DOI:
10.1039/C0NR00145G
(Paper)
Nanoscale, 2010,
2, 1767-1772
Received
22nd February 2010
, Accepted 10th May 2010
First published on
8th July 2010
Abstract
Fundamental properties of single-walled carbon nanotubes (SWNTs) that are individually dissolved using twenty base paired-double-stranded (ds) DNA, (dA)20/(dT)20, as well as single-stranded (ss) twenty-mers of oligo DNAs, adenine (dA)20 and thymine (dT)20, for comparison are described. In this study, unbound oligo DNAs are fully removed from the hybrid aqueous solutions using size-exclusion chromatography (SEC)-HPLC. Each SEC chromatogram of the solutions shows two separated peaks; one is the free oligo DNAs and the others are the oligo DNA/SWNT hybrids. The earlier eluent fractions (the hybrids) are separated into four size-separated fractions, and then their stability is evaluated by the re-injection of the fractions. The chromatograms of the earlier eluent fractions are almost identical to those of the original ones even after storage for one month, indicating the high stability of the dsDNA/SWNTs and ssDNA/SWNTs hybrids in water. The results free us from considering the desorption of the bound-oligo dsDNA or oligo ssDNA from their nanohybrids with the SWNTs, which is of significant advantage to the utilization of oligo DNA/SWNT nanobiohybrids in wide areas of science. We also investigated the near-IR absorption and photoluminescence (PL) spectral behaviors of the fractionated oligo DNA/SWNTs hybrids not containing corresponding free oligo DNA.
Introduction
Carbon nanotubes (CNTs)1 are important nanomaterials due to their remarkable electrical, mechanical, thermal and optical properties.2 The potential applications using these materials, however, are often limited because of their insolubility in many solvents due to strong intertube van der Waals interactions. Therefore, strategic approaches for the solubilization of the CNTs are important for realizing the practical applications of the material.3 Such studies may open many doors not only in chemistry and physics, but also in biochemistry, biology, pharmaceutics, and medicine.
The combination of the CNTs and DNA (or RNA) is of fundamental and applied interest in many chemical and biochemical areas. We have already reported the finding that double-stranded DNA (dsDNA) molecules dissolve single-walled carbon nanotubes (SWNTs) in aqueous solutions.4 On the other hand, Zheng et al.5 showed that single-stranded DNA (ssDNA) solubilizes SWNTs. An interesting finding is the ability to separate metallic- and semiconducting SWNTs using ion exchange chromatography for ssDNA-dissolved SWNTs,6 as well as SWNT-size sorting using size-exclusion chromatography (SEC).7 Very recently, it has been discovered that the separation of SWNTs having a single-chirality using ion exchange chromatography for the oligo ssDNA dissolved SWNTs is possible.8
After the findings that DNA solubilizes SWNTs, many groups have endeavored to understand the fundamental properties of the SWNT/ssDNA9,10 and SWNT/dsDNA11 nanobio hybrids and their applications in nano- and nanobio-related materials science. However, one must pay attention to the fact that large amounts of unbound (free) DNA molecules exist in SWNTs/DNA aqueous solutions. It is important to understand that the hybrids containing no free DNAs in the bulk are stable enough for use in many experiments. Recently, we reported that dsDNA (∼300 base pair)/SWNT hybrids are highly stable without desorption of the bound dsDNA from the hybrids for at least one month.12 However, many researchers use oligo DNA to solubilize SWNTs, and a significant number of papers has been published describing the structure, properties and functions of their nanohybrids as already described. Richert and coauthors13 described the stability of oligo ss- and ds DNA/SWNT hybrids by measuring half life time of flocculation of the hybrids by heating to 90 °C.
However, to the best of our knowledge, no clear-cut answers have yet been published describing the stability of oligo DNA-solubilized SWNTs not containing the free unbound oligo DNA. As oligo DNAs, we did not choose sequenced-base paired oligo-DNAs but chosen homo-oligo-DNAs for simplicity. In this experiment, twenty base paired-double-stranded (ds) DNA, (dA)20/(dT)20, as well as single-stranded (ss) twenty-mers of oligo DNAs, adenine (dA)20 and thymine (dT)20, for comparison were used to prepare the size-separated oligo DNA/SWNT hybrids not containing the free oligo ds- or ssDNA, and examined their stability using SEC. We also described near-IR absorption and photoluminescence (PL) spectra of these oligo DNA/SWNT hybrid solutions. We also tested twenty-mers of guanine and cytosine; however, twenty-mer of guanine was not suitable in this study because it forms an aggregate in water.
We prepared size-separated oligo DNA/SWNT hybrids not containing the free oligo ds- or ssDNA, and examined their stability using SEC. We also described near-IR absorption and photoluminescence (PL) spectra of these oligo DNA/SWNT hybrid solutions.
Experimental section
The single stranded oligo DNAs, (dA)20 and (dT)20, were purchased from Hokkaido System Science. Their purities estimated by HPLC are greater than 99%. The as-produced SWNTs, so-called HiPco, were obtained from Carbon Nanotechnologies, Inc., and used as received. The hybridization of the (dA)20 and (dT)20 were carried out by mixing their equimolar solutions (each 3 mL, 50 μM), followed by heating to 90 °C, and then gradually allowed to cool to 5 °C. Solubilization of the SWNTs using the oligo DNAs was conducted following the procedures described in Ref. 4. Typically, ∼0.5 mg of the SWNTs was added to an aqueous solution of the oligo DNA (5 mL, 25 μM tris-EDTA buffer, pH 8.0) and sonicated using a bath-type ultrasonic cleaner (Branson 5510) at temperatures below 5 °C for 1 h, followed by centrifugation (Sigma, 3K30C) at 60000 g at temperature below 5 °C for 1 h. The top 80% of the solutions were separated and used for this study. The UV-visible-near IR spectra and PL spectra were measured at 5 °C using a spectrophotometer (JASCO, V-570) and spectrofluorometer (SPEX Fluorolog-3, Horiba-Jobin Yvon), respectively. Size-exclusion chromatography columns (CNT SEC-2000, CNT SEC-1000, and CNT SEC-300, each 10.0 (inner diameter) × 250 mm (length), Sepax Technologies, Inc.) were connected to an HPLC system (Shimadzu). Tris-HCl buffer (1 mM, pH = 8.0) containing 0.1 mM EDTA was eluted at a flow rate of 1.00 mL min−1 at 15 °C. The elution was collected at 2.00 ml/fraction. Atomic force microscopy (AFM, tapping mode) measurements were performed using an SPM-9600 (Shimadzu). The sample solution was deposited onto a freshly cleaved mica substrate, rinsed with water, and then dried before the measurement.
Results and discussion
A. Preparation of individually dissolved SWNTs
Oligo DNA hybridization of (dA)20 and (dT)20 was confirmed by measuring the temperature dependent UV-visible spectrum of the (dA)20/(dT)20; namely, the Tm of the (dA)20/(dT)20 in a tris-EDTA buffer was determined to be 27.0 °C (Fig. S1 of the ESI†). In this study, all experiments were carried out at temperatures below 15 °C that are lower than the Tm of the ds(dA)20/(dT)20. Almost no denaturation of the oligo dsDNA occurs during the sonication because the Tm values of the hybrid before and after the sonication were virtually identical (details, see Fig. S1†).
Fig. 1 shows the UV-visible-near IR spectra and near IR photoluminescence (PL) of the SWNT solutions dissolved with (dA)20/(dT)20, (dA)20 and (dT)20, in which the characteristic absorption bands in the near-IR region due to the interband transition between the mirror image spikes in the density of states of the SWNTs14 appears, and the absorption intensities of the three solutions were not much different. Weisman et al.15 reported that the raw-SWNTs dissolved in an aqueous micelle solution of sodium dodecyl sulfate individually showed the PL in the near-IR region. Since their report, considerable attention has been focused on this unique optical behavior.16 From the PL of the solutions shown in the figure, we recognized the following chiralities of the SWNTs individually dissolved in the three oligo DNA solutions: (6,5), (7,5), (8,3), (8,4), (7,6), (9,4), (10,2), (8,6), (8,7), (9,5), (10,3), (11,1), (11,3) and (10,5). Although we recognized that the (7,5), (7,6) and (9,4)SWNTs, (6,5), (7,5), (7,6) and (9,4)SWNTs, and (7,6) and (9,4)SWNTs are enriched in (dA)20/(dT)20, (dA)20, and (dT)20, respectively, the 2D-PL mapping of the three different SWNT solutions are very similar, indicating that the double-stranded oligo DNA and the single-stranded oligo DNAs have a similar performance for the chiral selectivity of the SWNTs.
B. Separation, optical properties and stability
The SEC-HPLC chromatograms were measured at 15 °C for the SWNT aqueous tris-EDTA buffer solutions dissolved with (dA)20/(dT)20, (dA)20 or (dT)20, as well as the three different oligo DNA solutions not containing the SWNT. Peaks of the chromatograms of the three different oligo-DNA solutions not containing the SWNTs appeared at around 38 min (Fig. S2 of the ESI†). As shown in Fig. 2, a (dA)20/(dT)20-solubilized SWNT solution gave two peaks in the SEC chromatograms; the first fraction can be identified as the (dA)20/(dT)20-SWNT hybrid and the second as the SWNT-unbound (free) (dA)20/(dT)20. Similar SEC chromatograms were obtained for the solutions of the (dA)20-SWNTs and (dT)20-SWNTs (Fig. S3 of the ESI†). The obtained elution profiles for the oligo DNA-solubilized SWNTs resembles the SEC chromatogram for the solutions of the ssDNA-solubilized SWNTs7 and of dsDNA (300 bp)-solubilized SWNTs.12
The first peak portion in the chromatogram of the (dA)20/(dT)20-solubilized SWNT solutions was fractionated into four samples, fr1, fr2, fr3 and fr4 (Fig. 3, left), in which we observed a transparent pale gray color (Fig. 3, right). As can be seen in Fig. 4, the UV-vis-near IR spectra of the all fractions show characteristic absorption bands assigned to the interband transition of the SWNTs, indicating that the fractions contain SWNTs. From the PL shown in Fig. 5, we see strong spots from (9,4) and (7,6), (7,5) and (7,6), (7,5) and (9,4), (7,5), (7,6), (9,4) and (10,2) in fr1, fr2 and fr3, respectively. Similar experiments were carried out for the solutions of the (dA)20-SWNTs and (dT)20-SWNTs to obtain fr1A-fr4A and fr1T-fr4T, respectively, and their chromatograms as well as the UV-vis-near IR absorption for the fractionated samples are presented in the Fig. S4 of the ESI†. We also measured the PL of the fractionated samples, and the results are shown in the Fig. S5 and Fig. S6 of the ESI†. From the 2D-PL mapping shown in Fig. 5, S5 and S6, significant difference in the chirality of the SWNTs dissolved in the fractionated samples was no observed, indicating that at the present experimental condition, the affinities of (dA)20/(dT)20, (dA)20 and (dT)20 with the SWNTs are not much different even after the size-sorting of their hybrids.
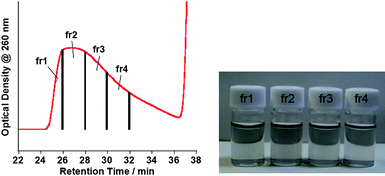 |
| Fig. 3 SEC chromatogram showing fractionation time for the (dA)20/(dT)20-solubilized SWNT solution (left) and photograph of the fractionated samples (fr1–fr4) (right). | |
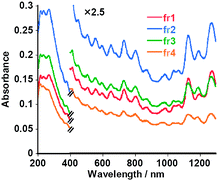 |
| Fig. 4 UV-vis-near IR absorption spectra of fractionated (dA)20/(dT)20-solubilized SWNT aqueous solutions: fr1 (red), fr2 (blue), fr3 (green) and fr4 (orange). | |
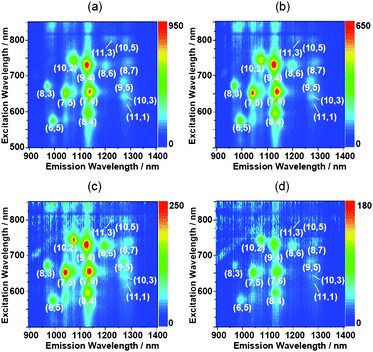 |
| Fig. 5 Near IR PL mappings of fr1 (a), fr2 (b), fr3 (c) and fr4 (d). | |
An AFM study was carried out using the obtained fractionated samples, and the results are presented in Fig. 6; from the height profiles of the AFM images, we observed the individually dissolved SWNTs. The length distributions for the randomly-selected 50 tubes in fr1, fr2, fr3 and fr4 from the (dA)20/(dT)20-SWNT hybrid solution gave average lengths of 534, 266, 156 and 105 nm, respectively (Fig. 7). It is evident that the earlier fractions contain longer nanotube hybrids than the later fractions, which is consistent with the SEC separation principle.
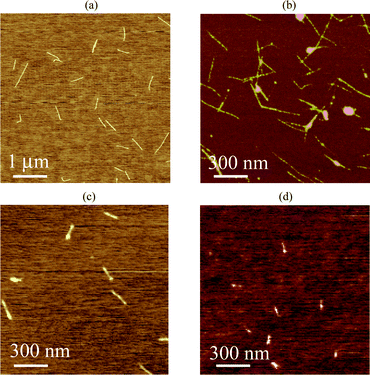 |
| Fig. 6 Typical AFM images of fractionated samples: fr1 (a), fr2 (b), fr3 (c) and fr4 (d). | |
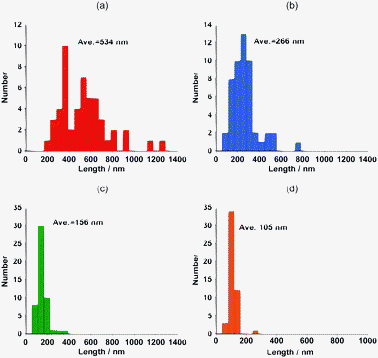 |
| Fig. 7 The length histograms obtained from the AFM images for fr1 (a), fr2 (b), fr3 (c) and fr4 (d). | |
In Fig. 8, an AFM image of the fr1 having high magnification is shown for the better understanding of the hybrids. The image suggests the formation of an oligo DNA-wrapped SWNT (details, see Fig. S7 of the ESI†, in which the height profile of the AFM image is shown together with the AFM image).
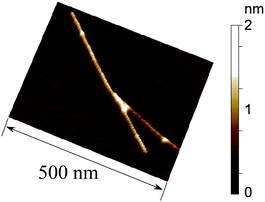 |
| Fig. 8 AFM image of (dA)20/(dT)20/SWNT hybrid (fr2). | |
The stabilities of the oligo-DNA/SWNT hybrids not containing the free oligo-DNAs were evaluated by re-injection of the SEC-fractionated solutions after storage in a refrigerator (4 °C) for 3 days, one week and one month, and the results are shown in Fig. 9. The chromatograms after 3 days and one week are virtually identical to those injected immediately after the collection of these collections. Almost no peak was observed around the retention time of 36–40 min. Even after storage for one month, the chromatograms for fr1–fr4 were virtually identical to the original, although very small peaks were observed around a retention time of 38 min, which corresponds to the retention time of the free oligo DNA. The concentration of the free oligo DNA was estimated to be ca. a few nM in the bulk solutions (details, see Table S1 of the ESI†). Fig. 10 shows the present finding schematically. As presented in Fig. 11 and Fig. 12, the SEC-fractionated hybrid solutions from the (dA)20/SWNTs ((fr1A to fr4A) and (dT)20/SWNTs (fr1T to fr4T) gave virtually the same stability as that of the (dA)20/(dT)20-SWNT hybrid, indicating that the affinities of the double-stranded oligo DNA with the SWNTs and the single-stranded DNA with the SWNTs in the buffer solutions are both very strong.
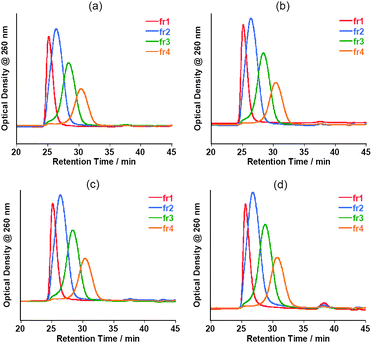 |
| Fig. 9 Chromatograms of fractionated (dA)20/(dT)20-solubilized SWNT aqueous solutions injected immediately after the separation (a) and those for the samples after storage at 4 °C for 3 days (b), 1 week (c) and 1 month (d). | |
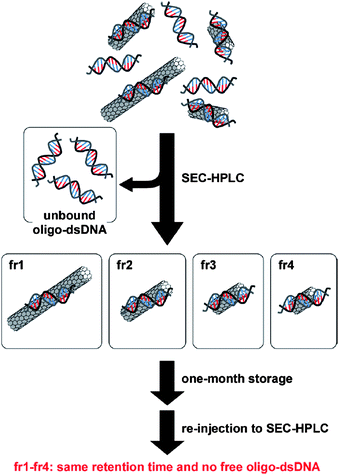 |
| Fig. 10 Schematic drawing for the sorting of oligo dsDNA/SWNTs and the stability of the sorted hybrid oligo-dsDNA/SWNTs. | |
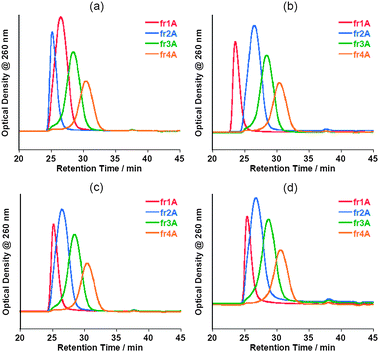 |
| Fig. 11 Chromatograms of fractionated (dA)20-solubilized SWNT aqueous solutions injected immediately after the separation (a) and those for the samples after storage at 4 °C for 3 days (b), 1 week (c) and 1 month (d). | |
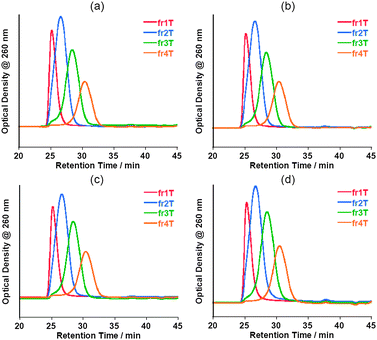 |
| Fig. 12 Chromatograms of fractionated (dT)20-solubilized SWNT aqueous solutions injected immediately after the separation (a) and those for the samples after storage at 4 °C for 3 days (b), 1 week (c) and 1 month (d). | |
Conclusions
We have removed the unwrapped oligo dsDNA and two different oligo ssDNAs from the corresponding oligo DNA-solubilized SWNT aqueous tris-EDTA buffer solutions and then obtained four fractionated size-separated oligo DNA/SWNT hybrids using SEC-HPLC treatments. Before and after the SEC-HPLC, the obtained oligo dsDNA/SWNT and oligo ssDNA/SWNT solutions gave similar visible-near IR and PL spectra. The chiral selectivity in the each four fractionated samples are somewhat different, but not significantly different. By using the re-injection procedure of the SEC-HPLC, we have discovered that, at the SEC chromatograph level, the fractionated dsDNA/SWNT and ssDNA/SWNT hybrids are highly stable without desorption from the hybrids to the unbound dsDNA (or ssDNA) for at least one month, indicating both the oligo dsDNA and ssDNA possess strong affinities with the SWNTs in the tris-EDTA buffer solutions. These results indicate that we virtually do not need to consider the desorption of the bound-oligo dsDNA or oligo ssDNA from their nanohybrids with the SWNTs in water, which is of significant advantage to the utilization of the hybrids in wide areas of science.
The present finding is important not only for the fundamental analysis of oligo dsDNA/SWNT and ssDNA/SWNT nanobio-hybrids, but also for their applications, especially in biological areas.
Acknowledgements
This work was supported by the Global COE Program “Science for Future Molecular Systems” (Kyushu University) from the Ministry of Education, Culture, Sports, Science and Technology, Japan.
Notes and references
- S. Iijima, Nature, 1991, 354, 56–58 CrossRef CAS.
-
(a)
P. J. F. Harris, Carbon nanotubes and related structures: New materials for the 21st Century, Cambridge University Press, New York, 1999 Search PubMed;
(b)
S. Reich, C. Thomsen and J. Maultzsch, Carbon Nanotubes: Basic Concepts and Physical Properties, Wiley-VCH, Weinheim, Germany, 2004 Search PubMed;
(c)
M. Meyyappan, Carbon Nanotubes: Science and Applications, CRC Press, New York, 2005 Search PubMed;
(d)
S. V. Rotkin and S. Subramoney, Applied Physics of Carbon Nanotubes: Fundamentals of Theory, Optics and Transport Devices, Springer, New York, 2005 Search PubMed;
(e)
A. Loiseau, P. Launois, P. Petit, S. Roche, J. P. Salvetat and Editors, Understanding Carbon Nanotubes. [In: Lect. Notes Phys., 2006; 677], 2006 Search PubMed;
(f)
M. J. O'Connell and Editor, Carbon Nanotubes: Properties and Applications, 2006 Search PubMed;
(g)
G. Dresselhaus, S. Dresselhaus Mildred and P. Avouris, Carbon Nanotubes: Synthesis, Structure, Properties and Applications, Springer, Berlin, 2001 Search PubMed.
-
(a) N. Nakashima, Int. J. Nanosci., 2005, 4, 119–137 CrossRef CAS;
(b) H. Murakami and N. Nakashima, J. Nanosci. Nanotechnol., 2006, 6, 16–27 CAS;
(c) D. Tasis, N. Tagmatarchis, A. Bianco and M. Prato, Chem. Rev., 2006, 106, 1105–1136 CrossRef CAS;
(d) N. Nakashima and T. Fujigaya, Chem. Lett., 2007, 36, 692–697 CrossRef CAS;
(e) A. Hirsch, Angew. Chem., Int. Ed., 2002, 41, 1853–1859 CrossRef CAS;
(f)
N. Nakashima, A. Fujigaya and H. Murakami, “Soluble Carbon Nanotubes”, in Chemistry of Carbon Nanotubes, eds, V. A. Basiuk, E. V. Basiuk, American Scientific Publisher, California, 2008, pp.113–128 (Chapter 6) Search PubMed;
(g) T. Fujigaya and N. Nakashima, Polym. J., 2008, 40, 577–589 CrossRef CAS;
(h)
N. Nakashima and T. Fujigaya, “Soluble carbon nanotubes and nanocomposite materials”, Encyclopedia of Nanoscience and Nanotechnology”, American Scientific Publishers, in press Search PubMed;
(i) T. Fujigaya, Y. Tanaka and N. Nakashima, Soluble Carbon Nanotubes and Application to Electrochemistry, Electrochemistry, 2010, 78, 2–15 CAS.
- N. Nakashima, S. Okuzono, H. Murakami, T. Nakai and K. Yoshikawa, Chem. Lett., 2003, 32, 456–457 CrossRef CAS.
- M. Zheng, A. Jagota, E. D. Semke, B. A. Diner, R. S. Mclean, S. R. Lustig, R. E. Richardson and N. G. Tassi, Nat. Mater., 2003, 2, 338–342 CrossRef CAS.
-
(a) M. Zheng, A. Jagota, M. S. Strano, A. P. Santos, P. Barone, S. G. Chou, B. A. Diner, M. S. Dresselhaus, R. S. Mclean, G. B. Onoa, G. G. Samsonidze, E. D. Semke, M. Usrey and D. J. Walls, Science, 2003, 302, 1545–1548 CrossRef CAS;
(b) M. Zheng and E. D. Semke, J. Am. Chem. Soc., 2007, 129, 6084–6085 CrossRef CAS;
(c) L. Zhang, S. Zaric, X. Tu, X. Wang, W. Zhao and H. Dai, J. Am. Chem. Soc., 2008, 130, 2686–2691 CrossRef CAS;
(d) L. Zhang, X. Tu, K. Welsher, X. Wang, M. Zheng and H. Dai, J. Am. Chem. Soc., 2009, 131, 2454–2455 CrossRef CAS.
- X. Huang, R. S. Mclean and M. Zheng, Anal. Chem., 2005, 77, 6225–6228 CrossRef CAS.
- X. Tu, S. Manohar, A. Jagota and M. Zheng, Nature, 2009, 460, 250–253 CrossRef CAS.
- Typical papers for 2004–2007 are:
(a) M. S. Strano, M. Zhen, A. Jagota, G. B. Onoa, D. A. Heller, P. W. Barone and M. L. Usrey, Nano Lett., 2004, 4, 543–550 CrossRef CAS;
(b) M. Zheng and V. V. Rostovtsev, J. Am. Chem. Soc., 2006, 128, 7702–7703 CrossRef CAS;
(c) D. A. Heller, E. S. Jeng, T.-K. Yeung, B. M. Martinez, A. E. Moll, J. B. Gastala and M. S. Strano, Science, 2006, 311, 508–511 CrossRef CAS;
(d) G. Dukovic, M. Balaz, P. Doak, N. D. Berova, M. Zheng, R. S. McLean and L. E. Brus, J. Am. Chem. Soc., 2006, 128, 9004–9005 CrossRef CAS;
(e) A. Ishibashi, Y. Yamaguchi, H. Murakami and N. Nakashima, Chem. Phys. Lett., 2006, 419, 574–577 CrossRef CAS;
(f) H. Kawamoto, T. Uchida, K. Kojima and M. Tachibana, J. Appl. Phys., 2006, 99, 094309 CrossRef;
(g) E. S. Jeng, A. E. Moll, A. C. Roy, J. B. Gastala and M. S. Strano, Nano Lett., 2006, 6, 371–375 CrossRef CAS;
(h) S. R. Vogel, M. M. Kappes, F. Hennrich and C. Richert, Chem.–Eur. J., 2007, 13, 1815–1820 CrossRef CAS;
(i) C. Lin, Y. Ke, Y. Liu, M. Mertig, J. Gu and H. Yan, Angew. Chem. Int. Ed., 2007, 46, 6089–6092 CrossRef CAS;
(j) Y. Chen, H. Liu, T. Ye, J. Kim and C. Mao, J. Am. Chem. Soc., 2007, 129, 8696–8697 CrossRef CAS;
(k) X. Zhao and J. K. Johnson, J. Am. Chem. Soc., 2007, 129, 10438–10445 CrossRef CAS;
(l) J. A. Fagan, J. R. Simpson, B. J. Bauer, S. H. Lacerda, M. L. Becker, J. Chun, K. Migler, A. R. H. Walker and E. K. Hobbie, J. Am. Chem. Soc., 2007, 129, 10607–10612 CrossRef CAS;
(m) S. R. Vogel, Katharina Müller, U. Plutowski, M. M. Kappes and C. Richert, phys. stat. sol, 2007, 244, 4026–4029 CrossRef CAS;
(n) R. A. Zangmeister, J. E. Maslar, A. Opdahl and M. J. Tarlov, Langmuir, 2007, 23, 6252–6256 CrossRef CAS;
(o) C. Fantini, A. Jorio, A. P. Santos, V. S. T. Peressinotto and M. A. Pimenta, Chem. Phys. Lett., 2007, 439, 138–142 CrossRef CAS;
(p) M. E. Hughes, E. Brandin and J. A. Golovchenko, Nano Lett., 2007, 7, 1191–1194 CrossRef CAS;
(q) S. M. Douglas, J. J. Chou and W. M. Shih, Proc. Natl. Acad. Sci. U. S. A., 2007, 104, 6644–6648 CrossRef CAS;
(r) M. L. Becker, J. A. Fagan, N. D. Gallant, B. J. Bauer, V. Bajpai, E. K. Hobbie, S. H. Lacerda, K. B. Migler and J. P. Jakupciak, Adv. Mater., 2007, 19, 939–945 CrossRef CAS;
(s) X. Han, Y. Li and Z. Deng, Adv. Mater., 2007, 19, 1518–1522 CrossRef;
(t) E. S. Jeng, P. W. Barone, J. D. Nelson and M. S. Strano, Small, 2007, 3, 1602–1609 CrossRef CAS;
(u) R. R. Lahiji, B. D. Dolash, D. E. Bergstrom and R. Reifenberger, Small, 2007, 3, 1912–1920 CrossRef CAS;
(v) X. Tu, P. E. Pehrsson and W. Zhao, J. Phys. Chem. C, 2007, 111, 17227–17231 CrossRef CAS;
(w) Q.-H. Yang, N. Gale, C. J. Oton, F. Li, A. Vaughan, R. Saito, I. S. Nandhakumar, Z.-Y. Tang, H.-M. Cheng, T. Brown and W. H. Loh, Nanotechnology, 2007, 18, 405706–405710 CrossRef;
(x) G. S. Pomales, L. S. Rodriguez, N. E. R. Velez and C. R. Cabrera, Phys. Status Solidi A, 2007, 204, 1791–1796 CrossRef;
(y) B. J. Bauer, M. L. Becker, V. Bajpai, J. A. Fagan, E. K. Hobbie, K. Migler, C. M. Guttman and W. R. Blair, J. Phys. Chem. C, 2007, 111, 17914–17918 CrossRef CAS.
- Typical papers for 2008-present are:
(a) C. Zhao, J. Ren and X. Qu, Chem.–Eur. J., 2008, 14, 5435–5439 CrossRef CAS;
(b) S. R. Shin, C. K. Lee, I. So, J.-H. Jeon, T. M. Kang, C. Kee, S. I. Kim, G. M. Spinks, G. G. Wallace and S. J. Kim, Adv. Mater., 2008, 20, 466–470 CrossRef CAS;
(c) S. E. Snyder and S. V. Rotkinp, Small, 2008, 4, 1284–1286 CrossRef CAS;
(d) H. Qian, P. T. Araujo, C. Georgi, T. Gokus, N. Hartmann, A. A. Green, A. Jorio, M. C. Hersam, L. Novotny and A. Hartschuh, Nano Lett., 2008, 8, 2706–2711 CrossRef CAS;
(e) Y. Wu, J. A. Phillips, H. Liu, R. Yang and W. Tan, ACS Nano, 2008, 2, 2023–2028 CrossRef CAS;
(f) R. R. Johnson, A. T. C. Johnson and M. L. Klein, Nano Lett., 2008, 8, 69–75 CrossRef CAS;
(g) C. Cao, J. H. Kim, D. Yoon, E.-S. Hwang, Y.-J. Kim and S. Baik, Mater. Chem. Phys., 2008, 112, 738–741 CrossRef CAS;
(h) Z. Zhou, H.-G. Kang, M. L. Clarke, S. H. D. P. Lacerda, M. Zhao, J. A. Fagan, A. Shapiro, T. Nguyen and J. Hwang, Small, 2009, 5, 2149–2155 CrossRef CAS;
(i) J. H. Kim, M. Kataoka, D. Shimamoto, H. Muramatsu, Y. C. Jung, T. Tojo, T. Hayashi, Y. A. Kim, M. Endo, M. Terrones and M. S. Dresselhaus, ChemPhysChem, 2009, 10, 2414–2417 CrossRef CAS;
(j) M. Shoda, S. Bando, Y. Maruyama and S. Iijima, J. Phys. Chem. C, 2009, 113, 6033–6036 CrossRef CAS;
(k) C. Y. Khripin, S. Manohar, M. Zheng and A. Jagota, J. Phys. Chem. C, 2009, 113, 13616–13621 CrossRef CAS;
(l) D. A. Heller, H. Jin, B. M. Martinez, D. Patel, B. M. Miller, T.-K. Yeung, P. V. Jena, C. Hobartner, T. Ha, S. K. Silverman and M. S. Strano, Nat. Nanotechnol., 2009, 4, 114–120 CrossRef CAS;
(m) R. R. Johnson, A. Kohlmeyer, A. T. C. Johnson and M. L. Klein, Nano Lett., 2009, 9, 537–541 CrossRef CAS;
(n) L. F. Pease III, D. H. Tsai, J. A. Fagan, B. J. Bauer, R. A. Zangmeister, M. J. Tarlov and M. R. Zachariah, Small, 2009, 5, 2894–2901 CrossRef.
-
(a) J. N. Barisci, M. Tahhan, G. G. Wallace, S. Badaire, T. Vaugien, M. Maugey and P. Poulin, Adv. Funct. Mater., 2004, 14, 133–138 CrossRef CAS;
(b) M. Iijima, T. Watabe, S. Ishii, A. Koshio, T. Yamaguchi, S. Bandow, S. Iijima, K. Suzuki and Y. Maruyama, Chem. Phys. Lett., 2005, 414, 520–524 CrossRef CAS;
(c) G. Lu, P. Maragakis and E. Kaxiras, Nano Lett., 2005, 5, 897–900 CrossRef CAS;
(d) G. O. Gladchenko, M. V. Karachevtsev, V. S. Leontiev, V. A. Valeev, A. Y. Glamazda, A. M. Plokhotnichenko and S. G. Stepanian, Mol. Phys., 2006, 104, 3193 CrossRef CAS;
(e) Y. Asada, T. Sugai, R. Kitaura and H. Shinohara, J. Nanomater., 2009, 2009 Search PubMed Article ID 257892.
- Y. Noguchi, T. Fujigaya, Y. Niidome and N. Nakashima, Chem. Phys. Lett., 2008, 455, 249–251 CrossRef CAS.
-
(a) S. R. Vogel, M. M. Kappes, F. Hennrich and C. Richert, Chem.–Eur. J., 2007, 13, 1815–1820 CrossRef CAS; S. R. Vogel, K. Müller, U. Plutowski, M. M. Kappes and C. Richert, Phys. Status Solidi B, 2007, 244, 4026–4029 CrossRef CAS.
- M. J. O'Connell, S. M. Bachilo, C. B. Huffman, V. C. Moore, M. S. Strano, E. H. Haroz, K. L. Rialon, P. J. Boul, W. H. Noon, C. Kittrell, J. Ma, R. H. Hauge, R. B. Weisman and R. E. Smalley, Science, 2002, 297, 593–596 CrossRef CAS.
- S. M. Bachilo, M. S. Strano, C. Kittrell, R. H. Hauge, R. E. Smalley and R. B. Weisman, Science, 2002, 298, 2361–2366 CrossRef CAS.
-
R. B. Weisman, Fluorescence spectroscopy of single-walled carbon nanotubes, Springer, New York, 2005 Search PubMed.
Footnote |
† Electronic supplementary information (ESI) available: Fig. S1–S7. See DOI: 10.1039/c0nr00145g |
|
This journal is © The Royal Society of Chemistry 2010 |
Click here to see how this site uses Cookies. View our privacy policy here.