DOI:
10.1039/D4LC00830H
(Paper)
Lab Chip, 2025,
25, 1439-1451
EV-Lev: extracellular vesicle isolation from human plasma using microfluidic magnetic levitation device†
Received
2nd October 2024
, Accepted 12th January 2025
First published on 7th February 2025
Abstract
Biological nanomaterials have unique magnetic and density characteristics that can be employed to isolate them into subpopulations. Extracellular nanovesicles (EVs) are crucial for cellular communication; however, their isolation poses significant challenges due to their diverse sizes and compositions. We present EV-Lev, a microfluidic magnetic levitation technique for high-throughput, selective isolation of small EVs (<200 nm) from human plasma. EV-Lev overcomes the challenges posed by the subtle buoyancy characteristics of EVs, whose small size and varied densities complicate traditional magnetic levitation techniques. It employs antibody-coated polymer beads of varying densities, integrating immuno-affinity and microfluidics to isolate EVs from sub-milliliter plasma volumes efficiently. It facilitates rapid, simultaneous sorting of EV subpopulations based on surface markers, such as CD9, CD63, and CD81, achieving high yield and purity. Subsequent size and morphology analyses confirmed that the isolated EVs maintain their structural integrity. EV-Lev could help uncover the cargo and function of EV subpopulations associated with multiple diseases including cancer, infectious diseases and help to discover potential biomarkers in small volume samples, while offering a portable, cost-effective, and straightforward assay scheme.
Introduction
Extracellular vesicles are a heterogeneous group of lipid bilayer vesicles (30 nm–10 μm) secreted naturally by almost all types of cells.1,2 EVs potentiate migration and proliferation of tumor cells by creating immunosuppression and chemoresistance within the tumor microenvironment.3–5 Proteins, nucleic acids, lipids, and metabolites encapsulated in EVs play an essential role for intercellular communication,6,7 rendering EVs promising candidates for next-generation drug delivery and therapeutic applications.8–10 EVs can also be a potent screening tool in liquid biopsies11,12 and could offer far-reaching implications in identifying tumor biomarkers and developing new therapies such as cancer vaccines.13–15 However, routine isolation and characterization of EVs are hampered by their heterogeneity in size and density.16,17
Various methods have been developed to isolate and classify EVs from complex biological fluids, leveraging their unique physical and biochemical characteristics (Table 1). Yet, there have been no clear definition of the best method in EV separation. The most established method, working based on physical properties, is differential ultracentrifugation.18 Ultracentrifugation separates particles by size and density through consecutive centrifugation steps. However, it is not suitable for handling small volumes of rare and precious samples.19 Another approach is the polymer precipitation method,20 which aggregates EVs using polymers based on size, followed by centrifugation to precipitate the sample. While cost-effective and efficient, this method often results in low purity as the samples are usually contaminated with proteins and other non-EV molecules.21 Size-exclusion chromatography (SEC) is yet another technique, where EVs are segregated based on size.22 In SEC, particle separation is achieved as smaller particles elute slower than larger ones, due to their ability to penetrate the column's pores and hence take a longer path. Although valuable for certain applications, SEC lacks the resolution to differentiate particles of similar sizes effectively.23 Isolation methods based on biochemical interactions, such as antigen–antibody linkage, have been widely used to selectively isolate EVs by targeting commonly expressed proteins on their surfaces.24 To date, antibody-based isolation has largely focused on isolating subtypes via magnetic beads.25 EVs are often captured by antibodies on magnetic beads which target a membrane protein family called tetraspanins (CD9, CD63, CD81, CD82, and CD151).26
Table 1 Comparison of commonly used EV isolation methods
Isolation type |
Description |
Purity |
Ref. |
Ultra-centrifugation |
Samples are centrifuged at ultra-high speeds to force separation of particles by size and density |
Dependent on additional filtration steps |
27
|
Precipitation |
Samples are incubated with buffer to decrease solubility of the EVs and then centrifuged to precipitate EVs from solution |
High possibility of contamination from other sample or cell components |
28
|
Ultra-filtration |
Sample are processed through a size-selecting semi-permeable membrane |
Contamination from protein aggregates |
29
|
Magnetic bead immunoaffinity |
Bead conjugation to EV-specific antibodies allows for high purity EV isolation. Magnets are used to separate out beads from impurities in the sample |
Highly pure |
25
|
Size exclusion chromatography |
Molecules are separated by their size through a chromatography column |
High purity |
23
|
Microfluidic technologies have opened new avenues for EV research, offering increased specificity, purity, and efficiency of isolation over conventional methods. Recent studies have achieved immunomagnetic microfluidic separation using magnetic beads that are functionalized with either a single marker (i.e., CD63,30,31 CD9 (ref. 32)) or all three markers (i.e., CD9, CD63, and CD81)33 on each bead to isolate EVs. In assays where multiplex arrays are created to detect multiple markers on a single bead, different EV subtypes are often not separately recovered after detection.32 Similarly, polymer microbeads with CD63 or EpCAM antibodies have been used to capture, trap, and elute EVs in the microfluidic channel, allowing for isolation based on one marker at a time (either CD63 or EpCAM).34
Here, we introduce a method of EV isolation based on more than one marker, using microfluidic magnetic levitation, EV-Lev (Fig. 1). EV-Lev is an immunoaffinity-based microfluidic platform capable of simultaneously separating polymer bead–EV complexes based on different markers. This approach introduces a unique dimension to bead-based immunoaffinity separation by utilizing the density-based separation capability of magnetic levitation principle. By encoding polymer beads of different densities with EV-specific antibodies, we isolated and collected EV–bead complexes through separate outlets of our platform and released the captured EV fractions from the beads in their intact form after separation.
 |
| Fig. 1 The principle of EV-Lev for EV isolation via magnetic levitation. Streptavidin beads and biotinylated antibodies for EV subpopulations are incubated to conjugate. After washing, conjugated beads are incubated overnight with filtered human plasma. Washed EV–bead compounds are flowed through the microfluidic magnetic levitation sorter (EV-Lev). The final products are isolated EVs on polymer beads which are then eluted and sent for downstream analysis such as transmission electron microscopy (TEM) and interferometric scattering (iSCAT) microscopy. | |
The novelty of our study centers on a fundamentally new concept in EV isolation that employs non-magnetic polymer beads in a magnetic levitation system. The traditional immunomagnetic separation techniques use magnets that lead magnetically active beads to aggregate without selectivity. However, in EV-Lev, the beads that are non-magnetic respond to the magnetic field based on their densities and do not aggregate and instead get sorted in line as the flow pulls them out. This approach achieves simultaneous magnetic sorting in a microfluidic channel creating a flow-based system that allows the individual beads to be sorted with their captured EVs. Such an approach has not been demonstrated before and enables multiple new dimensions as the individual beads with a specific subtype of EVs can be individually visualized and imaged on the bead surface very similar to the capabilities of flow-cytometry introduced in imaged flow channels. This represents a significant advancement over existing methods by combining the specificity of immunocapture with the precision of magnetic levitation in a flow-based system, enabling both selective isolation and characterization of EV subpopulations in a single platform. This capability potentially could open new dimensions into EV characterization that would not be possible just by magnetic immunocapture methods.
Magnetic levitation has been used to separate cells and larger particles based on density for a variety of life science and biomedical applications without requiring any labels.35–38 On the other hand, EVs, with their inherently varied densities and sizes in the nanometer range,39 present a challenge for direct magnetic levitation due to the subtle differences in their buoyancy characteristics. Magnetic levitation of EVs, in its native form, is not only challenging but also time-consuming, as it would require a significant period for the EVs to equilibrate and achieve levitation under a magnetic field due to their small sizes.38,40 This is because the small size and low mass of EVs result in a less pronounced and slower response to magnetic fields. In EV-Lev, two polymer beads, each with distinct densities, serve as floating capture surfaces for EVs. Due to their larger size (μm) compared to the EVs (nm), these beads respond to the magnetic field and form distinct levitation height profiles instantaneously depending on their densities (ESI,† page 8).41 This property allows for effective separation and collection of beads at different outlets. The surfaces of polymer beads with different densities are decorated with antibodies recognizing common tetraspanins, i.e., anti-CD9, anti-CD63, or anti-CD81. Each surface marker is paired with a bead of a specific density, and the beads are then sorted via magnetic levitation, as EV-Lev directs the beads to different flow channels simultaneously with the sample flow. Hence, the isolation of the two different bead–EV complex takes place at the same time after a single bead-mixing step where the sample is mixed with all the beads of different types. This brings a unique feature to EV isolation where multiple subtypes can be pulled simultaneously from a sub-mL of sample. After isolation with EV-Lev, eluted EVs were analyzed using interferometric scattering (iSCAT) microscopy and transmission electron microscopy (TEM), which revealed that the collected particles fall within the small EV range (<200 nm) and have preserved their structural integrity.
Identifying distinct EV subpopulations in sub-mL levels of a sample could potentially help uncover the cargo and functions of different EVs in various diseases (e.g., cancer and infectious diseases) and contribute to the discovery of potential biomarkers, particularly when sample availability is limited.
Results and discussion
Here, we developed a microfluidic magnetic levitation-based bead sorting system (Fig. 1 and S1†)—“EV-Lev”—to isolate EVs based on their protein markers. This device incorporates two permanent magnets arranged so that their identical poles face each other, and the entire assembly costs ∼$15 (Table S1†). This unique magnetic configuration creates a magnetic field gradient where the magnetic field minima are obtained in the centerline between the magnets. In EV-Lev, the diamagnetic polymer beads move from regions of high to low magnetic field intensity. Consequently, beads of two different densities, each capturing specific population of EVs based on their surface marker, levitate to distinct heights in a paramagnetic levitation medium containing gadolinium (Gd). Specifically, we used polystyrene (PS) and polymethyl methacrylate (PMMA) beads, with densities of 1.05 and 1.18 g mL−1 respectively, coated with antibodies to capture different EV subpopulations. After sorting at various outlets, captured EVs were eluted from PS and PMMA beads, respectively. Isolated EVs were then analyzed using transmission electron microscopy (TEM), nanoparticle tracking analysis (NTA), interferometric scattering (iSCAT) microscopy and total RNA assays.
Our approach offers specific advantages as the levitation of these antibody-decorated beads is instantaneous, contrasting sharply with the gradual levitation of EVs. By utilizing beads with distinct densities and attaching specific EV markers to them, we can expedite and enhance the efficiency of the sorting process, leveraging the rapid and distinct levitation characteristics of these bead–EV complexes.
Validation of EV capture on polymeric beads
We decorated the polymeric beads with different anti-CD antibodies through streptavidin–biotin interaction and used these beads as a floating assay surface when mixed with filtered human plasma. We initially employed the exosome total isolation chip (ExoTIC), as reported by Liu et al.,42 for isolating EVs from filtered human plasma. EVs that pass through a 200 nm membrane filter system with the ExoTIC chip are collected on a 50 nm membrane in PBS (Fig. 2). Following isolation, 20 μL of purified EVs were incubated with 1× solution (see Experimental: attachment of antibodies to polymer beads) of CD9, CD63, and CD81 antibodies coupled with PMMA beads (Fig. 2A). EVs captured on the PMMA beads were then eluted. Eluted EVs were subjected to iSCAT microscopy analysis to determine the size and particle concentration (Fig. 2B, S2 and S3†).
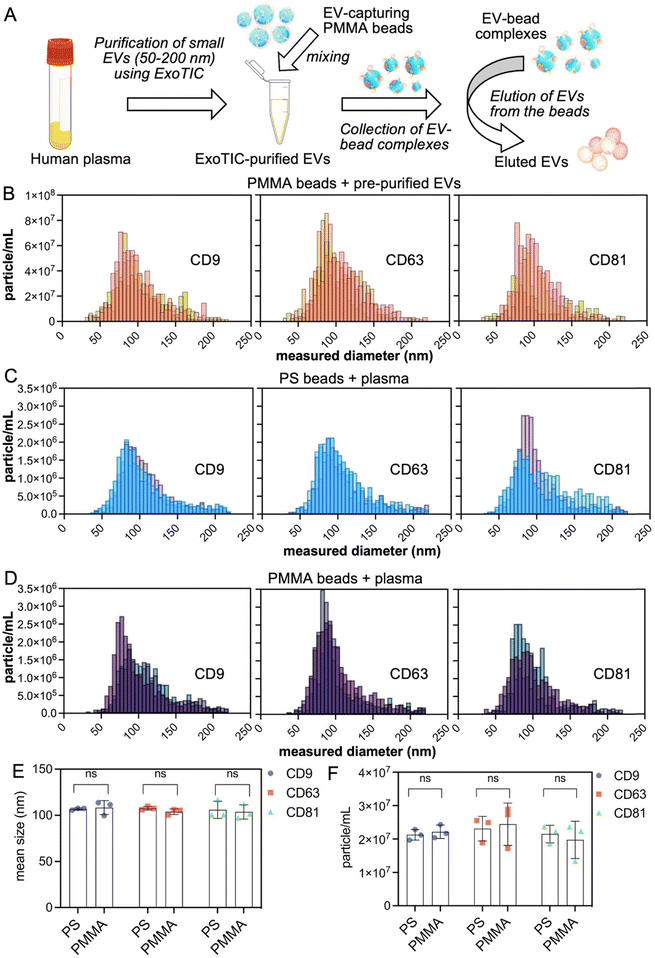 |
| Fig. 2 Characterization of bead-based assay for pre-purified EVs (A and B) and EVs captured from small volumes of plasma samples (i.e., 50 μl) (C–F). A. Illustration of EV capturing using pre-purified EVs from human plasma using PMMA-CD9, PMMA-CD63, and PMMA-CD81 beads. B. iSCAT results showing the size distribution of the pre-purified EVs after elution from PMMA-CD9, PMMA-CD63, and PMMA-CD81 beads. C. iSCAT results showing the size distribution of the plasma isolated EVs eluted from PS-CD9, PS-CD63, and PS-CD81 beads. D. iSCAT results showing the size distribution of the plasma isolated EVs eluted from PMMA-CD9, PMMA-CD63, and PMMA-CD81 beads. E. Comparison of size of plasma isolated EVs collected by different beads under three different CD markers. F. Comparison of concentration of plasma isolated EVs collected by different beads under three different CD markers. (ns: >P: 0.05). | |
iSCAT was chosen for measuring the size and number of particles in the eluted EVs due to its minimal sample volume requirement.43 20 μL of purified EVs—corresponding to 8.5 × 1010 particles per mL final concentration—were used for the EV capture experiments with PMMA-CD9, PMMA-CD63, and PMMA-CD81 beads. The PMMA-CD9, PMMA-CD63, and PMMA-CD81 beads collected 6.27 × 108 ± 1.01 × 108 particles per mL, 7.04 × 108 ± 1.93 × 108 particles per mL, and 5.24 × 108 ± 2.88 × 108 particles per mL, respectively. The size of the eluted particles were 103.27 ± 3.50 nm, 105.03 ± 9.64 nm, and 103.63 ± 4.17 nm for the PMMA-CD9, PMMA-CD63, and PMMA-CD81 beads, respectively. These findings validated that EVs can be captured on and eluted from polymer bead surfaces that are functionalized with surface markers. The theoretical maximum for EV recovery using PMMA and PS beads was also reported and discussed in the ESI,† Table S2 and page 9. After validating the EV-Lev method with purified EVs, we applied it to capture EVs directly from filtered human plasma onto the polymer beads, without prior EV purification. This approach was employed in all subsequent experiments, demonstrating the platform's capability to isolate EVs directly from complex biological samples. To characterize the isolation process from plasma, we incubated different antibody beads with a small volume (i.e., 50 μL) of filtered plasma where all the plasma came from a single healthy blood sample to avoid sample to sample variation in tetraspanin levels (Fig. 2C–F). In this regard, we used biotin anti-CD9, -CD63, and -CD81 to functionalize PS (Fig. 2C) or PMMA (Fig. 2D) beads using a 1× bead solution and 50 μL of filtered plasma. Average diameters of EVs collected by PS-CD9, PS-CD63, PS-CD81, PMMA-CD9, PMMA-CD63, and PMMA-CD81 beads were 106.80 ± 1.13 nm, 107.70 ± 1.87 nm, 105.93 ± 9.41 nm, 108.33 ± 7.55 nm, 103.87 ± 3.11 nm, 103.63 ± 7.75 nm, respectively, indicating the capture of EVs at the small size range <200 nm. The EV concentration after the elution from PS-CD9, PS-CD63, PS-CD81, PMMA-CD9, PMMA-CD63, and PMMA-CD81 beads were at 2.12 × 107 ± 1.58 × 106, 2.31 × 107 ± 3.68 × 106, 2.15 × 107 ± 2.61 × 106, 2.21 × 107 ± 2.00 × 106, 2.44 × 107 ± 6.32 × 106, and 1.98 × 107 ± 5.57 × 106 particles per mL, respectively. According to a t-test comparison (Tables S3 and S4†), the number or size of EVs collected by either PS or PMMA beads was not significantly different in terms of different CD antibodies, meaning that different polymer beads did not significantly alter the amount EVs collected from the same plasma sample (Fig. 2E and F). To show the presence of biological content in collected particles, we extracted RNA and measured total RNA concentration of EVs captured from 20 μL of filtered plasma (Fig. S4†).
We also investigated the surface of the polymeric beads using scanning electron microscopy (SEM) (Fig. 3A) after they are coupled with antibodies (i), incubated with filtered plasma afterwards (ii), and subjected to elution after the incubation with filtered plasma (iii). SEM images showed the beads had different texture in both steps of treatment (ii and iii) compared to the antibody-coated beads (i), indicating the changes due to the binding and the elution of the EVs from the bead surface. We also performed a flow cytometry (FC) analysis on EVs eluted from 1× of anti-CD9 polystyrene beads or 1× of anti-CD81 polystyrene beads incubated with a 50 μL of filtered plasma (Fig. 3B and C). In this regard, after incubation with filtered plasma, beads were co-stained with anti-CD9 and CD81 immunofluorescent antibodies as described in the Experimental section. Median fluorescent intensity values (reported in Fig. S5†) showed that EV fraction captured by PS-CD9 beads had a higher CD9 expression, compared to the EV fraction captured by PS-CD81 beads. Interestingly, EVs captured by CD9 beads also showed a significantly high CD81 expression profile. This could be attributed to the prevalence of CD9+ EVs that are highly enriched with CD81 within the given plasma sample. Overall, these results indicate that bead-based assay could fractionate EVs based on different CD expression profiles. This could potentially be leveraged for enrichment of subpopulations by pulling out the EV fractions selectively after sorting polymer beads at different outlets to perform further downstream analysis.44–46
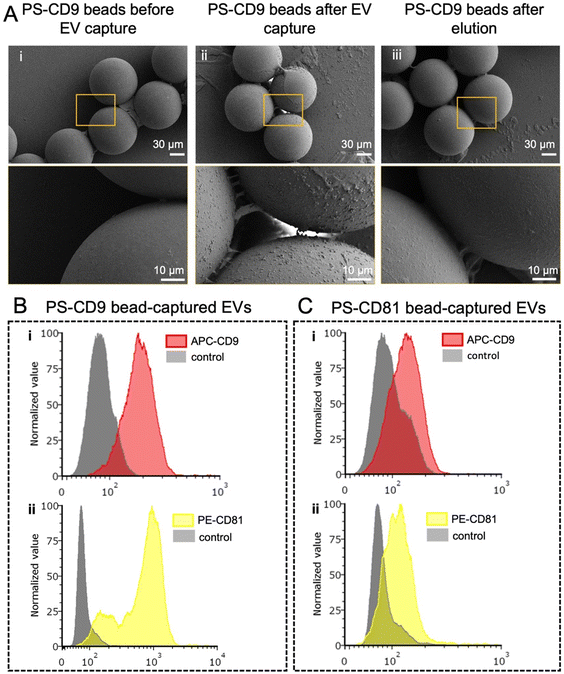 |
| Fig. 3 Characterization of the bead-based capture using SEM and FC after elution from the beads. A. Representative scanning electron microscopy (SEM) images showing the surface morphology of polystyrene (PS) beads before the capture of extracellular vesicles, after the capture of extracellular vesicles, and after the release of captured extracellular vesicles. i) PS beads conjugated with anti-CD9 antibody, ii) PS beads bound with anti-CD9 antibody and extracellular vesicles, and iii) PS beads following anti-CD9 antibody and EV conjugation and elution process, respectively. FC results for EVs eluted from beads co-stained with APC-CD9 (red-red laser) and PE-CD81 (yellow-blue laser) fluorescent dyes. B. PS-CD9 EVs under i) APC-CD9 and ii) PE-CD81. C. PMMA-CD81 EVs under i) APC-CD9 and ii) PE-CD81. | |
Magnetic levitation of non-magnetic polymer beads
For the magnetic levitation of polymer beads, a paramagnetic solution containing gadolinium ions (Gd3+) was used. Paramagnetic medium enhances the magnetic susceptibility difference between the beads and the surrounding media, hence the magnetic force acting on particles. Thus, the beads could be levitated using mild magnetic field created by permanent N52 grade magnets at the surface (∼0.3 T).41 To differentiate and quantify the number of different density beads, beads in different sizes, i.e., PS: 100 μm and PMMA: 37 μm, have been selected. To find the optimal paramagnetic agent (Gd3+) concentration for our application, we levitated a mixture of 1× PS and PMMA beads in a static magnetic levitation platform at different Gd3+ concentrations. A final concentration of 50, 75, 100, or 200 mM Gd3+ was prepared by diluting 1 M Gadavist solution with 1× PBS. Increasing concentrations of Gd3+ resulted in an increase in the levitation height for both beads (Fig. 4A–D). The average bead separation at 50 mM paramagnetic media was 317 μm, at 75 mM paramagnetic media was 220 μm, at 100 mM paramagnetic media was 165 μm, at 200 mM was paramagnetic media 78 μm (Fig. S6†). At 100 and 200 mM Gd3+, it is not feasible to separate and collect pure bead fractions at the top and bottom outlets as they levitate close to each other. At 50 mM, PMMA beads seemed to levitate closely at the bottom of the capillary. Therefore, 75 mM Gd3+ was selected as the optimal condition as the beads could be separated and levitated at the same time. The density of this optimum paramagnetic solution was found to be 1.032 g mL−1. In addition to the static levitation of PMMA and PS beads, we incubated 400 μL of filtered human plasma with a mixture of 1× mixture of PS and PMMA beads and tested their static levitation at 75 mM Gd3+. We observed that the levitation height of denser PMMA-CD63 bead was altered due to the binding to less dense PS-CD81 beads (Fig. 4E). These results indicate that EVs co-expressing different surface markers could also be, creating a sandwich assay using polymeric beads during levitation (Fig. 4F).
 |
| Fig. 4 Static magnetic levitation measurements for PS and PMMA beads at A. 50 mM, B. 75 mM, C. 100 mM, and D. 200 mM of Gd concentrations, with their respective levitation heights (μm) measured from the bottom magnet. E. Levitation of antibody-coated PS (PS-CD81) and PMMA (PMMA-CD63) beads mixed with 400 μL of human plasma and 1× bead solution. Beads in circle indicates the capture of extracellular vesicles expressing both proteins (i.e., CD81 and CD63) in a sandwich assay depicted in F. F. Schematic representation for the sandwich assay that capture extracellular vesicles expressing both CD81 and CD63 protein (scale bars: 300 μm). | |
Characterization of microfluidic isolation of EVs with EV-Lev
We then applied optimal conditions to our microfluidic capillary sorter to separate the mixture of PS and PMMA beads (Fig. 5A). The goal here was to find the highest throughput with the highest bead purity, where purity is calculated as (beads in expected channel/total beads) × 100% (Fig. S7†). The results showed a high purity rate when the ratio of bottom to top is 1 (10 μL min−1)
:
5 (50 μL min−1). The highest percentage purity (%) was 93.3 and 99.8 at the given top to bottom rates (Fig. S8 and Video S1†) with an overall throughput of 3.6 mL h−1.
 |
| Fig. 5 Characterization of EV-Lev-based microfluidic isolation of EVs. A. Representative real-time sorting of streptavidin-coated PS and PMMA beads in EV-Lev sorter. B. iSCAT and C. TEM, respectively, for EV-Lev isolated EVs. Characterization of ultracentrifuge-based isolated particles in terms of concentration and morphology using E. NTA and F. TEM, respectively. Comparison of mean D. size and G. particle concentration of EVs after EV-Lev (top and bottom channel) and UC. 450 μL of human plasma was used both in B and E. Scale bars: 200 nm. ns: >P: 0.05, *: ≤P, **: ≤0.01, ***: ≤0.001. | |
While EV capture was demonstrated with small sample volumes (e.g., 50 μL of plasma) as a proof of concept, the scalability of EV-Lev allows for processing larger sample volumes by proportionally increasing the quantity of the beads. By maintaining the same bead-to-sample ratio, the system can handle larger volumes efficiently. For instance, in our experiments (Fig. 5), we scaled up to 450 μL of plasma, which is 9 times the initial sample volume by increasing the bead count to 9× accordingly. After incubating 450 μL of filtered plasma with a mixture of PS-CD9 and PMMA-CD81 beads, the beads were subjected to sorting and elution. Sorting purity for top and bottom outlets were given in Fig. S9.† Following the elution, we quantified and determined the size of eluted particles (Fig. 5B). iSCAT results revealed that the mean size of the eluted particles was 105.3 ± 6.2 nm and 105.3 ± 6.6 nm (n = 4 spots) for top and bottom channels, respectively. EV-Lev yielded a particle concentration of 9.83 × 106 ± 4.77 × 106 particles per mL and 9.85 × 106 ± 3 × 106 particles per mL for top and bottom channels, respectively. In addition, we analyzed the EVs collected from the same amount of filtered plasma (i.e., 450 μL) using ultracentrifugation (UC) method (Fig. 5E). The mean size of UC-EVs was measured as 101.2 ± 7.3 nm by NTA (Fig. S10†). The concentration of particles collected after UC was measured to be 1.15 × 109 ± 4.2 × 108 particles per mL. The size of the EVs collected by EV-Lev and UC method were similar (Fig. 5D, G and Tables S5 and S6†). In addition, TEM images revealed that EV-Lev isolated EVs had a typical cup shape and did not contain any small aggregates or debris (Fig. 5C, F and S11†). Even though UC had a higher particle yield per mL, enriched EVs contained more co-precipitates compared to EV-Lev method. In conclusion, EV-Lev produced much more clean, pure, and uniform EVs isolated from human plasma, compared to ultracentrifugation.
Here, we have demonstrated the isolation of two EV subpopulations using PS and PMMA beads. However, this platform is designed with the flexibility to expand beyond two subtypes by incorporating additional bead densities and corresponding flow channels. To show that EV-Lev can be modified to accommodate simultaneous isolation of more than two beads, sorting of three beads with different densities (i.e., green: 1.02, violet: 1.06, and red: 1.09 g mL−1) in one process have been shown using a 3D-printed, three-channel magnetic levitation setup (Video S2†). This demonstration further shows the scalability and versatility of EV-Lev method as a tool for multiplexed EV isolation. Additionally, the scalability of EV-Lev approach is noteworthy; the sorting capacity could be significantly expanded by integrating parallel sorters, allowing for the processing of multiple samples concurrently in a cost-effective manner.
Experimental
Fabrication of high-throughput microfluidic magnetic levitation device
Sorting of the density beads was performed in a microfluidic magnetic levitation device, EV-Lev. EV-Lev comprises two neodymium (NdFeB) magnets, each measuring 2 mm × 5 mm × 50 mm (K&J Magnetics), and a glass capillary channel with dimensions of 1 mm × 1 mm × 50 mm and a wall thickness of 0.2 mm (VitroCom), and two aluminum side mirrors, each 1 mm × 6 mm × 50 mm (EMF Corp.). The components were assembled using PMMA pieces cut via a laser cutter. EV-Lev feature two outlet channels constructed using a 30 gauge, 0.5 inch needle (Fisnar) for the bottom outlet, and a 24 gauge, 0.5 inch needle (Fisnar) for the top outlet. The inlet and bottom outlet channels were connected using Tygon tubing, having an inner diameter (ID) of 0.010 inch and an outer diameter (OD) of 0.030 inch, respectively. The top outlet channel was connected using Tygon tubing with an ID of 0.020 inch and an OD of 0.060 inch. All tubings and microfluidic connections were securely epoxied to ensure a leak-proof sorting device. Two outlet channels were then inserted into PMMA piece with two holes, placed into the glass capillary on one end and epoxied. Lastly, the inlet tubing was epoxied directly on the other end of the glass capillary.
Preparation of filtered plasma samples from human blood
The plasma was isolated from healthy human blood following protocol by Thermo Fisher using plasma tubes treated with EDTA and filtered through a 0.22 μm filter (Millex-GV Syringe Filter Unit, PVDF). Filtered plasma was aliquoted and stored at −20 °C. ExoTIC42 device was used to obtain pre-purified extracellular vesicles using 300 μL plasma diluted in 5 mL PBS. Purified EVs were aliquoted and stored at −80 °C.
Attachment of antibodies to polymer beads
Streptavidin polymer beads were decorated with either biotin anti-CD9 antibody [MEM-61] (ab28094, Abcam), biotin anti-CD63 antibody [MEM-259] (ab134331, Abcam), or biotin anti-CD81 antibody [M38] (ab239238, Abcam) through streptavidin–biotin interaction. For 1× bead solution of PS beads, streptavidin polystyrene beads (1% in solution as packaged by company), biotinylated antibody, and 1× PBS were added to a solution at a 12
:
5
:
83 μL ratio, respectively. For 1× bead solution of PMMA beads, streptavidin-coated PMMA beads (1% in solution as packaged by company), biotinylated antibody, and 1× PBS were added to a solution at a 6
:
5
:
89 μL ratio, respectively. These solutions were incubated at 4 °C for 30 minutes on a rotating platform. After incubation, beads were washed and resuspended in 1× PBS.
Capture of extracellular vesicles from human plasma on polymer beads
The filtered plasma was added to the antibody-conjugated bead solution in PBS. This solution was incubated overnight at 4 °C on a rotating platform. After incubation, beads were spun down at 10
000×g and washed with 1× PBS.
Elution of extracellular vesicles from polymer beads
For batch characterization, beads collected in PBS were washed in 300 μL of molecular biology grade water (HyClone, HyPure Molecular Biology Grade Water). Washed beads and 100 μL of elution buffer47––20 mM Tris-HCl, 7.4 pH (Quality Biological Tris HCl, Sigma Aldrich HCl), 150 mM NaCl (Sigma Aldrich), 2 mM EDTA (Sigma Aldrich), in nuclease free water (Invitrogen)–– were mixed with a pipette and incubated at room temperature for 5 minutes. This solution was centrifuged at 10
000g for 5 minutes. The resulted supernatant (which contained the captured EVs) and pellet (which contained the beads) were used for further analysis in iSCAT, RNA measurement, TEM, SEM, and FC. For microfluidic sorting experiments, beads were eluted in 300 μL of elution buffer.
Nanoparticle tracking analysis (NTA)
For profiling the size and particle number, the sample was run through the NS300 nanoparticle tracking analyzer. The number of particles were determined by a scientific complementary metal-oxide-semiconductor (sCMOS) camera and 532 nm green laser at 25 °C. Three measurements were done per sample and the mean value was used in the graphs. NTA was performed for the measurement of plasma EVs after ultracentrifuge.
Interferometric scattering (iSCAT) microscopy
To characterize the concentration and size distribution of EVs eluted from EV-Lev, a custom interferometric scattering microscope48,49 was utilized. In iSCAT imaging, the visualization of nanosized particles was achieved through the interference between a scattered field and a background field, originating from the nanoparticles and sample substrate. This process created an interference signal on the camera plane, which was indicative of the particle's size. We used silicon (Si) substrates covered with SiO2 thin films, each having a thickness of 100 nm, as a sample substrate to immobilize EVs. The sample substrate was illuminated in a widefield configuration using an LED with a wavelength of 530 nm (Thorlabs M530L4). This was achieved by focusing the light onto the back focal plane of an objective lens (50× Nikon, LMPlanFL). The reflected signal, along with the scattered field, was then collected using the same objective lens and imaged onto a CMOS camera (Basler acA2440). For each measurement, a sample spot containing a 0.2 μL sample was created using a standard pipette and dried for 15 minutes to immobilize the particles. The sample spot containing the particles was then scanned, and z-stacks of interferometric images were acquired using a motorized sample stage (Standa). The best-focused images were determined by examining the intensity of the particle images in the acquired set. Particle detection and counting were performed using a custom MATLAB script, with particle sizes determined by correlating their sizes to the image contrast of the particles. The detected particle count in the interferometric images was converted to particles per mL to measure concentration, considering the sample volume.
Total RNA measurement
RNA was extracted from the eluted EVs which are collected from 20 μL of filtered plasma according to the MiRNeasy Kit (Qiagen) and quantified by Quantifluor RNA System Kit (Promega).
Scanning electron microscopy (SEM)
10 μL of PS bead solution was added onto the double-sided adhesive carbon tapes which were attached onto the specimen stubs and the samples were air dried. The sample was coated with gold via sputter deposition, applying a thickness of 9 nm using a sputtering system (Leica ACE600). The stubs were observed in Zeiss Sigma SEM with Schottky field emission (FE) source and GEMINI electron optical column.
Flow cytometry (FC)
Extracellular vesicles eluted from anti-CD9- or anti-CD81-coated polystyrene beads were captured by 20 μL of CD9 or CD81 magnetic beads (Invitrogen Exosome-Human CD9 or CD81 flow detection reagent; 10620D or 10616D), respectively, after a 3 hour incubation. Co-staining of eluted EVs were done by using 2 μL of fluorescent antibodies of CD9 (Miltenyi, CD9 antibody, anti-human, APC, REAfinity) and CD81 (Miltenyi, CD81 antibody, anti-human, PE, REAfinity). The mixture was incubated for an hour. The mixture was subjected to 2000×g for 5 minutes, the beads were resuspended in 150 μL of 1× PBS and transferred into a 96-well plate (Corning 96-Well Round-Bottom Microplate). FC measurement was done using a Cytek Guava (easyCyte) flow cytometry system. Red-red or yellow-blue lasers were selected for APC-CD9 or PE-CD81 antibodies, respectively. Magnetic beads of CD9 or CD81 were run as control conditions in FC.
Magnetic levitation of polymer beads
Streptavidin-coated polymer beads of two different materials and sizes were prepared: 37 μm diameter polymethyl methacrylate (PMMA) beads (PolyAn Microspheres) and 100 μm diameter polystyrene (PS) beads (Spherotech). PMMA and PS beads were suspended in a solution of 1× PBS (Gibco, pH 7.5) containing gadolinium (Gadavist, Bayer Healthcare) at increasing concentrations (50 mM, 75 mM, 100 mM, and 200 mM) and mixed until homogeneous. 30 μL of the bead solution was then injected into a capillary tube, which was sealed at both ends using Critoseal (McCormick Scientific). The capillary tube was inserted into a static magnetic levitation device. The levitation of beads within the tube was captured using an EVOS M5000 microscope (Invitrogen, Thermo Fisher). The levitation height of the beads was measured using a MATLAB code developed in-house, as previously described.38 The differences in levitation heights and the separation distances between the PS and PMMA beads were calculated and analyzed for each concentration of gadolinium.
Microfluidic sorting of polymer beads using EV-Lev
The levitation solution was prepared with 75 mM Gadavist, 0.01% pluronic in 1× PBS, and a mixture of PS and PMMA beads. Capillary sorter was attached to two 3 mL syringes (LuerLock) with a 30 gauge, 0.5 inch needle (Fisnar) for bottom outlet and a 24 gauge, 0.5 inch needle (Fisnar) for the top outlet. These syringes were then positioned in two microfluidic pumps, which were set to withdraw bead samples from the respective outlets. Prior to the introduction of the levitation solution, a solution of 0.01% pluronic and 75 mM Gadavist was flowed through the channel for one minute to prevent unspecific binding. Following this, the levitation solution was introduced into the EV-Lev at various flow rates for the top and bottom outlets, including rates such as 10–25 μL min−1, 10–50 μL min−1, 10–75 μL min−1, and 10–100 μL min−1. The sorting purity for each flow rate configuration was then assessed. Microfluidic sorting of EV-captured polymer beads using EV-Lev was performed using 450 μl filtered plasma together with a 9× of bead solution containing a mixture of PS-CD9 and PMMA-CD81. Microfluidic sorting of EV-captured beads was performed for one plasma sample with four different spot measurements on iSCAT.
Isolation of extracellular vesicles using differential ultracentrifugation (UC)
450 μL of filtered plasma was transferred into appropriate tubes (Thermo Scientific, PA Thin-Walled Tube, 33 mL) for ultracentrifugation and the volume was completed to 20 mL using sterile-filtered 1× PBS. The tube was subjected to centrifugation at 20
000×g at 4 °C for 30 minutes using a SureSpin 630 rotor, and the EV pellet was resuspended in sterile filtered 1× PBS. A subsequent wash step was applied, and the EV pellet was resuspended in sterile filtered 1× PBS. Resuspended EV pellet was filtered through a 0.22 μm syringe filter and subjected to ultracentrifugation at 100
000×g for 90 minutes, followed by a wash step of EV pellets in sterile filtered 1× PBS and ultracentrifugation at 100
000×g for 90 minutes. The final EV pellet was resuspended in 300 μL of sterile filtered 1× PBS and stored at −80 °C for further characterization by NTA and TEM. UC was performed for one plasma sample with four measurements in NTA.
Transmission electron microscopy (TEM)
300 mesh carbon coated copper grids (Formvar) were cleaned by a glow discharge system (Denton Desk III). Then, 20 μL of eluted EV sample was added onto shiny side of the grids, the sample was allowed to absorption for 5 minutes. The grids were washed by gently touching the shiny side of grid face to a droplet of double-distilled water. Later, the samples were negatively stained using 1% uranyl acetate. 3 drops of stain were flown over the shiny side of the grid and the last was allowed to remain 1 minute on the grids. The excess stain was removed by touching the grid edge with a filter paper and the grids were allowed to air dry. The grids were observed in the JEOL JEM-1400 TEM at 120 kV and photos were captured using a Gatan Orius digital camera.
Fabrication of 3D-printed microfluidic device
A Formlabs 3+ 3D resin printer, utilizing Formlabs Clear Resin V4 (RS-F2-GPCL-04), was employed to fabricate a three-channel microfluidic device. This device was designed to levitate beads of three distinct densities using 1.02, 1.06 and 1.09 g mL−1 standard density marker beads (Cospheric). Two NdFeB magnets were positioned in the same orientation as in the two-channel microfluidic device. This setup included an increased height of 3.75 mm between the magnets and three outlets, showing that EV-Lev can be modified to accommodate simultaneous isolation of multiple EV subpopulations. The beads were levitated in a medium containing 0.01% of pluronic and 150 mM of Gd in PBS. Flow rates for top, middle, and bottom channel were 500 μL min−1, 200 μL min−1, and 400 μL min−1, respectively.
Live subject statement
Anonymous, de-identified whole blood samples from healthy donors were purchased from Stanford Blood Center.
Statistical information
All experiments were repeated at least three times unless stated, and data are presented as mean ± standard deviation (SD) from the mean values of replicates. Statistical significance was determined using GraphPad Prism (Version 10) by an ordinary one-way analysis of variance (ANOVA) corrected for multiple comparisons (Tukey's) and an unpaired two-tailed t-test with Welch's correction after conducting a Kolmogorov–Smirnov test for normality.
Conclusions
Here, we developed a microfluidic platform and isolation method that leverages unique densities of beads for the separation of EVs from human plasma simultaneously. EV-Lev method, leveraging antibody–antigen interactions and microfluidics, is capable of isolating particles within the EV size range from as little as 20–50 μL of plasma, without the need for complex or expensive equipment. EV-Lev effectively collects different EV subpopulations that have overlapping sizes—a task challenging for conventional size-based isolation techniques. The current configuration of EV-Lev includes two outlet channels; however, this design can be enhanced by adding more channels to increase further functionality and enable simultaneous isolation based on multiple EV subpopulations.
Additionally, the scalability of EV-Lev approach is noteworthy; the sorting capacity could be significantly expanded by integrating parallel sorters, allowing for the processing of multiple samples concurrently in a cost-effective manner. We also note that there are some limitations of this protocol. Since it is label-dependent, it may not capture all EV sub-types especially with unknown markers. In addition, the use of antibody-conjugated beads could increase the cost per assay in clinical studies. The presented method is extremely versatile, with the potential ability to sort any biological molecules rapidly and accurately with known surface markers. In addition, our findings with healthy plasma samples suggest that EV-Lev has the potential to be a highly valuable tool for EV separation from patient samples typically available in minute quantities. In the future, we anticipate that isolation and molecular characterization of tumor derived EVs from bodily fluids could help better understand the tumor microenvironment, identify potential therapeutic targets, and therefore could be an important clinical tool for developing personalized therapies.
Data availability
The data supporting this article have been included as part of the ESI.†
Author contributions
S. Y., T. D., J. G., and N. G. D. conceptualized the study; S. Y., T. D., and N. G. D. designed the EV-Lev platform; S. Y., T. D., U. A., U. P., A. H. K., and M. R. B. performed the experiments; S. Y., T. D., U. A, U. P., and M. R. B. analyzed the data; S. Y., T. D., U. A., and N. G. D. wrote the paper. All authors read and edited the paper.
Conflicts of interest
N. G. D. is a co-founder of and has an equity interest in Levitas Bio, Inc., a company that develops new biotechnology tools for cell sorting. Her interests were viewed and managed in accordance with the conflict-of-interest policies.
Acknowledgements
We would like to thank Prima Sinawang and Dr. Jie Wang from the Bio-Acoustic MEMS in Medicine Labs (BAMM) at Stanford School of Medicine for the training on NTA and RNA extraction protocols. We would also like to thank Ruth Yamawaki and John Perrino from Stanford Cell Science Imaging Facility for the training on TEM and SEM. N. G. D. acknowledges funding support from the Career Award at the Scientific Interface (CASI) from the Burroughs Wellcome Foundation (BWF, grant number G-1018148.01), McCormick and Gabilan Faculty Award from Stanford University, and the Donald E. and Delia B. Baxter Foundation. U. A. acknowledges funding from the European Union's Horizon Europe Research and Innovation Programme under the Marie Skłodowska-Curie grant agreement No. 101066038. M. R. B. acknowledges the travel grant from OEFG for research stay and support by Eurostar grant (E115719). Research reported in this publication was also supported in part by the National Cancer Institute (NCI) of the National Institutes of Health (NIH) under award number R25CA217729 (Canary CREST Program). The project described was supported, in apart, by NIH S10 Award Number 15100D028536-01, titled “OneView 4XAK SCMOS camera for transmission electron microscopy applications” from the Office of Research Infrastructure Programs (ORIP). Its contents are solely the responsibility of the authors and do not necessarily represent the official views of the NCRR or the National Institutes of Health. We would like to thank Alfa Ozaltin, Nil Ertok, Aria Gao and Malavika Ramarao for their help in bead sorting in the 3D-printed microfluidic device.
References
- G. van Niel, G. D'Angelo and G. Raposo, Shedding light on the cell biology of extracellular vesicles, Nat. Rev. Mol. Cell Biol., 2018, 19(4), 213–228 CrossRef CAS PubMed.
- S. M. Johnson, A. Banyard, C. Smith, A. Mironov and M. G. McCabe, Large Extracellular Vesicles Can be Characterised by Multiplex Labelling Using Imaging Flow Cytometry, Int. J. Mol. Sci., 2020, 22, 8723 CrossRef PubMed.
- T. L. Whiteside, Tumor-Derived Exosomes and Their Role in Cancer Progression, Adv. Clin. Chem., 2016, 74, 103–141 CAS.
- Y. Liu, K. Shi, Y. Chen, X. Wu, Z. Chen and K. Cao,
et al. Exosomes and Their Role in Cancer Progression, Front. Oncol., 2021, 11, 639159 CrossRef CAS PubMed.
- A. Reale, T. Khong and A. Spencer, Extracellular Vesicles and Their Roles in the Tumor Immune Microenvironment, J. Clin. Med., 2022, 11(23), 6892 CrossRef CAS PubMed.
- R. Isaac, F. C. G. Reis, W. Ying and J. M. Olefsky, Exosomes as mediators of intercellular crosstalk in metabolism, Cell Metab., 2021, 33(9), 1744–1762 CrossRef CAS PubMed.
- L. M. Doyle and M. Z. Wang, Overview of Extracellular Vesicles, Their Origin, Composition, Purpose, and Methods for Exosome Isolation and Analysis, Cell, 2019, 8(7), 727 CrossRef CAS PubMed.
- I. K. Herrmann, M. J. A. Wood and G. Fuhrmann, Extracellular vesicles as a next-generation drug delivery platform, Nat. Nanotechnol., 2021, 16(7), 748–759 CrossRef CAS PubMed.
- A. Hashemi, M. Ezati, M. P. Nasr, I. Zumberg and V. Provaznik, Extracellular Vesicles and Hydrogels: An Innovative Approach to Tissue Regeneration, ACS Omega, 2024, 9(6), 6184–6218 CrossRef CAS PubMed.
- K. Warmink, J. L. Rios, S. Varderidou-Minasian, M. Torres-Torrillas, D. R. van Valkengoed and S. Versteeg,
et al. Mesenchymal stem/stromal cells-derived extracellular vesicles as a potentially more beneficial therapeutic strategy than MSC-based treatment in a mild metabolic osteoarthritis model, Stem Cell Res. Ther., 2023, 14(1), 137 CrossRef PubMed.
- B. Irmer, S. Chandrabalan, L. Maas, A. Bleckmann and K. Menck, Extracellular Vesicles in Liquid Biopsies as Biomarkers for Solid Tumors, Cancers, 2023, 15(4), 1307 CrossRef CAS PubMed.
- M. A. Di Bella, Overview and Update on Extracellular Vesicles: Considerations on Exosomes and Their Application in Modern Medicine, Biology, 2022, 11(6), 804 CrossRef CAS PubMed.
- R. E. Lane, D. Korbie, M. M. Hill and M. Trau, Extracellular vesicles as circulating cancer biomarkers: opportunities and challenges, Clin. Transl. Med., 2018, 7(1), e14 CrossRef PubMed.
- J. Hong, M. Jung, C. Kim, M. Kang, S. Go and H. Sohn,
et al. Senescent cancer cell-derived nanovesicle as a personalized therapeutic cancer vaccine, Exp. Mol. Med., 2023, 55(3), 541–554 CrossRef CAS PubMed.
- X. Zhang, H. Zhang, J. Gu, J. Zhang, H. Shi and H. Qian,
et al. Engineered Extracellular Vesicles for Cancer Therapy, Adv. Mater., 2021, 33(14), 2005709 CrossRef CAS PubMed.
- F. Shekari, M. Abyadeh, A. Meyfour, M. Mirzaei, N. Chitranshi and V. Gupta,
et al. Extracellular vesicles as reconfigurable therapeutics for eye diseases: Promises and hurdles, Prog. Neurobiol., 2023, 225, 102437 CrossRef CAS PubMed.
- R. Nieuwland, P. R. M. Siljander, J. M. Falcón-Pérez and K. W. Witwer, Reproducibility of extracellular vesicle research, Eur. J. Cell Biol., 2022, 101(3), 151226 CrossRef CAS PubMed.
- M. A. Livshits, E. Khomyakova, E. G. Evtushenko, V. N. Lazarev, N. A. Kulemin and S. E. Semina,
et al. Isolation of exosomes by differential centrifugation: Theoretical analysis of a commonly used protocol, Sci. Rep., 2015, 5(1), 17319 CrossRef PubMed.
- L. L. Yu, J. Zhu, J. X. Liu, F. Jiang, W. K. Ni and L. S. Qu,
et al. A Comparison of Traditional and Novel Methods for the Separation of Exosomes from Human Samples, BioMed Res. Int., 2018, 2018, 3634563 Search PubMed.
- Y. Weng, Z. Sui, Y. Shan, Y. Hu, Y. Chen and L. Zhang,
et al. Effective isolation of exosomes with polyethylene glycol from cell culture supernatant for in-depth proteome profiling, Analyst, 2016, 141(15), 4640–4646 RSC.
- J. Chen, P. Li, T. Zhang, Z. Xu, X. Huang and R. Wang,
et al. Review on Strategies and Technologies for Exosome Isolation and Purification, Front. Bioeng. Biotechnol., 2020,(9), 811971 Search PubMed.
- A. Gámez-Valero, M. Monguió-Tortajada, L. Carreras-Planella, M. Franquesa, K. Beyer and F. E. Borràs, Size-Exclusion Chromatography-based isolation minimally alters Extracellular Vesicles' characteristics compared to precipitating agents, Sci. Rep., 2016, 6(1), 33641 CrossRef PubMed.
- K. Sidhom, P. O. Obi and A. Saleem, A Review of Exosomal Isolation Methods: Is Size Exclusion Chromatography the Best Option?, Int. J. Mol. Sci., 2020, 21(18), 6466 CrossRef CAS PubMed.
- S. Gurunathan, M. H. Kang, M. Jeyaraj, M. Qasim and J. H. Kim, Review of the Isolation, Characterization, Biological Function, and Multifarious Therapeutic Approaches of Exosomes, Cell, 2019, 8(4), 307 CrossRef CAS PubMed.
- S. Jiawei, C. Zhi, T. Kewei and L. Xiaoping, Magnetic bead-based adsorption strategy for exosome isolation, Front. Bioeng. Biotechnol., 2022, 10, 942077 CrossRef PubMed.
- Z. Andreu and M. Yáñez-Mó, Tetraspanins in extracellular vesicle formation and function, Front. Immunol., 2014, 5, 442 Search PubMed.
-
T. F. Scientific, Exosome isolation from cell culture by ultracentrifugation: optimized protocols, 2022 Search PubMed.
- M. A. Rider, S. N. Hurwitz and D. G. Meckes, ExtraPEG: A Polyethylene Glycol-Based Method for Enrichment of Extracellular Vesicles, Sci. Rep., 2016, 6(1), 23978 CrossRef CAS PubMed.
- S. Shu, C. L. Allen, S. Benjamin-Davalos, M. Koroleva, D. MacFarland and H. Minderman,
et al. A Rapid Exosome Isolation Using Ultrafiltration and Size Exclusion Chromatography (REIUS) Method for Exosome Isolation from Melanoma Cell Lines, Methods Mol. Biol., 2021, 289–304 CrossRef CAS PubMed.
- F. Niu, X. Chen, X. Niu, Y. Cai, Q. Zhang and T. Chen,
et al. Integrated Immunomagnetic Bead-Based Microfluidic Chip for Exosomes Isolation, Micromachines, 2020, 11(5), 503 CrossRef PubMed.
- Z. Yu, S. Lin, F. Xia, Y. Liu, D. Zhang and F. Wang,
et al. ExoSD chips for high-purity immunomagnetic separation and high-sensitivity detection of gastric cancer cell-derived exosomes, Biosens. Bioelectron., 2021, 194, 113594 CrossRef CAS PubMed.
- Y. Bai, Y. Lu, K. Wang, Z. Cheng, Y. Qu and S. Qiu,
et al. Rapid Isolation and Multiplexed Detection of Exosome Tumor Markers Via Queued Beads Combined with Quantum Dots in a Microarray, Nano-Micro Lett., 2019, 11(1), 59 CrossRef CAS PubMed.
- N. He, S. Thippabhotla, C. Zhong, Z. Greenberg, L. Xu and Z. Pessetto,
et al. Nano pom-poms prepared exosomes enable highly specific cancer biomarker detection, Commun. Biol., 2022, 5(1), 660 CrossRef CAS PubMed.
- M. Tayebi, Y. Zhou, P. Tripathi, R. Chandramohanadas and Y. Ai, Exosome purification and analysis using a facile microfluidic hydrodynamic trapping device, Anal. Chem., 2020, 92(15), 10733–10742 CrossRef CAS PubMed.
- K. Delikoyun, S. Yaman, E. Yilmaz, O. Sarigil, M. Anil-Inevi and K. Telli,
et al. HologLev: A Hybrid Magnetic Levitation Platform Integrated with Lensless Holographic Microscopy
for Density-Based Cell Analysis, ACS Sens., 2021, 6(6), 2191–2201 CrossRef CAS PubMed.
- N. Puluca, N. G. Durmus, S. Lee, N. Belbachir, F. X. Galdos and M. G. Ogut,
et al. Levitating Cells to Sort the Fit and the Fat, Adv. Biosyst., 2020, 4(6), e1900300 CrossRef PubMed.
- U. Goreke, A. Bode, S. Yaman, U. A. Gurkan and N. G. Durmus, Size and density measurements of single sickle red blood cells using microfluidic magnetic levitation, Lab Chip, 2022, 22(4), 683–696 RSC.
- N. G. Durmus, H. C. Tekin, S. Guven, K. Sridhar, A. Arslan Yildiz and G. Calibasi,
et al. Magnetic levitation of single cells, Proc. Natl. Acad. Sci. U. S. A., 2015, 112(28), E3661–E3668 CrossRef CAS PubMed.
- K. Brennan, K. Martin, S. P. FitzGerald, J. O'Sullivan, Y. Wu and A. Blanco,
et al. A comparison of methods for the isolation and separation of extracellular vesicles from protein and lipid particles in human serum, Sci. Rep., 2020, 10(1), 1039 CrossRef CAS PubMed.
- A. A. Ashkarran and M. Mahmoudi, Magnetic levitation of nanoscale materials: the critical role of effective density, J. Phys. D: Appl. Phys., 2024, 57(6), 065001 CrossRef CAS.
- S. Yaman and H. C. Tekin, Magnetic Susceptibility-Based Protein Detection Using Magnetic Levitation, Anal. Chem., 2020, 92(18), 12556–12563 CrossRef CAS PubMed.
- F. Liu, O. Vermesh, V. F.-G. Mani, J. Tianjia, T. J. Ge, S. J. Madsen and A. Sabour,
et al. The Exosome Total Isolation Chip, ACS Nano, 2017, 11(11), 10712–10723 CrossRef CAS PubMed.
- M. A. Lebedeva, E. Palmieri, P. Kukura and S. P. Fletcher, Emergence and Rearrangement of Dynamic Supramolecular Aggregates Visualized by Interferometric Scattering Microscopy, ACS Nano, 2020, 14(9), 11160–11168 CrossRef CAS PubMed.
- S. Jennrich, M. Pelzer, T. Tertel, B. Koska, M. Vüllings and B. K. Thakur,
et al. CD9- and CD81-positive extracellular vesicles provide a marker to monitor glioblastoma cell response to photon-based and proton-based radiotherapy, Front. Oncol., 2022, 12, 947439 CrossRef CAS PubMed.
- K. Khanna, N. Salmond, S. Halvaei, A. Johnson and K. C. Williams, Separation and isolation of CD9-positive extracellular vesicles from plasma using flow cytometry, Nanoscale Adv., 2023, 5(17), 4435–4446 RSC.
- B. Hosseinkhani, N. M. S. van den Akker, D. G. M. Molin and L. Michiels, (Sub)populations of extracellular vesicles released by TNF-α-triggered human endothelial cells promote vascular inflammation and monocyte migration, J. Extracell. Vesicles, 2020, 9(2001–3078), 1801153 CrossRef CAS PubMed.
- W. Nakai, T. Yoshida, D. Diez, Y. Miyatake, T. Nishibu and N. Imawaka,
et al. A novel affinity-based method for the isolation of highly purified extracellular vesicles, Sci. Rep., 2016, 6(1), 33935 CrossRef CAS PubMed.
- A. D. Kashkanova, M. Blessing, A. Gemeinhardt, D. Soulat and V. Sandoghdar, Precision size and refractive index analysis of weakly scattering nanoparticles in polydispersions, Nat. Methods, 2022, 19(5), 586–593 CrossRef CAS PubMed.
- U. Aygun, H. Urey and A. Yalcin Ozkumur, Label-free detection of nanoparticles using depth scanning correlation interferometric microscopy, Sci. Rep., 2019, 9(1), 9012 CrossRef PubMed.
|
This journal is © The Royal Society of Chemistry 2025 |
Click here to see how this site uses Cookies. View our privacy policy here.