Probing interactions of antimony species with DNA by short column capillary electrophoresis coupled with inductively coupled plasma mass spectrometry†
Received
19th July 2010
, Accepted 20th August 2010
First published on 29th September 2010
Abstract
The interaction of two inorganic antimony species (SbIII and SbV) with Herring fish DNA was studied by short column capillary electrophoresis (SC-CE) coupled with inductively coupled plasma mass spectrometry (ICP-MS). No SbV-DNA interaction was observed under simulated physiological conditions. The thermodynamic and kinetic parameters for the interaction between SbIII and DNA were determined by the SC-CE-ICP-MS assay. The stoichiometry for the interaction of SbIII with DNA was 1
:
1. The binding constant for the SbIII-DNA interaction was (1.36 ± 0.07)×106 L mol−1, showing strong affinity of SbIII to DNA under physiological condition. The interaction of SbIII with DNA was exothermic and thermodynamically favorable, both enthalpically and entropically driven. The ΔH, ΔS and ΔG values were –(8.30 ± 0.44) kJ mol−1, 90.6 ± 1.5 J mol−1 K−1, and –(28.1 ± 0.6) kJ mol−1, respectively. The binding of SbIII to DNA followed a first-order kinetics for Sb III and a zero-order kinetics for DNA with a reaction rate constant of (2.7 ± 0.1) × 10−3 h−1 at 37 °C and an apparent activation energy (Ea) of 194 ± 11 kJ mol−1.
Introduction
As evidence for antimony's toxicity continues to accumulate with similar chemical and toxicological properties to arsenic, there is increasing concern relating to the concentrations and chemical forms of this element in the environment.1 A large amount of antimony-containing compounds is released annually into the environment where a considerable interconversion of antimony compounds by chemical and biological action can occur.2 Particular concerns relate to the issue of chronic antimony exposure and the associated long-term effects that may prevail. The toxicity of antimony is largely dependent upon chemical species and the most toxic characteristics are displayed by the inorganic antimony(III) oxyanion. There is sufficient evidence for the carcinogenicity of antimony trioxide in experimental animals.3Antimony containing compounds have been used for almost a century in the clinical treatment of the parasitic disease, leishmaniasis. About 60 years ago, the trivalent antimonial drugs were substituted by less toxic pentavalent antimonials in the treatment of this disease. The pentavalent antimony compounds, ammonium-21-tungsto-9-antimoniate and NSC 13778 have been shown to exert anti-HIV activities.4–7 The mechanism of action of antimonial drugs is established that a significant fraction of SbV is reduced to SbIII following the administration of pentavalent antimonials in humans.8 Although antimony compounds have been used in medicine for decades, their molecular pharmacology and biochemical reactions are limited. So, it is imperious to secern SbV and SbIII and clarify the interaction model between two different species with ligand, in view of the dependence of the toxicity and biological behaviour on their oxidation state.2
For antimony speciation at low concentrations, capillary electrophoresis (CE) has been interfaced to inductively coupled plasma mass spectrometry (ICP-MS), one of the most efficient and robust element-specific techniques.9,10 Up to date, the CE-ICP-MS hybrid technique has been applied to study the interaction between metals or metalloids with natural ligands, such as humic substances or fulvic acids, and the interchanging with metal species in metalloproteins.11 However, there are only a few papers dealing with antimony speciation12–14 but no work relates to antimony interaction with biomolecules by CE-based hybrid techniques.
RNA and DNA are important biomolecules and some metal or metalloids interfere the DNA replication and RNA message conduction possibly.11DNA plays a key role in the synthesis of proteins (gene expression) as well as its own replication making it a potential target for drugs, especially for antiviral, antibiotic and anticancer action.15 The apparent absence of previous chemical studies of the complexation of antimony to DNA prompts us to investigate the binding of DNA to SbIII and SbV.
Here we report the application of CE-ICP-MS hybrid technique for sensitively probing the interaction between SbIII/SbV and DNA by simultaneously monitoring 121Sb for Sb species and 31P for DNA species. Considering that typical CE separation takes several minutes or even longer (ca. 30 min), short column CE (SC-CE) based on shortening of the separation pathway, are preferred to substantially shorten the separation time, as shown in our previous works for chromium within 60 s and iron within 50 s without losing the separation efficiency and selectivity, and as well to increase the efficiency of the CE-ICP-MS hyphenated technique.16,17 Here, we completed the quantitative analysis of the kinetics and thermodynamics for the interaction between SbIII and DNAin vitro, including the measurements of the equilibrium constant (K), the enthalpy change (ΔH), the entropy change (ΔS), the Gibbs free energy change (ΔG), the reaction orders (m and n) and the reaction rate constant (k). To the best of our knowledge, this is the first study regarding interaction between inorganic antimony species and DNA, and should help to understand the reactivity and chemical toxicity of SbIII towards DNA.
Experimental
Instrumentation
The separation of Sb and Sb-DNA adduct was conducted on a laboratory-built CE system, which was composed of a 0–30 kV high-voltage power supply (Model DW 300–1, Tianjin Dongwen high-voltage power supply factory, Tianjin, China), a hydrodynamic sampling unit, and a 50 μm i.d. × 375 μm o.d. fused-silica capillary with a length of 16 cm (Yongnian Optical Fiber Co., Hebei, China). The power supply was operated in voltage-controlled mode. The inlet end of the capillary was held at a positive potential while the outlet end was grounded. The sample solution was introduced into the separation capillary using a hydrodynamic method under an N2 pressure of 6.7 kPa for 6 s. A positive voltage of 15–25 kV was used for the electrophoretic separations. Prior to separation, the capillary was flushed with the running electrolyte solution for 5 min. The capillary was reconditioned daily by flushing with 0.1 mol L−1NaOH for 10 min and ultrapure water for 10 min.
An X-7 Series ICP-MS instrument with a hexapole collision/reaction cell was used for simultaneous measurement of 31P and 121Sb from the CE effluent (Thermo Elemental, Cheshire, UK). Plasmalab software was used for instrument control, data acquisition and data handling. The MicroMist nebulizer (GE, Australia) was mounted on the standard spray chamber without any modification. Prior to hyphenation with CE, the ICP-MS experimental parameters were optimized using the tuning solution containing 1.0 μg L−1 of 9Be, 59Co, 115In and 238U. Data acquisition was initiated immediately once the CE separation voltage was turned on. The ICP-MS signal was detected at a 200 ms-per-point rate. The ICP-MS settings are summarized in Table 1. All signal quantifications were done in the peak area mode by monitoring 31P for DNA and 121Sb for Sb during each CE run.
Table 1 Instrumental operation conditions for CE-ICPMS
ICP-MS
|
Thermo Elemental X-7 Series |
Plasma conditions
|
Rf power |
1280 W |
Coolant gas flow |
Ar, 14.8 L min−1 |
Auxiliary gas flow |
Ar, 1.02 L min−1 |
Nebuliser gas flow |
Ar, 0.99 L min−1 |
Spray chamber |
Twinnabar with Helix, 20 mL cyclonic, Borosilicate glass |
Nebulizer |
MicroMist |
Sampling and skimmer cones |
Nickel, 1.1 mm and 0.75 mm orifice |
Collision/reaction cell gas |
He, 4.2 mL min−1 |
Mass spectrometer
settings
|
Resolution |
Standard |
Channels monitored |
121Sb
|
31P |
Internal standard used |
115In
|
Data acquisition
|
Scanning mode |
Profile TRA |
Dwell time |
200 ms |
Integration mode |
Peak area |
CE |
Voltage applied |
16 kV |
Injection |
Hydrodynamic mode, 100 nL per injection |
Capillary |
Fused silica, 50 μm i.d. × 375 μm o.d. × 16 cm length |
Electrolyte solution |
30 mM NH4Ac + 50 mM Tris (pH 8.0) |
ICP-MS make-up buffer |
2% HNO3 added with 1 μg L−1 In |
Construction of the high efficient SC-CE-ICP-MS interface
A home-made CE-ICP-MS interface with high transmission efficiency was constructed on the basis of a cross design. The schematic diagram for the instrument set-up of the CE-ICP-MS hybrid technique is shown in Fig. 1. A 1/16-inch polyether ether ketone (PEEK) cross fitting was used to connect the make-up solution delivery PTFE tubing, the Pt electrode, the CE capillary and the micro PTFE tubing to the ICP-MS system.18 To reduce the diffusion of sample zone and to timely transport the separated Sb species in the CE effluent, the capillary was inserted into a micro-PTFE tubing (400-μm i.d. × 1 mm o.d.) through the PEEK cross and positioned directly inside the MicroMist nebulizer of the ICP-MS system by a section of thicker wall tygon tube (0.9-mm i.d. × 2-cm long) at the tail end of the micro-PTFE tubing. Dead volume was thus eliminated in the present interface by omitting an annectent part to the end of PTFE tubing to the ICP-MS system. Between the CE capillary and the inner wall of the micro-PTFE tubing, a sheath flow was introduced around the CE capillary to keep an electrical connection for stable electrophoretic separations as well as to carry the CE effluent for nebulization. The outlet of CE capillary was retracted 2–4 mm from the tip end of the micro-PTFE tubing. The CE capillary outlet was grounded through a Pt electrode and the sheath flow of 2% v/v HNO3 solution containing 1.0 μg L−1 In was produced under pressure created by an argon flow under the control of an air flow meter. The mixture of CE effluent and HNO3 solution was nebulized by the MicroMist nebulizer of the ICP-MS system, and introduced into the ICP-MS by an argon carrier. The experimental conditions of the CE-ICP-MS system are given in Table 1.
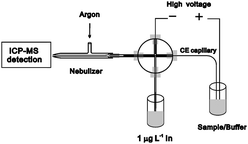 |
| Fig. 1 Schematic diagram for the interface of SC-CE hyphenated with ICP-MS. | |
Reagents
All chemicals and reagents used in this study were at least of analytical grade. Ultrapure water obtained from WaterPro water purification system (Labconco Corporation, Kansas City, MO, USA) was used throughout this work.
A 1000 mg L−1 stock standard solution for SbIII was prepared by dissolving K(SbO)C4H4O6 (Lot 042K3462, FW 324.9, Sigma-Aldrich, St. Louis. MO, USA) in ultrapure water. A 1000 mg L−1 stock standard solution for SbV was prepared by dissolving KSb(OH)6 (Lot 12K3666, FW 262.9, Sigma-Aldrich, St. Louis. MO, USA) in ultrapure water. All stock standard solutions were stored in polyethylene bottles in a refrigerator at 4 °C. Herring sperm DNA (Lot D3159, Sigma, St. Louis, MO) was used for antimony species-DNA binding study. Working standard solutions of antimony species were prepared by stepwise diluting the stock solutions immediately before analysis.
The make-up solution was prepared from electronic-pure nitric acid (Beijing Institute of Chemicals, Beijing, China) containing 1.0 μg L−1115In (100 mg L−1, National Research Center for Standard Materials, Beijing, China). The electropherotic buffer solution of 30 mmol L−1NH4Ac (Beijing Chemicals, Beijing, China) mixed with 50 mmol L−1 Tris (Beijing Chemicals, Beijing, China) was filtered through a 0.45-μm filter and degassed in an ultrasonic bath prior to use.
Stoichiometry for the binding of SbIII to DNA
The stoichiometry for the binding of SbIII to DNA can be obtained from the number of SbIII bound per DNA molecule (n), and determined from the change in the peak area according to the following equation:19 | 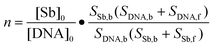 | (1) |
where [Sb]0 and [DNA]0 are the initial molar concentrations of SbIII and DNA, respectively, SSb,f and SSb,b denote the peak area for the unbound and DNA-bound SbIII, respectively, while SDNA,f and SDNA,b represent the peak area for the unbound and Sb-bound DNA, respectively.
Calculation of the thermodynamical parameters for the Sb-DNA interaction
As can be seen from Results and Discussion Section, an average stoichiometry of 1
:
1 for the binding of SbIII to DNA was obtained. Since the interaction between SbIII and DNA follows 1
:
1 stoichiometry, we obtain the following reaction:then the equilibrium constant (Kb, binding constant) can be expressed as | 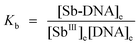 | (3) |
where [SbIII]e, [DNA]e and [Sb-DNA]e are the equilibrium concentrations of SbIII, DNA and Sb-DNA adduct, respectively.
Rearranging eqn (3) yields eqn (4):
| [Sb-DNA]e = Kb [SbIII]e[DNA]e | (4) |
Thus, a plot of [Sb-DNA]eversus[SbIII]e[DNA]e should be linear with a slope of Kb.
The values of ΔH and ΔS for the SbIII and DNA interaction can be calculated by plotting ln Kb against 1/T according to Van't Hoff equation (eqn (5)):
| 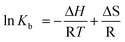 | (5) |
where R is the gas constant, and
T is absolute temperature. Once the values of Δ
H and Δ
S are known, the value of Δ
G can be calculated according to
eqn (6):
Calculation of the kinetical parameters for the Sb-DNA interaction
For the interaction between SbIII and DNA, the reaction rate can be defined as: |  | (7) |
where k is the rate constant, while n and m refer to the kinetic orders for SbIII and DNA, respectively.
When the initial concentration of DNA ([DNA]0) is excessive relative to that of SbIII, [SbIII]0, then k1 = k[DNA]m can be taken as constant, thus we can get
| 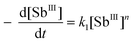 | (8) |
If t1/3 refers to the time needed for [SbIII]0 to fall to 2/3[SbIII]0, then
| t1/3 = B1([SbIII]0)1−n | (9) |
or
| lnt1/3=lnB1 + (1−n)ln[SbIII]0 | (10) |
where
B1 is a constant. Thus, the value of
n can be determined by plotting ln
t1/3 against ln [Sb
III]
0
Similarly, when the [SbIII]0 is excessive relative to [DNA]0, k2 = k[SbIII]n can be taken as constant, thus we can get
| 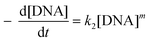 | (11) |
If t1/3 refers to the time needed for [DNA]0 to fall to 2/3[DNA]0, then
or
| lnt1/3=lnB2 + (1−m)ln[DNA]0 | (13) |
where
B2 is a constant. Thus, the value of
m can be determined by plotting ln
t1/3 against ln [DNA]
0.
For the reaction with n = 1 and m = 0, we can get
where C is a constant. Thus, the k value can be determined by plotting ln [SbIII] versust. Once the values of k at various temperatures are calculated, the apparent activation energy (Ea) can be determined according to well-known Arrhenius equation.
Procedures
A 10 mmol L−1phosphate buffer containing 100 mmol L−1NaCl at pH 7.4 was used as an incubation solution to simulate the binding experiments under physiological conditions. To determine Kb at various temperatures (293, 298, 303, 310, and 318 K) for the interaction between SbIII and DNA, an invariable concentration of DNA (2.0 μmol L−1) was incubated with various concentrations of SbIII for more than 12 h to construct the plot of [Sb-DNA]eversus[SbIII]e[DNA]e.
For the kinetic experiments, a mixture solution of fixed initial concentration of SbIII and DNA was incubated at 293, 298, 303, 310, and 318 K, respectively. Aliquots were continuously taken from the mixture at a specific temperature during the incubation course from the beginning up to 192 h for CE-ICP-MS assay to obtain a series of incubation time-dependent electrophoregrams of free SbIII and the Sb–DNA adduct.
Results and discussion
Modification of the interface setup for speciation of inorganic antimony species
Since inorganic SbIII and SbV species are both anions, species are retained owing to the electrophoretic mobility taking place towards the anode. The electroosmotic and electrophoretic movements occur in opposite directions inside the unmodified capillary. In our SC-CE-ICP-MS hybrid system, an appropriate suction force from the nebulizer was utilized to counteract with the opposite electrophoretic movement. No modification for the wall of the capillary was needed to reverse the electroosmotic flow for the Sb species with negative effective charge.12,14,20 The mobilization of SbIII and SbV species depend on the coaction of the electroosmotic movement, the suction force and electrophoretic movement. The outlet of the CE capillary was directly inserted into the MicroMist nebulizer, and the space between them was optimized by surveying the separation of SbIII and SbV. By omitting the connection tubing from the nebulizer, the interface was simplified and the transporting efficiency was improved with good stability and negligible breakage of the separation of SbIII and SbV. As shown in Fig. 2 (a), baseline separation of SbIII and SbV was obtained with a mixture of 30 mmol L−1NH4Ac and 50 mmol L−1 Tris as the running buffer. Less negatively charged SbIII migrated faster than SbV due to slower electrophoretic movement opposite to electroosmotic flow at pH 8.0. Better peak shape was obtained in more Tris contained electrolyte solution. Judging from the peak shapes and resolution, a mixture of 30 mmol L−1NH4Ac and 50 mmol L−1 Tris was optimal for further Sb speciation and interaction between Sb species and DNA. Under the optimal conditions, the SC-CE-ICP-MS hyphenated technique gave the detection limit (S/N = 3) of 2 nmol L−1 (as Sb) for free SbIII species and the DNA adduct, and 4 nmol L−1 (as DNA) for free DNA and the SbIII adduct. The low detection limits and good resolution for the separation of antimony species from the DNA adducts and from free DNA allow sensitively probing the interaction between Sb and DNA.
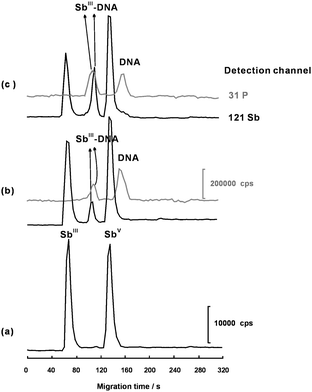 |
| Fig. 2 A series of electropherograms demonstrating the separation of SbIII and SbV and the interaction between SbIII and DNA. (a) 2.0 μmol L−1 of SbIII and SbV in the absence of DNA; (b) 2.0 μmol L−1 of SbIII and SbV incubation with 2.0 μmol L−1 of DNA for 24 h; (c) 2.0 μmol L−1 of SbIII and SbV incubation with 2.0 μmol L−1DNA for 192 h. Other conditions as shown in Table 1. | |
Evidence for the interactions of Sb species with DNA
The general potentiality of ICP-MS for DNA analyses has been reflected in several studies utilizing 31P detection for quantitative investigations of the adduct formation of calf thymus DNA and styrene oxide21,22 and melphalan.23 Pröfrock and co-workers24 used CE-ICP-MS hybrid technique with octopole reaction system in combination with less expensive quadrupole mass analysers for phosphorus determination of DNA in enzymatic digests samples. The interference of polyatomic ions like 14N16O1H+ to phosphorus was eliminated by collision cell technology (CCT) with helium as reaction gas where a collision cell is placed before the analyzer quadrupole. So, by using CCT technique, the DNA and Sb bound DNA fractions could be quantified by monitoring different phosphorus peaks (31P) of DNA with CE-ICP-MS in this work.
When DNA was incubated with SbIII and SbV solution for 12 h, a new peak appeared after SbIII in accompany with a decrease in the intensity of SbIII peak (Fig. 2(b)). As the incubation time increased, the SbIII peak gradually decreased while the fresh Sb peak increased slowly (Fig. 2(c)). However, in the case of SbV, no observable change occurred to SbV peak and no second fresh Sb peak appeared in the electropherograms. These results confirm that SbIII did bind to DNA, whereas SbV did not. Results from size-exclusion chromatography ICP-MS and direct infusion ES–MS in Pergantis's work also show that SbV could complex with an RNA oligomer, but not with a DNA oligomer because the presence of vicinal cishydroxyl groups is critical and essential for complexation of SbV with ribose to occur.25 Therefore, further studies focused on the interaction SbIII and DNA.
Thermodynamics for the interaction of SbIII and DNA
Fig. 3(a) shows a series of electropherograms for evaluating the Kb value by incubating 2.0 μmol L−1 of DNA with SbIII at different concentration levels at 310 K. From Fig. 3(a), a linear plot of [SbIII-DNA]eversus[SbIII]e[DNA]e was obtained (Fig. 3(b)). The Kb value was calculated to be (1.36 ± 0.07) × 106 L mol−1 from the slope of the fitted linear plot in Fig. 3(b). Similarly, the Kb values at other temperatures (293, 298, 303 and 318 K) were also determined for further calculation of the values of ΔH and ΔS. According to eqn (5), the values of ΔH and ΔS at 310 K and at pH 7.4 were estimated to be –(8.30 ± 0.44) kJ mol−1 and (90.6 ± 1.5) J mol−1 K−1, respectively. The ΔG value of –(28.1 ± 0.6) kJ mol−1 at 310 K was then obtained according to eqn (6).
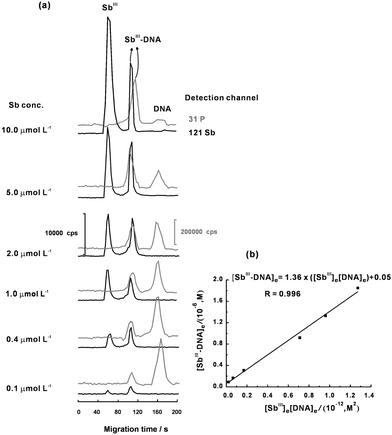 |
| Fig. 3 (a) A series of electropherograms for evaluating the Kb value by incubating 2.0 μmol L−1 of DNA with SbIII at different concentration levels. (b) Plot for calculating the Kb value. Other conditions as shown in Table 1. | |
The above results show that the interaction of SbIII with DNA are thermodynamically favorable, driven by negative enthalpy change with large positive entropy change. The negative ΔH implies that exothermically bonding reaction occurred during the covalent coordination of SbIII with the ribose moiety. As reported by Klüfers and Mayer, SbIII binds to guanosine at both the 2′ and 3′ hydroxyl positions of the ribose moiety to form a 1
:
2 Sb–guanosinecomplex after use of NMR spectroscopy.26 The negative ΔG supports a spontaneous reaction between SbIII and DNA. The observed large positive entropy changes possibly originates from the release of the water of “hydration” from DNA bases and the protonization of the trivalent antimony (SbIII) from the metallorganic compound of potassium antimony(III) tartrate.
Kinetics for the interaction between SbIII and DNA
To evaluate the kinetic order for SbIII, SbIII at various initial concentrations from 0.01 to 2.0 μmol L−1 were incubated with 200 μmol L−1DNA to obtain a series of electropherograms (Fig. 4(a)). Similarly, DNA at various initial concentration from 0.02 to 0.2 μmol L−1 were incubated with 20 μmol L−1SbIII to obtain a series of electropherograms for calculating the kinetic order for DNA (Fig. 5(a)). Plots were obtained according to eqn (10) and (13) for deducing the kinetic orders for SbIII and DNA (Fig. 4(b) and 5(b)). The results show that the binding of SbIII with DNA follows first-order kinetics for SbIII and zero-order kinetics for DNA.
![(a) A series of electropherograms obtained from the reaction mixture of SbIII (0.01–2.0 μmol L−1) and DNA (200 μmol L−1) when one third of SbIII has been reacted with DNA. (b) Plot of t1/3versusln[Sb]0 for obtaining the kinetic order on the basis of eqn (10). Other conditions as shown in Table 1.](/image/article/2011/JA/c0ja00079e/c0ja00079e-f4.gif) |
| Fig. 4 (a) A series of electropherograms obtained from the reaction mixture of SbIII (0.01–2.0 μmol L−1) and DNA (200 μmol L−1) when one third of SbIII has been reacted with DNA. (b) Plot of t1/3versusln[Sb]0 for obtaining the kinetic order on the basis of eqn (10). Other conditions as shown in Table 1. | |
![(a) A series of electropherograms obtained from the reaction mixture of DNA (0.02–0.2 μmol L−1) and SbIII (20 μmol L−1) when one third of DNA has been reacted with SbIII. (b) Plot of t1/3versusln[DNA]0 for obtaining the kinetic order for DNA on the basis of eqn (13). Other conditions as shown in Table 1.](/image/article/2011/JA/c0ja00079e/c0ja00079e-f5.gif) |
| Fig. 5 (a) A series of electropherograms obtained from the reaction mixture of DNA (0.02–0.2 μmol L−1) and SbIII (20 μmol L−1) when one third of DNA has been reacted with SbIII. (b) Plot of t1/3versusln[DNA]0 for obtaining the kinetic order for DNA on the basis of eqn (13). Other conditions as shown in Table 1. | |
A mixture solution of fixed initial concentration of SbIII and DNA was incubated to obtain a series of incubation time-dependent electrophoregrams of free SbIII and the Sb–DNA adduct during the incubation course up to 192 h (Fig. 6(a)). Then, the k value of (2.7 ± 0.1) × 10−3 h−1 at 310 K was calculated according to eqn (14). Similarly, the k values at other temperatures (293, 298, 303 and 318 K) were also determined for further calculation of the value of Ea based on well-known Arrhenius equation. The determined Ea value for the interaction between SbIII and DNA was 194 ± 11 kJ mol−1, suggesting that the interaction proceeded at a moderate rate under physiological conditions. In comparison with the previous work, the Ea value for the interaction between SbIII and DNA is much larger than those for the interactions of CdII and mercury species with DNA.27,28 Based on these kinetic results, we assume that the interaction of SbIII and DNA might proceed with slow release and protonization of the trivalent antimony (SbIII) from the metallorganic compound of potassium antimony(III) tartrate (the rate-limiting step) followed by fast coordination of the electron-deficient SbIII with the guanosine at both the 2′ and 3′ hydroxyl positions of the ribose moiety. More substantial evidences are yet required to postulate an exact kinetic mechanism for the interaction of SbIII and DNA.
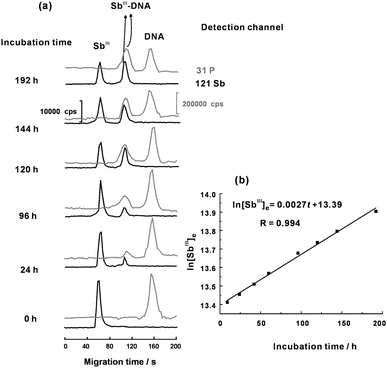 |
| Fig. 6 (a) A series of electropherograms for evaluating the k value obtained by incubating 2.0 μmol L−1 of DNA with 2.0 μmol L−1 of SbIII at different reaction time course. (b) Plot for determining k value. Other conditions as shown in Table 1. | |
Conclusions
The interaction of SbV/SbIII and DNA has been studied by SC-CE-ICP-MS assay. The obtained thermodynamical and kinetic data under the simulated conditions in this work allow an insight into the physiological process between the antimony-containing drug and DNA. The results show that SbIII has high potential affinity to DNA with favorable enthalpical change and entropical change, whereas SbV does not interact with DNA.
Acknowledgements
The authors kindly acknowledge the financial support from the National Natural Science Foundation of China (20775037, 20705014, 20935001, 20975054), the Specialized Research Fund for the Doctoral Program of Higher Education (20070055055), the Key Project of Chinese Ministry of Education (109040), the Fok Ying Tong Education Foundation (114041), the Tianjin Natural Science Foundation (08JCYBJC00600, 10JCZDJC16300), and the Program for New Century Excellent Talents (NCET-09-0482).
Notes and references
- T. Gebel, Chem.-Biol. Interact., 1997, 107, 131–144 CrossRef CAS.
- P. Smichowski, Talanta, 2008, 75, 2–14 CrossRef CAS.
- E. Rojas, L. A. Herrera, L. A. Poirier and P. Ostrosky-Wegman, Mutat. Res., 1999, 443, 157–181 CAS.
- J. Fischer, L. Ricard and R. Weiss, J. Am. Chem. Soc., 1976, 98, 3050–3052 CrossRef CAS.
- M. Souyri-Caporale, M. G. Tovey, K. Ono, C. Jasmin and J. C. Chermann, J. Gen. Virol., 1984, 65, 831–835 Search PubMed.
- R. H. Kimberlin and C. A. Walker, Arch. Virol., 1983, 78, 9–18 CrossRef CAS.
- Q. Yang, A. G. Stephen, J. W. Adelsberger, P. E. Roberts, W. Zhu, M. J. Currens, Y. Feng, B. J. Crise, R. J. Gorelick, A. R. Rein, R. J. Fisher, R. H. Shoemaker and S. Sei, J. Virol., 2005, 79, 6122–6133 CrossRef.
- N. Miekeley, S. R. Mortari and A. O. Schubach, Anal. Bioanal. Chem., 2002, 372, 495–502 CrossRef CAS.
- M. Krachler and H. Emons, TrAC, Trends Anal. Chem., 2001, 20, 79–90 CrossRef CAS.
- R. Miravet, E. Hernández-Nataren, A. Sahuquillo, R. Rubio and J. F. López-Sánchez, TrAC, Trends Anal. Chem., 2010, 29, 28–39 CrossRef CAS.
- X.-B. Yin, Y. Li and X.-P. Yan, TrAC, Trends Anal. Chem., 2008, 27, 554–565 CrossRef CAS.
- B. Michalke and P. Schramel, J. Chromatogr., A, 1999, 834, 341–348 CrossRef CAS.
- M. Krachler and H. Emons, J. Anal. At. Spectrom., 2001, 16, 20–25 RSC.
- C. Casiot, O. F. X. Donard and M. Potin-Gautier, Spectrochim. Acta, Part B, 2002, 57, 173–187 CrossRef.
- L. D. Mello, R. M. S. Pereira, A. C. H. F. Sawaya, M. N. Eberlin and L. T. Kubota, J. Pharm. Biomed. Anal., 2007, 45, 706–713 CrossRef CAS.
- B.-H. Li and X.-P. Yan, Electrophoresis, 2007, 28, 1393–1398 CrossRef CAS.
- B.-H. Li and X.-P. Yan, J. Sep. Sci., 2007, 30, 916–922 CrossRef CAS.
- Y. Li, J.-M. Liu, Y.-L. Xia, Y. Jiang and X.-P. Yan, Electrophoresis, 2008, 29, 4568–4574 CrossRef CAS.
- Y. Li, X.-P. Yan and Y. Jiang, Angew. Chem., Int. Ed., 2005, 44, 6387–6391 CrossRef CAS.
- C. Casiot, M. C. B. Alonso, J. Boisson, O. F. X. Donard and M. Potin-Gautier, Analyst, 1998, 123, 2887–2893 RSC.
- C. Siethoff, I. Feldmann, N. Jakubowski and M. Linscheid, J. Mass Spectrom., 1999, 34, 421–426 CrossRef CAS.
- M. Edler, N. Jakubowski and M. Linscheid, Anal. Bioanal. Chem., 2005, 381, 205–211 CrossRef CAS.
- M. Edler, N. Jakubowski and M. Linscheid, J. Mass Spectrom., 2006, 41, 507–516 CrossRef CAS.
- D. Pröfrock, P. Leonhard and A. Prange, J. Anal. At. Spectrom., 2003, 18, 708–713 RSC.
- H. R. Hansen and S. A. Pergantis, Anal. Bioanal. Chem., 2006, 385, 821–833 CrossRef CAS.
- P. Klüfers and P. Mayer, Z. Anorg. Allg. Chem., 1997, 623, 1496–1498 CAS.
- Y. Li, Y.-L. Xia, Y. Jiang and X.-P. Yan, Electrophoresis, 2008, 29, 1173–1179 CrossRef CAS.
- Y. Li, Y. Jiang and X.-P. Yan, Anal. Chem., 2006, 78, 6115–6120 CrossRef CAS.
Footnotes |
† This article is part of a themed issue highlighting outstanding and emerging work in the area of speciation. |
‡ Present address: College of Chemistry, Tianjin Normal University, Tianjin 300387, China. |
|
This journal is © The Royal Society of Chemistry 2011 |
Click here to see how this site uses Cookies. View our privacy policy here.