024, 10, e12465 Search PubMed.
- K. Ono, M. M. Condron and D. B. Teplow, Proc. Natl. Acad. Sci. U. S. A., 2009, 106, 14745–14750 CrossRef CAS PubMed.
|
This journal is © The Royal Society of Chemistry 2025 |
Click here to see how this site uses Cookies. View our privacy policy here.