DOI:
10.1039/C1PY00259G
(Paper)
Polym. Chem., 2011,
2, 2850-2856
Influence of polymer conformations on the aggregation behaviour of alternating dialkylsilylene-[4,4′-divinyl(cyanostilbene)] copolymers†
Received
8th June 2011
, Accepted 2nd September 2011
First published on 3rd October 2011
Abstract
The influence of the substituents at silicon on the aggregation enhanced emission (AEE) of alternating dialkylsilylene-[4,4′-divinyl(cyanostilbene)] copolymers 3 and 4 is examined. The presence of the bulky isopropyl group in 3b significantly enhances the quantum yield upon aggregation. On the other hand, the emission intensity of the methyl-substituted polymer 3a is only slightly enhanced under the same conditions. The bulky isopropyl substituents on silicon may exert the Thorpe–Ingold effect on these copolymers resulting in conformation difference, which may dictate the nature of the aggregation between polymers, and hence, the photophysical behaviour of these polymers.
Introduction
The conformation of a polymer chain and the feature of its interchain interactions should be closely related, and an ensemble of these factors, inter alia, will determine the macroscopic properties of polymers and nanomaterials.1 Direct correlation of the polymer conformation with the aggregation properties, in general, is a nontrivial problem.1 Interrelationship between the conformation of poly(p-phenylene-ethynylene)s, substituted with well-designed hydrophobic and hydrophilic moieties, and interchain interactions has been explored.2 Substituents on poly(p-phenylene-vinylene)s have been shown to control the interchain interactions, hence the mode of aggregation can be adjusted.3 It is known that a change of medium,4 ionic strength,5 temperature,6 irradiation,7 and other relevant factors may affect the polymer conformation and the aggregation nature of polymers. Circular dichroic (CD) properties of certain silicon-containing polymers are enhanced upon aggregation.8,9 In this regard, the nature of the substituents on silicon may play an important role in the conformation of the polymers and hence the aggregated form.
We recently found that the photophysical properties of alternating dialkylsilylene-divinylarene copolymers 1 depend on the size of the substituent R on silicon, which may exert the Thorpe–Ingold effect on the conformation of these copolymers.9–11 The advantage of using polymer 1 is that the chromophores Ar1 and Ar2 as well as the alkyl substituent R can readily be modified. The conformational equilibrium of the distyrylsilane subunit in 1 may dictate the overall morphology of the polymer, and hence, the distance between the two adjacent chromophores in these polymers can be tuned (eqn (1)). Indeed, the STM images of 1 (Ar1 = Ar2 = 1,4-C6H4, R = Me) exhibit a fluffy morphology, whereas those of 1 with same chromophores and R = iPr show a coil-like structure.11a The emission wavelength of methyl substituted 2a appears at much shorter wavelengths than that of isopropyl-substituted 2b, presumably due to the different folding nature of these two polymers. In addition, 2a is CD inactive in dilute solution (10 mg L−1) and becomes active only in the aggregated state at a high concentration (1 g L−1), whereas 2b shows CD response at dilute conditions.
| 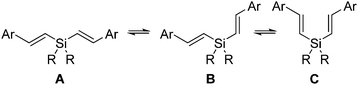 | (1) |
It is known that the emission of small molecules such as substituted cyano-substituted stilbenes can be enhanced upon aggregation.12,13 Planisation of chromophore and restriction of excimer formation in the presence of the cyano substituent may account for this unusual photophysical behaviour.12bSiloles and other related systems behave similarly. The aggregation enhanced emission (AEE) has also been found in polymers such as polysiloles,14 poly(p-phenylene-ethynylene)s,15 poly(1,4-bis(β-cyano-4′-methylstyryl)benzene)s,16 polyacetylenes,17 and poly(quinoline)s.18 It is therefore envisaged that a variation of the substituent R on silicon in 1 may offer a useful probe to elucidate the relationship between the conformation of the individual polymer and the aggregation nature of these polymers. 4,4′-Divinyl-cyanostilbene chromophore was chosen because similar monomers with related chromophores are known to exhibit aggregation induced emission (AIE).12b We now wish to report the synthesis and photophysics of alternating dialkylsilylene-[divinyl(cyanostilbene)] copolymers 3 and 4.
Results and discussion
Synthesis
The synthesis of monomer 5 and copolymers 3 and 4 are summarised in Scheme 1. The Heck reaction of aryl halides with vinylsilanes is the key protocol for the synthesis of 3–5 and the details are described in the Experimental section. It is noteworthy that the orientations of the cyano substituents in 3b are random, while those in 4 are unidirectional.
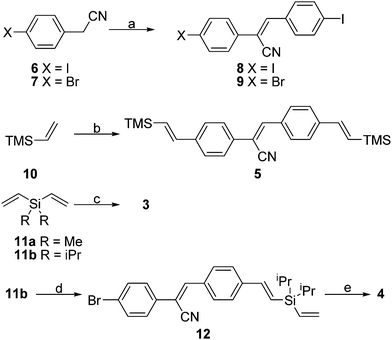 |
| Scheme 1
a: 4-Iodobenzaldehyde, NaOH, EtOH, 8: 90%, 9: 89%; b: 8 (0.5 equiv.), Pd(OAc)2. Bu4NOAc, 5: 20%; c: 8 (1 equiv.), Pd(OAc)2. Bu4NOAc, 3a: 75% (Mn = 5100, PDI = 1.85); 3b: 70% (Mn = 5600, PDI = 1.97); d: 9 (1 equiv.), Pd(OAc)2. Bu4NOAc, 12%; e: Pd(OAc)2, P(o-tol)3, 65% (Mn = 5800, PDI = 2.11). | |
Steady state photophysical properties of 3–5
The absorption spectra of monomer 5 are compared with those of polymers 3 and 4 in Fig. 1 and the relevant photophysical properties are summarised in Table 1. The absorption maximum (λmax) of 5 in THF appeared at 360 nm, whereas the absorption of the thin film of 5, prepared by thermal evaporation on a quartz substrate, exhibited broader full width at half maximum (fwhm) at the similar wavelength than that in solution. The λmax for 3a, with methyl substituents on silicon, was observed at the similar wavelength. On the other hand, the λmax for 3b and 4, having isopropyl groups on silicon, exhibited bathochromic shifts relative to those of 3a and 5. It is worth noting that the fwhm for polymers 3 and 4 was also broader than that for 5.
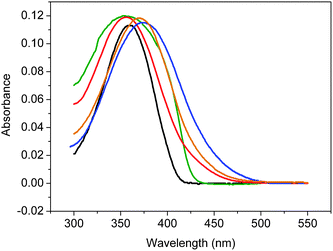 |
| Fig. 1
Absorption spectra of (a) 5 in THF (1.0 × 10−5 M, black), (b) thin film of 5 (green, arbitrary unit), (c) 3a in THF (3.2 mg L−1, red), (d) 3bTHF (3.7 mg L−1, blue), and (e) 4 in THF (3.7 mg L−1, brown). | |
Table 1 Photophysical parameters of 3a, 3b, and 4 in mixed n-hexane–THF solutions
n-Hexane/THF |
3a
|
3b
|
4
|
λ
max/nm |
λ
em/nm |
Φ
a
|
τ
b/ps |
λ
max/nm |
λ
em/nm |
Φ
a
|
τ
b/ps |
λ
max/nm |
λ
em/nm |
Φ
a
|
τ
b/ps |
Quantum yields, Φ, were estimated by using coumarin-1 (Φ = 0.99 in ethyl acetate) as the standard.
Fluorescence lifetimes, τ, were obtained by using single exponential fitting of fluorescence decay profiles.
|
0 |
358 |
489 |
0.02 |
190 |
372 |
488 |
0.05 |
180 |
370 |
493 |
0.10 |
200 |
1 |
360 |
485 |
0.05 |
190 |
370 |
478 |
0.29 |
260 |
363 |
465 |
0.32 |
290 |
489 |
2 |
360 |
472 |
0.06 |
190 |
370 |
471 |
0.45 |
310 |
363 |
464 |
0.47 |
310 |
492 |
489 |
3 |
358 |
470 |
0.06 |
200 |
370 |
466 |
0.51 |
330 |
362 |
461 |
0.50 |
330 |
496 |
485 |
4 |
359 |
467 |
0.06 |
190 |
370 |
466 |
0.52 |
350 |
360 |
460 |
0.50 |
340 |
496 |
485 |
The emission profiles of 3–5 in dilute THF solution (1 × 10−5 M) are shown in Fig. 2 and the quantum yields of these substrates are outlined in Table 1. Like the related monomer 13,12b the quantum yield of 5 was extremely low (<0.001). The emission band of 5 in solid showed a significant bathochromic shift without the vibronic fine structure (Fig. 2) and the quantum yield was 0.48. An increase of quantum yield in solid for 5 could be rationalised by the synergetic planarisation and J-type aggregation resulting in AIE.12b Indeed, the crystal structure of 5 showed that the molecule in solid was essentially planar with a dihedral angle between two benzene rings around 20°. In addition, the packing pattern also suggests that 5 might be oriented in a J-type aggregation (Fig. 3). As shown in Fig. 2, the emission maxima (λem) of 3 and 4 appeared at much longer wavelengths than that of 5 in THF but close to that of 5 in solid state. Moreover, the fluorescence intensities of 3 and 4 were much enhanced and the quantum yields for 3a, 3b and 4 in THF were 0.02, 0.05, and 0.11, respectively.
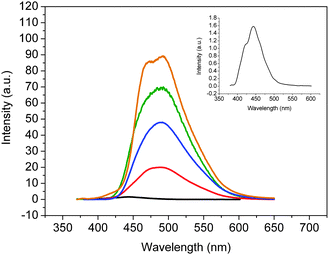 |
| Fig. 2
Emission spectra of (a) 5 in THF (1.0 × 10−5 M, black), (b) thin film of 5 (green, arbitrary unit), (c) 3a in THF (3.2 mg L−1, red), (d) 3bTHF (3.7 mg L−1, blue), and (e) 4 in THF (3.7 mg L−1, brown). Inset: expanded emission spectrum of 5 in THF. | |
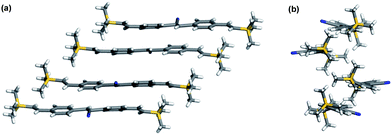 |
| Fig. 3 X-Ray packing structures of 5 (a) front view and (b) side view. | |
The monosilylene spacer in alternating dialkylsilylene-divinylarene copolymers 1 is known to be an insulator, and the possible σ–π conjugation, if any, could be neglected when other photophysical properties prevail.9–11,19–23 Accordingly, the conjugation length of the chromophore in 3 and 4 would be the same as that in 5. In general, the photophysical properties of 1 are quite similar to those of the corresponding monomers, when the conjugation length of the chromophore is long enough for the exciton delocalisation.20 On the other hand, interactions between adjacent chromophores or through space interactions in certain silicon containing copolymers 1 (e.g. Ar = Ar′ = 1,4-C6H4) are known to lead to new emission at longer wavelengths.11a,20 It is noteworthy that the fluorescence resonance energy transfer21 and photoinduced electron transfer11b,22,23 between neighbouring chromophores are common in this type of copolymers 1. Moreover, interactions between these adjacent chromophores are closely related to the distance separating these two chromophores. Indeed, the steric bulkiness of the substituents on silicon would control the relative separation between the adjacent conjugated moieties, known as the Thorpe–Ingold effect in organosilicon chemistry.10 As shown in Table 1, the quantum yields of 3b and 4 were indeed higher than that of 3a.
The aggregation behaviour of polymers was studied in a mixed solvent of n-hexane and THF. Polymers 3 and 4 are highly soluble in THF but sparingly soluble in n-hexane. It is interesting to note that these polymers gave clear solutions in 80% n-hexane and 20% THF without precipitation. Dynamic light scattering investigations revealed that the average particle sizes of 3a and 3b were 90 and 100 nm, respectively, under these conditions (Fig. 4). These results indicate that 3 may exist in an aggregated state in mixed n-hexane–THF co-solvents. The photophysical properties of 3 and 4 in these mixed solvents at different ratios are tabulated in Table 1 and the spectra are shown in Fig. 5–7. The quantum yields for 3a were only slightly increased in these mixed solvent systems. It is particularly noteworthy that the quantum yields of 3b and 4 were significantly enhanced as the ratio of n-hexane increased under the same conditions. The orientations of chromophores in 3b are random, while those in 4 are unidirectional. However, there was little discrepancy in photophysical properties between these two isomeric polymers in aggregated states.
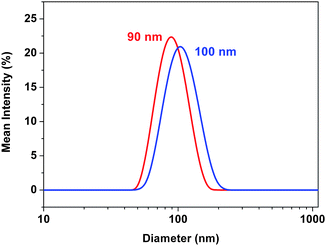 |
| Fig. 4 Particle size distribution of 3a (red) and 3b (blue) in n-hexane/THF (80/20). | |
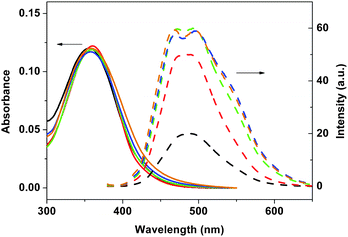 |
| Fig. 5 Absorption (solid lines) and emission spectra (dashed lines) of 3a in different ratios of n-hexane/THF: 0/100 (black), 50/50 (red), 67/33 (green), 75/25 (blue), and 80/20 (brown). | |
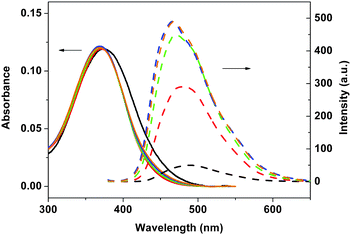 |
| Fig. 6 Absorption (solid lines) and emission spectra (dashed lines) of 3b in different ratios of n-hexane/THF: 0/100 (black), 50/50 (red), 67/33 (green), 75/25 (blue), and 80/20 (brown). | |
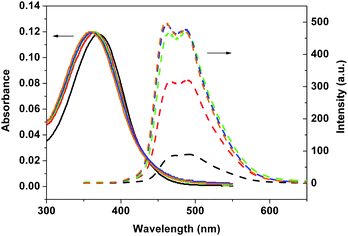 |
| Fig. 7 Absorption (solid lines) and emission spectra (dashed lines) of 4 in different ratios of n-hexane/THF: 0/100 (black), 50/50 (red), 67/33 (green), 75/25 (blue), and 80/20 (brown). | |
As shown in Fig. 5, little changes in Stokes shifts for 3a were observed for the solvent dependent photophysical behaviour. On the other hand, the Stokes shifts for 3b and 4 decreased with increasing n-hexane content in these mixed solvents. A decrease in Stokes shifts implies that the chromophore may become more rigid as the polarity of the mixed solvent decreased. As shown in Table 1, quantum yields of 3b and 4 significantly increased with the increasing n-hexane content in mixed solvents. The only structural difference between 3a and 3b is the size of the substituents on silicon (Me vs.iPr) in these polymers. It seems likely that the isopropyl substituents on silicon as in 3b and 4 would exert the Thorpe–Ingold effect on the local conformation of the divinylsilane moiety, and hence the overall conformation of the polymer.9,10 On the other hand, the methyl-substituted polymers such as in 3a would be more fluxional.9,10 Such conformational difference may affect the nature of the aggregation of these dialkylsilylene-divinylarene copolymers 3 and 4.
Solid state properties
The glass transition temperature (Tg) of 3b (150 °C) is higher than that of 3a (118 °C).†Powder X-ray diffraction (XRD) of both 3a and 3b showed three characteristic peaks at 2θ = 40, 46, and 68°.† However, the half widths of these peaks for 3a were slightly broader than those of 3b. The crystal sizes of 3a and 3b, calculated by Scherrer's formula,24 were 9.9 and 11.5 nm, respectively.† These results suggest that 3a and 3b might exhibit a similar packing structure, but the range of the ordered orientation seems to be more extended in 3b than in 3a. The scanning tunneling microscopic (STM) images of 3a and 3b are shown in Fig. 8. It is interesting to note that the morphology for each of the polymeric molecules 3a in aggregated form appears to be an elongated rod, whereas 3b shows a coil-like molecular structure. This observation was similar to those reported earlier on related polymers.11,22c The discrepancy in solid state properties between 3a and 3b might correlate to the photophysical behaviour of the aggregation properties of these polymers in solution, although the actual mode of interactions remains to be solved.
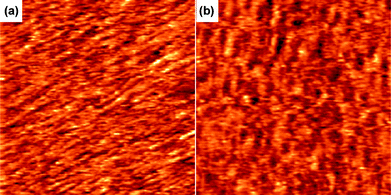 |
| Fig. 8
STM images of (a) 3a and (b) 3b on highly ordered pyrolytic graphite (HOPG). Imaging conditions: Ebias, itunneling, and image sizes were 0.95 V, 90 pA, and 50 × 50 nm. | |
Kinetic measurements
Time-resolved fluorescence spectroscopy using femto-second Ti-sapphire laser equipped with a streak camera was employed to give the fluorescence decay lifetimes by single exponential fitting. The decay profiles for 3a, 3b and 4 are shown in Fig. 9 and the results are also outlined in Table 1. The lifetimes for 3a in different mixed n-hexane–THF solvents were around 190 ps, whereas those for 3b and 4 were gradually increased from 180 to 350 ps under the same conditions. An increase of fluorescence lifetimes may be attributed to the lower contribution from other radiationless decay processes, and hence, a higher quantum yield would be expected. These kinetic results were consistent with the steady state measurement discussed above.
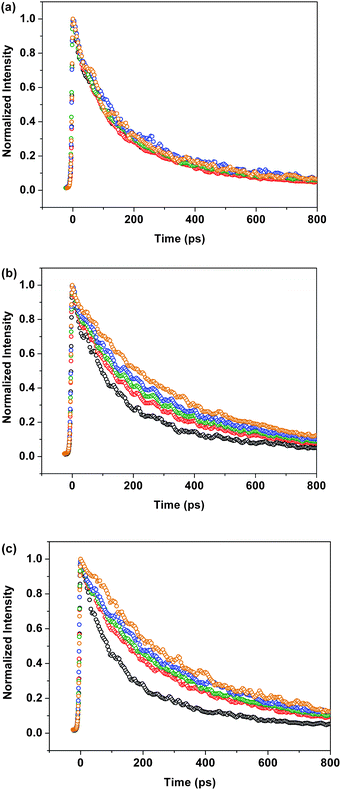 |
| Fig. 9 Fluorescence decay profiles of (a) 3a, (b) 3b, and (c) 4 in different ratios of n-hexane/THF: 0/100 (black), 50/50 (red), 67/33 (green), 75/25 (blue), and 80/20 (brown). | |
Conclusions
In summary, we have demonstrated an interesting feature on the influence of the substituents at silicon on the AEE of alternating dialkylsilylene[divinyl(cyanostilbene)] copolymers 3 and 4. The presence of the bulky isopropyl group in 3b significantly enhances the quantum yield upon aggregation. On the other hand, the emission intensity of the methyl-substituted polymer 3a is only slightly enhanced under the same conditions. It is known that the substituents on silicon may exert the Thorpe–Ingold effect on silylene-spaced divinylarene copolymers.9–11 Consequently, the conformation of 3b may be very different from that of 3a due to the steric effect. It seems likely that this conformation difference may dictate the nature of the aggregation between polymers, and hence, the photophysical behaviour of these polymers. The present study offers a preliminary platform to correlate the conformation of this silicon-containing polymer and the mode of interchain aggregation.
Experimental section
General
High-resolution mass spectrometric measurements were obtained from a Jeol-JMS-700 mass spectrometer using the FAB method in 3-nitrobenzyl alcohol matrix and the EI method was collected with a resolution of 8000(3000) (5% valley definition). Gel permeation chromatography (GPC) was performed on a Waters GPC machine using an isocratic HPLC pump (1515) and a refractive index detector (2414). THF was used as the eluent (flow rate = 1 mL min−1). Waters Styragel HR2, HR3, HR3, and HR4 (7.8 × 300 mm) columns were employed for molecular weight determination, and polystyrenes were used as the standard (Mn values ranging from 375 to 3.5 × 106). Absorption spectra were recorded on a Hitachi U-3310 spectrophotometer and emission spectra on a Hitachi F-4500 fluorescence spectrophotometer. The quantum yield was obtained using coumarin-I in EtOAc as reference (Φ = 0.99). Particle sizes of polymers in mixed co-solvent were measured on a Malvern Zetasizer Nano S90 using dynamic light scattering. X-Ray powder diffraction patterns were recorded on a PANalytical X′ Pert PRO. The glass transition temperature was measured on a LT-Modulate DSC 2920 calorimeter.
A solution of NaOH (50 mg) in ethanol (30 mL) was added dropwise to the mixture of 6 (2.43 g, 10.0 mmol) and 4-iodobenzaldehyde (2.32 g, 10.0 mmol) in ethanol (50 mL). The reaction mixture was stirred at rt for 1 h. The precipitate was collected as a white solid (4.11 g, 90%); mp 157–158 °C; 1H NMR (CDCl3, 400 MHz): δ 7.39 (d, J = 8.8 Hz, 2H), 7.44 (s, 1H), 7.59 (d, J = 8.4 Hz, 2H), 7.77 (d, J = 8.8 Hz, 2H), 7.80 (d, J = 8.4 Hz, 2H); 13C NMR (CDCl3, 100 MHz): δ 95.4, 97.3, 111.4, 117.1, 127.4, 130.5, 132.6, 133.5, 138.08, 138.09, 141.0; IR (KBr) ν 3046, 2221, 1581, 1486, 1405, 1340, 1011, 992, 926, 832, 817 cm−1; HRMS (EI+) (M+) calcd for C15H9I2N: 456.8825. Found: 456.8826.
A solution of NaOH (50.0 mg) in ethanol (30 mL) was added dropwise to the mixture of 7 (1.96 g, 10.0 mmol) and 4-iodobenzaldehyde (2.32 g, 10.0 mmol) in ethanol (50 mL). The reaction mixture was stirred at rt for 1 h. The precipitate was collected as a white solid (3.73 g, 91%); mp 135–136 °C; 1H NMR (CDCl3, 400 MHz): δ 7.42 (s, 1H), 7.52 (d, J = 8.8 Hz, 2H), 7.57 (d, J = 8.8 Hz, 2H), 7.59 (d, J = 8.4 Hz, 2H), 7.81 (d, J = 8.4 Hz, 2H); 13C NMR (CDCl3, 100 MHz): δ 97.3, 111.3, 117.2, 123.6, 127.3, 130.5, 132.1, 132.6, 132.9, 138.1, 141.0; IR (KBr) ν 3047, 2220, 1645, 1490, 1403, 1077, 1002, 919, 833, 820 cm−1; HRMS (FAB+) (M+) calcd for C15H979BrIN: 408.8963. Found: 408.8964.
A mixture of 8 (0.91 g, 2.0 mmol), 10 (0.20 g, 2.0 mmol), Pd(OAc)2 (45.0 mg, 0.2 mmol), and Bu4NOAc (1.82 g, 6.0 mmol) in dry DMF (30 mL) was stirred at 80 °C for 24 h. After filtration over celite, the crude product was chromatographed on silica gel (10% CH2Cl2/hexane) to give 8 as a yellow solid (0.16 g, 20%); mp 128–129 °C; 1H NMR (CDCl3, 400 MHz): δ 0.18 (s, 18H), 6.57 (d, J = 19.2 Hz, 1H), 6.61 (d, J = 19.2 Hz, 1H), 6.89 (d, J = 19.2 Hz, 1H), 6.90 (d, J = 19.2 Hz, 1H), 7.45–7.55 (m, 5H), 7.64 (d, J = 8.4 Hz, 2H), 7.88 (d, J = 8.4 Hz, 2H); 13C NMR (CDCl3, 100 MHz): δ −1.12, −1.11, 110.5, 118.0, 125.9, 126.7, 126.8, 129.5, 131.3, 132.30, 133.0, 133.7, 139.0, 140.2, 140.9, 142.2, 142.3; IR (KBr) ν 3056, 2993, 2955, 2894, 2217, 1600, 1511, 1416, 1251, 982, 868, 836 cm−1; HRMS (FAB+) (M+) calcd for C25H31NSi2: 401.1995. Found: 401.1989.
A mixture of 9 (0.82 g, 2.0 mmol), 11b (0.34, 2.0 mmol), Pd(OAc)2 (22.0 mg, 0.1 mmol), and Bu4NOAc (1.21g, 4.0 mmol) in dry DMF (30 mL) was stirred at 80 °C for 24 h. After filtration over celite, the crude product was chromatographed on silica gel (10% CH2Cl2/hexane) to give 12 as a pale yellow solid (90.0 mg, 12%); mp 88–89 °C; 1H NMR (CDCl3, 400 MHz): δ 1.00–1.20 (m, 14H), 5.85 (dd, J = 7.8, 17.0 Hz, 1H), 6.15 (d, J = 17.0 Hz, 1H), 6.18 (d, J = 7.8 Hz, 2H), 6.56 (d, J = 19.2 Hz, 1H), 7.00 (d, J = 19.2 Hz, 1H), 7.50 (s, 1H), 7.53–7.59 (m, 6H), 7.88 (d, J = 8.4 Hz, 2H); 13C NMR (CDCl3, 100 MHz): δ 11.5, 18.3, 18.4, 109.7, 117.4, 123.0, 125.6, 126.5, 127.0, 129.3, 131.8, 132.48, 132.50, 133.1, 134.5, 140.1, 141.5, 145.0; IR (KBr) ν 3120, 3047, 2983, 2222, 1640, 1491, 1403, 1390, 1070, 998, 910, 818 cm−1; HRMS (FAB+) (M+) calcd for C25H28BrNSi: 449.1174. Found: 449.1176.
General procedure for the preparation of 3
A mixture of dialkyldivinylsilane (0.2 mmol), 8 (82.0 mg, 0.2 mmol), Pd(OAc)2 (3.0 mg), and Bu4NOAc (60.0 mg, 0.2 mmol) in dry DMF (3.0 mL) was refluxed under N2 for 18 h. After cooling to rt, the mixture was poured into EtOAc. The precipitate was collected and dissolved in THF, and then reprecipitated with MeOH. The product was collected by filtration and washed with MeOH.
Yield: 75%; 1H NMR (CDCl3, 400 MHz): δ 0.20–0.40 (br, 6H), 6.55–6.75 (br, 2H), 6.90–7.05 (br, 2H), 7.40–8.00 (m, 9H); IR (KBr) ν 3027, 2983, 2957, 2213, 1603, 1509, 1415, 1251, 1041, 986, 840, 804 cm−1; Mn = 5100, PDI = 1.85.
Yield: 70%; 1H NMR (CDCl3, 400 MHz): δ 1.00–1.40 (m, 14H), 6.50–6.70 (br, 2H), 7.00–7.15 (br, 2H), 7.50–8.00 (m, 9H); IR (KBr) ν 3027, 2939, 2806, 2214, 1603, 1508, 1460, 1416, 1024, 989, 794 cm−1; Mn = 5600, PDI = 1.97.
A mixture of 12 (90.0 mg, 0.2 mmol), Pd(OAc)2 (3.0 mg), and P(o-tol)3, (5.0 mg) in dry DMF (4.0 mL) was refluxed under N2 for 24 h. After cooling to rt, the mixture was poured into EtOAc. The precipitate was collected and dissolved in THF, and then reprecipitated with MeOH. The product was collected by filtration and washed with MeOH to give product (49.0 mg, 65%); 1H NMR (CDCl3, 400 MHz): δ 1.00–1.35 (m, 14H), 6.50–6.65 (br, 2H), 7.00–7.15 (br, 2H), 7.50–7.80 (m, 7H), 7.85–8.00 (m, 2H); IR (KBr) ν 3027, 2940, 2862, 2211, 1601, 1510, 1461, 1260, 1094, 1014, 989, 880, 759 cm−1; Mn = 5800, PDI = 2.11.
Time-resolved fluorescence experiments
A mode-locked Ti:sapphire laser (wavelength: 740 nm; repetition rate: 76 MHz; pulse width: <200 fs) passed through an optical parametric amplifier to produce 370 nm pulse laser. The fluorescence of the sample was reflected by a grating (150 g mm−1; BLZ: 500 nm) and detected by an optically triggered streak camera (Hamamatsu C5680) with a time resolution of about 0.3 ps. The sample was prepared with 1.0 × 10−5 M concentration, and using ultra-micro cuvette with 1 mm pathlength to maintain the excitation at the same time. The signal was collected for ten times to decrease the signal to noise ratio.
STM imaging
An aliquot of polymer in solvent (n-hexane
:
THF = 1
:
1) was placed on HOPG (Advanced Ceramics, ZYH grade) followed by a treatment of shear flow.25STM imaging was carried out with a NanoScope IIIa controller (Veeco Metrology Group/Digital Instruments) at room temperature. The STM probes were commercially available Pt/Ir tips (PT, Nanotips, Veeco Metrology Group/Digital Instruments). Typical imaging conditions of bias voltage and tunneling current were about 0.95 V and 90 pA, respectively. The images were filtered with a first-order flattening to minimise noise and without further processing.
Preparation of aggregated polymer
THF solutions of polymer 3 or 4 were prepared and the concentrations were adjusted to be 2.0 × 10−5, 3.0 × 10−5, 4.0 × 10−5, and 5.0 × 10−5 M. These aliquots (1.0 mL) were slowly added (1.0 mL min−1), respectively, into different volumes of n-hexane (1.0 mL, 2.0 mL, 3.0 mL, and 4.0 mL) under ultrasound irradiation to form the aggregates of polymers for photophysical measurements.
Acknowledgements
We thank the National Science Council and the National Taiwan University for support. We thank Professor Huan-Cheng Chang for helpful discussion.
Notes and references
- For reviews, see:
(a) Ph. Leclère, E. Hennebicq, A. Calderone, P. Brocorens, A. C. Grimsdale, K. Müllen, J. L. Brédas and R. Lazzaroni, Prog. Polym. Sci., 2003, 28, 55–81 CrossRef;
(b) C. R. Ray and J. S. Moore, Adv. Polym. Sci., 2005, 177, 91–150 CAS;
(c) J. Zheng and T. M. Swager, Adv. Polym. Sci., 2005, 177, 151–179 CAS.
-
(a) J. Kim and T. M. Swager, Nature, 2001, 41, 1030–1034 CrossRef;
(b) J. Kim, I. A. Levitsky, D. T. McQuade and T. M. Swager, J. Am. Chem. Soc., 2002, 124, 7710–7718 CrossRef CAS;
(c) Z. Zhu and T. M. Swager, J. Am. Chem. Soc., 2001, 124, 9670–9671 CrossRef.
- T.-Q. Nguyen, V. Doan and B. J. Schwartz, J. Chem. Phys., 1999, 110, 4068–4078 CrossRef CAS.
-
(a) Z. G. Zhang, J. P. Deng, J. W. Li and W. T. Yang, Polym. J., 2008, 40, 436–441 CrossRef CAS;
(b) S. Ghosh, A. Sannigrahi, S. Maity and T. Jana, J. Phys. Chem. B, 2010, 114, 3122–3132 CrossRef CAS.
-
(a) R. Traiphol, N. Charoenthai, T. Srikhirin, T. Kerdcharoen, T. Osotchan and T. Maturos, Polymer, 2007, 48, 813–826 CrossRef CAS;
(b) Q.-L. Fan, Y. Zhou, X.-M. Lu, X.-Y. Hou and W. Huang, Macromolecules, 2005, 38, 2927–2936 CrossRef CAS;
(c) A. P.-Z. Clark, A. J. Cadby, C. K.-F. Shen, Y. Rubin and S. H. Tolbert, J. Phys. Chem. B, 2006, 110, 22088–22096 CrossRef CAS.
-
(a) F. B. Dias, J. Morgado, A. L. Macanita, F. P. da Costa, H. D. Burrows and A. P. Monkman, Macromolecules, 2006, 39, 5854–5864 CrossRef CAS;
(b) R. Traiphol, P. Sanguansat, P. Srikhirin, T. Kerdcharoen and T. Osotchan, Macromolecules, 2006, 39, 1165–1172 CrossRef CAS.
- E. J. Harbron, D. A. Vicente, D. H. Hadley and M. R. Imm, J. Phys. Chem. A, 2005, 109, 10846–10853 CrossRef CAS.
-
(a) H. Nakashima, M. Fujiki, J. R. Koe and M. Motonaga, J. Am. Chem. Soc., 2001, 123, 1963–1969 CrossRef CAS;
(b) W. Peng, M. Motonaga and J. R. Koe, J. Am. Chem. Soc., 2004, 126, 13822–13826 CrossRef CAS.
-
(a) Y.-J. Cheng, H. Liang and T.-Y. Luh, Macromolecules, 2003, 36, 5912–5914 CrossRef CAS;
(b) M.-Y. Yeh and T.-Y. Luh, Chem.–Asian J., 2008, 3, 1620–1624 CrossRef CAS.
- T.-Y. Luh and Z. Hu, Dalton Trans., 2010, 39, 9185–9192 RSC.
-
(a) M.-Y. Yeh, H.-C. Lin, S.-L. Lee, C.-h. Chen, T.-S. Lim, W. Fann and T.-Y. Luh, Chem. Commun., 2007, 3459–3461 RSC;
(b) M.-Y. Yeh, H.-C. Lin, S.-L. Lee, C.-h. Chen, T.-S. Lim, W. Fann and T.-Y. Luh, Macromolecules, 2007, 40, 9238–9243 CrossRef CAS.
-
(a) J. Luo, Z. Xie, J. W. Y. Lam, L. Cheng, H. Chen, C. Qiu, H. S. Kowk, X. Zhan, Y. Liu, D. Zhu and B. Z. Tang, Chem. Commun., 2001, 1740–1741 RSC;
(b) B.-K. An, S.-K. Kwon, S. D. Jung and S. Y. Park, J. Am. Chem. Soc., 2002, 124, 14410–14415 CrossRef CAS.
- For a review, see: Y. Hong, J. W. Y. Lam and B. Z. Tang, Chem. Commun., 2009, 4332–4353 RSC.
-
(a) A. Qin, C. K. W. Jim, Y. Tang, J. W. Y. Lam, J. Liu, F. Mahtab, P. Gao and B. Z. Tang, J. Phys. Chem. B, 2008, 112, 9281–9288 CrossRef CAS;
(b) W. Z. Yuan, H. Zhao, X. Y. Shen, F. Mahtab, J. W. Y. Lam, J. Z. Sun and B. Z. Tang, Macromolecules, 2009, 42, 9400–9411 CrossRef CAS;
(c) Z. Zhao, D. Liu, F. Mahtab, L. Xin, Z. Shen, Y. Yu, C. Y. K. Chan, P. Lu, J. W. Y. Lam, H. H. Y. Sung, I. D. Williams, B. Yang, Y. Ma and B. Z. Tang, J. Chem. Educ., 2011, 17, 5998–6008 CAS.
-
(a) A. Satrijo and T. M. Swager, J. Am. Chem. Soc., 2007, 129, 16020–16028 CrossRef CAS;
(b) J. H. Wosnick, C. M. Mello and T. M. Swager, J. Am. Chem. Soc., 2005, 127, 3400–3405 CrossRef CAS;
(c) A. Satrijo, S. E. Kooi and T. M. Swager, Macromolecules, 2007, 40, 8833–8841 CrossRef CAS.
- S.-J. Lim, B.-K. An and S.-Y. Park, Macromolecules, 2005, 38, 6236–6239 CrossRef CAS.
- W. Z. Yuan, A. Qin, J. W. Y. Lam, J. Z. Sun, Y. Dong, M. Häussler, J. Liu, H. P. Xu, Q. Zheng and B. Z. Tang, Macromolecules, 2007, 40, 3159–3166 CrossRef CAS.
- W. Y. Huang, H. Yun, H. S. Lin, T. K. Kwei and Y. Okamoto, Macromolecules, 1999, 32, 8089–8093 CrossRef CAS.
- For a review, see: T.-Y. Luh and Y.-J. Cheng, Chem. Commun., 2006, 4669–4678 RSC.
-
(a) Y.-J. Cheng, S. Basu, S.-j. Luo and T.-Y. Luh, Macromolecules, 2005, 38, 1442–1446 CrossRef CAS;
(b) R.-M. Chen, K.-M. Chien, K.-T. Wong, B.-Y. Jin, T.-Y. Luh, J.-H. Hsu, W. Fann and T.-Y. Luh, J. Am. Chem. Soc., 1997, 119, 11321–11322 CrossRef CAS.
-
(a) Y.-J. Cheng, T.-Y. Hwu, J.-H. Hsu and T.-Y. Luh, Chem. Commun., 2002, 1978–1979 RSC;
(b) Y.-J. Cheng and T.-Y. Luh, Chem.–Eur. J., 2004, 10, 5361–5368 CrossRef CAS;
(c) Y.-J. Cheng and T.-Y. Luh, Macromolecules, 2005, 38, 4563–4568 CrossRef CAS.
-
(a) H.-W. Wang, Y.-J. Cheng, C.-H. Chen, T.-S. Lim, W. Fann, W. C.-L. Lin, Y.-P. Chang, K.-C. Lin and T.-Y. Luh, Macromolecules, 2007, 40, 2666–2671 CrossRef CAS;
(b) M.-Y. Yeh, H.-C. Lin, S. L. Lee, C.-H. Chen, T.-S. Lim, W. Fann and T.-Y. Luh, Macromolecules, 2007, 40, 9238–9243 CrossRef CAS;
(c) H.-W. Wang, M.-Y. Yeh, C.-H. Chen, T.-S. Lim, W. Fann and T.-Y. Luh, Macromolecules, 2008, 41, 2762–2770 CrossRef CAS.
-
(a) C. A. van Walree, M. R. Roest, W. Schuddeboom, L. W. Jenneskens, J. W. Verhoeven, J. M. Warman, H. Kooijman and A. L. Spek, J. Am. Chem. Soc., 1996, 118, 8395–8407 CrossRef CAS;
(b) A. Zehnacker, F. Lahmani, C. A. van Walree and L. W. Jenneskens, J. Phys. Chem. A, 2000, 104, 1377–1387 CrossRef CAS.
-
Elements of X-Ray Diffraction, ed. B. D. Cullity and S. R. Stock, Prentice Hall, 3rd edn, 2001 Search PubMed.
-
(a) S.-L. Lee, N.-T. Lin, C.-h. Chen, H.-C. Yang and T.-Y. Luh, Chem.–Eur. J., 2009, 15, 11594–11600 CrossRef CAS;
(b) S.-L. Lee, C.-Y. J. Chi, M.-J. Huang, C.-h. Chen, C.-W. Li, K. Pati and R.-S. Liu, J. Am. Chem. Soc., 2008, 130, 10454–10455 CrossRef CAS.
Footnote |
† Electronic supplementary information (ESI) available: XRD patterns of 3a and 3b, differential scanning calorimetry curves of 3a and 3b, 1H and 13C NMR spectra of 5, 8, 9, and 12, 1H NMR spectra of polymers 3a, 3b, and 4, the crystallographic data for 5, and crystal size estimation. CCDC reference numbers 842709. For ESI and crystallographic data in CIF or other electronic format see DOI: 10.1039/c1py00259g |
|
This journal is © The Royal Society of Chemistry 2011 |
Click here to see how this site uses Cookies. View our privacy policy here.