DOI:
10.1039/C2AN16141A
(Paper)
Analyst, 2012,
137, 1343-1350
Use of cellular electrical impedance sensing to assess in vitro cytotoxicity of anticancer drugs in a human kidney cell nephrotoxicity model†
Received
22nd November 2011
, Accepted 9th December 2011
First published on 12th January 2012
Abstract
Nephrotoxicity is one of the major concerns for anticancer drug safety because most drugs are metabolized and excreted by the kidneys. Convenient tools able to perform rapid in vitro cytotoxicity analysis and identify drug side effects in kidney cells during early phases of drug discovery could be beneficial to drug development programs. Here we developed an electrical cell-substrate impedance sensing system (ECIS) capable of continuously measuring the dosage and time response of human proximal tubular epithelial (HK2) cells exposed to four drugs throughout the experimental period. These drugs induced HK2 cell apoptosis/death in a dose-dependent manner, although with very different dose-response effects. DDP (50 μM) was the most cytotoxic and induced obvious HK2 cell apoptosis rapidly after exposure. The other three drugs had much lower cytotoxicity, even at concentrations approaching 1 mM. The results obtained from our ECIS system correlated well with conventional in vitro assays such as flow cytometry and cell viability assays. Notably, the continuous and automatic measurements provided by ECIS system allow for better resolution for drugs with different temporal toxicity profiles. Furthermore, we investigated the effect of DDP's antidotes, glutathione and sodium subsulfite, on DDP-induced cytotoxicity, both of which decreased nephrotoxicity of DDP in a dose-dependent manner. Overall this study illustrates the convenience of ECIS for direct, continuous assessment of the cytotoxicity of anticancer drugsin vitro. ECIS has the potential to become a useful, non-invasive analytical method for early evaluation of drugs and antidotes of toxins.
1. Introduction
Although a wide spectrum of potent anticancer drugs are used for cancer chemotherapy, new highly active drugs with better human tolerance and safety are continuously sought. The determination of potential cytotoxic side effects on human organs is one of the important issues in the determination of the safety of chemicals. Most of these drugs are metabolized by the kidneys and the nephrotoxicity of drugs is a major concern, particularly during anticancer treatment.1–3 The mechanisms of drug-induced nephrotoxicity are complex, however direct toxicity of drugs to renal tubular cells4 is considered paramount as these cells are exposed to far higher drug levels than that in the blood due to their role in concentrating and reabsorbing the glomerular filtrate.5 Although animal models are typical and necessary methods to assess nephrotoxicity,6 the experiments are expensive and labor-intensive. Therefore, in vivo animal experiments are not suitable for high throughput drug screening. In vitro model cell cytotoxicity assays are believed to represent a convenient alternative to some aspects of animal testing of the nephrotoxicity of candidate drugs particularly for high throughput early assessment of the major cell status effects of drugs, and for high throughput molecular assays of pathways and mechanisms activated or inhibited by the test compounds.7In vitrocell assays can readily provide valuable preliminary drug toxicity information at a much higher throughput than animal tests or molecular-level tests.8,9 Many of these in vitrocell-based assays involve markers or enzymes, such as neutral red uptake, lactate dehydrogenase activity or the 3-(4,5-dimethylthiazol-2-yl)-2,5-diphenyltetrazolium bromide (MTT) assay7–9 to assess the quantitative cytotoxicity of drugs by evaluating cell viability under stimulation. However one major common disadvantage of these indicator methods is that they are typically undertaken at a limited number of defined time points, and thus do not provide insight of the cellular dynamics between the cells and the drug under test.
In contrast, electrical cell-substrate impedance sensing (ECIS) is a label-free and non-invasive/non-damaging technique which can been used to continuously monitor a variety of cell biological processes relevant to drug screening, such as changes in cell shape during apoptosis, the effects of drugs on cell migration and cell cycling events.10–12 In particular regard to cytotoxicity analysis, several reports have illustrated that ECIS monitoring of cell impedance provides sensitive measurements of chemical toxicity, and such ECIS measurements relate closely to estimations of cytotoxicity made by other established techniques.13,14
In this study an ECIS system was used for continuous, quantitative assessment of the cytotoxic responses of human HK2 renal tubular cells to several commonly used anticancer drugs. HK2 are an immortalized proximal tubular cell line derived from normal human kidneys and are an established cell model for nephrotoxicity testing in vitro.15,16 Four anticancer drugs were examined: cisplatin (DDP) and carboplatin (CDP) are both platinum containing compounds, 5-fluorouracil (5-FU) is a nucleoside analog, and cyclophosphamide (CTX) is a DNA alkylating agent. DDP has well-proven efficacy in the treatment of a wide range of tumors, although it has high nephrotoxicity which circumspects use clinically.17,18 It is also known to induce apoptosis or necrosis in kidney cells.19CDP, a second-generation drug developed from DDP, and has a better tolerance profile than DDP,20 whilst both 5-FU and CTX are rarely associated with acute nephrotoxicity.21 Clinically, both DDP and CDP (platinum-containing) drugs and have significantly higher cytotoxicity and nephrotoxicity than either CTX or 5-FU.22 Because of the dangers of DDP, it is also administered clinically at lower levels in combination with other weaker anticancer drugs such as 5-FU and CTX, to provide high anticancer toxicity, with a reduced degree of nephrotoxicity.23,24 These drugs were tested to determine whether the ECIS-based impedance system could accurately reflect their known cytotoxicity with HK2 renal tubular cellsin vitro. The cellular responses of the HK2 cells were also assayed in parallel studies using two standard cytoxicity methods, flow cytometry and the MTT assay. In addition, the effects of two antagonists of DDP cytotoxicity, sodium thiosulfate (STS)25 and glutathione (GSH),26 were also examined using the ECIS system.
2. Materials and methods
2.1 Materials and reagents
Pyrex glass wafers were purchased from Corning, Inc. (New York, NY). Polydimethylsiloxane (PDMS, Sylgard 184) was supplied by Dow Corning, Inc. (Midland, MI). DMEM/F12 medium, fetal bovine serum, trypsin/EDTA solution, penicillin, and streptomycin were purchased from Gibco BRL, Inc. (Grand Island, NY). Propidium iodide (PI) was purchased from Invitrogen (Carlsbad, CA). All of the other reagents were supplied by Sigma-Aldrich (St. Louis, MO).
2.2
Cell culture
HK2
cells were obtained from the American Type Culture Collection (ATCC, Manassas, VA). The cells were incubated at 37 °C in a humidified incubator containing a 5% CO2 atmosphere with DF12 (1
:
1 mixture of DMEM and F12 media) supplemented with 10% fetal bovine serum, 50 IU mL−1penicillin, and 50 μg mL−1streptomycin. HK2 Cells for seeding were prepared as a monodisperse cell suspension using standard tissue culture techniques with 0.25% trypsin containing 0.53 mM EDTA.
2.3 Impedance measurements and data processing methods
The ECIS system consisted of the ECIS device and an impedance measurement module. When cells adhered to the electrode surfaces of the chips or underwent morphological changes on the electrode surfaces, the impedance of the electrodes changed, and the signal was collected with a NI DAQ PCI-6110 multifunctional data acquisition card (National Instruments, Austin, TX) that was controlled by a LabVIEW™ (National Instruments) program. The eight channels of each chip were measured in division time, and four chips were measured simultaneously for each experiment. The impedance was measured, calculated, and displayed automatically by a computer in real time.
Details of the impedance measurements have previously been described.12 A 15 kΩ current limiting resistor was connected to the ECIS device in the impedance measurement module. Because impedance of the current limiting resistor was far larger than the impedance of the ECIS device (∼10 Ω), the current in the measurement circuit can be treated as constant. Therefore, the impedance of the ECIS device can be calculated by the voltage drop across the device, and the measuring range of our system could be adjusted by the limiting resistor.
2.4 Experimental procedures
Before the cells were seeded, the ECIS device was sterilized with 75% ethanol for 15 min, dried with N2, and irradiated with ultraviolet radiation for 15 min. DF12 medium (1 mL) was then added to the ECIS device and incubated at 37 °C for 20 min to record the background impedance value (Z0). Next, the cell suspension (500 μL, 1 × 105cells) was added into each cell culture chamber. After a 10 min equilibration, the device was placed into the incubator for cell culture and impedance sensing. The impedance (Zx) was measured every 5 min and displayed in real time by LABVIEW. The final impedance data were normalized as Zx/Z0 to reduce the effect of background impedance. The HK2 cells are randomly seeded in the chambers of the device for cytotoxicity testing, and the impedance measured is the average signal of the whole population. All fresh culture medium was pre-warmed at 37 °C for 10 min to decrease impedance variation due to medium exchanges. All solutions were filtered through a 0.22 μm membrane (Corning Inc., Beijing, China) before use.
The seeded the cells were adhered to the surface of the glass chip after a 5 h incubation, then fresh medium containing various concentrations of drug(s) were then added, replacing the initial seed cell culture medium. Each of the anticancer drugs was individually dissolved directly into the DF12 medium to avoid any additional cytotoxic effects of DMSO. The ECIS chips were incubated at 37 °C in a standard cell culture incubator and the impedance monitoring typically lasted about 48 h until the end of the experiments, with similar times and conditions for positive and negative groups. The exposure time of the HK2 cells to the different drugs varied slightly with the response of the cells and is shown in the relevant Figures.
The antidotes of DDP, GLU and STS were dissolved at various concentrations separately in DF12 medium containing 50 μM DDP. Negative control impedance was measured in DF12 media, while DF12 media containing 50 μM DDP served as positive control. To examine whether GLU or STS alone have measurable effects on the cell cultures, we directly dissolved GLU or STS in DF12 medium in the same concentration range as used in antidote tests, and compared impedance with the negative control ECIS experiments (DF12 media alone). The coefficient (IC) of inhibition for treatment with GSH or STS at different concentrations after 24 h treatment was calculated using the equation:
ZDDP refers to the cellular impedance at 24 h after 50 μM DDP treatment, while
ZC refers to the cellular impedance of the control.
2.5.1
Fluorescence labeling
.
Annexin V/PI staining assay was used to classify HK2 cells in the early apoptosis and late apoptosis/necrosis stages. For fluorescence imaging, 1 μM PI and 4 μM Annexin V-FITC were mixed in DF12 medium and directly added to the plate after the 24 h DDP treatment and then incubated for 30 min at room temperature. After washing with phosphate buffered saline (PBS), images were taken using a fluorescence microscope (DM-IRB, Leica, Solms, Germany) with a CCD camera (DP-71, Olympus, Tokyo, Japan).
2.5.2
Apoptosis analysis by flow cytometry.
After a 24 h treatment with DDP, the cells were trypsinized, washed with PBS, and collected by centrifugation at 300 g for 3 min. The cells were then resuspended in freshly prepared 4 μM Annexin V-FITC staining solution and incubated at 37 °C for 30 min in the dark. The stained cells were collected by centrifugation, resuspended in DF12 with 20% fetal bovine serum, and incubated briefly at 37 °C until flow cytometry analysis (FCA). The 1 μM PI solution was added 15 min before FCA. The excitation wavelength was 488 nm, and the emitted fluorescence of Annexin V-FITC and PI were collected by 525 and 575 nm band pass filters, respectively. At least 10
000 cells were analyzed per sample. The level of early apoptosis and late apoptosis or necrosis were determined as the percentage of Annexin V+/PI− and Annexin V+/PI+ cells, respectively.
2.5.3 MTT assays.
Some 100 μL of HK2 cell suspension (5 × 104cells mL−1) was seeded in each well of a 96-well culture plate. After 5 h incubation, the cells adhered to the cell culture well, and the medium was replaced with 100 μL fresh medium containing DDP at various concentrations. Cells were exposed to DDP for 4, 8, 12, 16, and 20 h. At the end of the exposure, 20 μL MTT solutions in PBS (5 mg mL−1, Sigma-Aldrich, Shanghai, China) were added to each well for a 4 h incubation. Next, 150 μl DMSO was added, and the culture plate shaken on an orbital shaker at room temperature for 30 min. Finally, the optical density of each sample was measured at 570 nm with a spectrophotometer (Model 680, Bio-Rad, Shanghai, China).
3. Results and discussion
3.1 Device design and fabrication
Fig. 1a shows the structure of the ECIS device, which is composed of four elements: a printed circuit board (PCB), a glass chip, a PDMS cell culture cavity, and a poly methyl methacrylate (PMMA) cover. The glass chip with interdigitated array electrodes for impedance sensing was fabricated on a Pyrex glass wafer using standard semiconductor fabrication techniques with a conductive layer of Ti/Au (30 nm/200 nm) and an insulation layer of Si3N4/SiO2 (400 nm/200 nm). The insulation layer was removed by reactive ion etching to expose the impedance sensing electrodes and bonding pads.
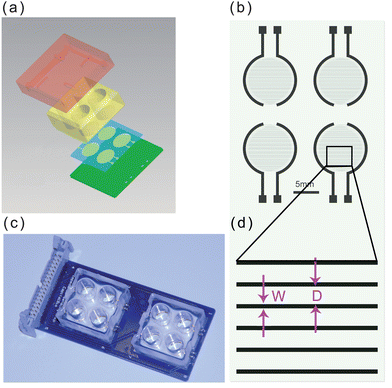 |
| Fig. 1 The design and fabrication of the ECIS device. (a) Illustration of the device structure. (b) Schematic drawing of the sensor electrodes arrays. (c) Fully assembled device consisting of two chips. (d) Enlarged view of (b); W = 20 μm, and D = 80 μm. | |
The fabricated glass chip was bonded to the PCB by a thermal ball bonder. PDMS cavities were cast using a PMMA mold to create environments for cell culture and impedance sensing. The PDMS pre-polymer was mixed in a 1
:
10 ratio of curing and base agent, degassed under vacuum, and then cured in an oven at 72 °C for 2 h to produce the elastomeric replicas. The cured PDMS cavity was covalently bonded to the glass chip by oxygen plasma treatment (FEMTO, Diener Plasma-Surface-Technology, Ebhausen, Germany). A PMMA cover was placed on the PDMS cavity to prevent bacterial contamination and reduce evaporation during cell culture.
A schematic diagram of the electrodes on the glass chip is shown in Fig. 1b. The width of the electrode is 20 μm, and the inter-electrode distance is 80 μm, as shown in Fig. 1d. The final encapsulation of the ECIS device is shown in Fig. 1c. The size of each PDMS cavity is 1 cm in height and 1 cm in diameter. There are eight groups of impedance sensing electrodes in the corresponding cavities for simultaneous monitoring of cell behavior under a number of conditions, or for experimental replication.
3.2 Real-time monitoring of the cellular response to DDP exposure
We used an ECIS system to monitor DDP-induced apoptosis in HK2 cells by measuring cellular impendence every 5 min over 48 h. In parallel, we compared the data obtained from the ECIS system with data from standard fluorescence labeling, FCA and MTT assays determined at several discrete time points to compare the evaluation of the cell apoptosis process monitored by the different techniques. A normalized impedance curve is shown in Fig. 2a. During treatment with 50 μM DDP, cellular impedance began to decline within 8 h, indicating apoptosis or necrosis. These observations were verified independently by fluorescent imaging, shown in Fig. 2b–d. Bright-field and fluorescent images are shown, at three time points: 5, 10, and 15 h after of continuous culture with 50 μM DDP, respectively. Dead and late-stage apoptotic cells stain with PI (red fluorescence), while early apoptotic cells are stained by Annexin V-FITC (green fluorescence). Five hours after treatment, no significant morphological changes were observed by FCA, and only rare cells were stained with PI/Annexin V-FITC. However, after 15 and 20 h of exposure to DDP, the HK2 cells displayed significant morphological changes, and the number of cells stained by PI/Annexin V-FITC increased markedly, indicating that an increasing number of cells were apoptotic. In contrast to the discrete time point measurements, Fig. 2a shows a decline in cellular impedance was clearly observable as early as 5 h after adding DDP. The decreasing impedance demonstrates that ECIS can measure the drug-induced changes to the cell membrane at early stages of the cytotoxic process. Obviously the standard fluorescence labeling, FCA and MTT assays could have been performed at additional time points, but at the cost of significantly increased effort and experimental replication. In contrast, the ECIS method provides multiple chambers for continuous parallel or replicate measurements on small numbers of cells. The continuous measurement can provides a better temporal resolution for toxicity profiles because the standard end point measurement may neglect the intermediate time events.
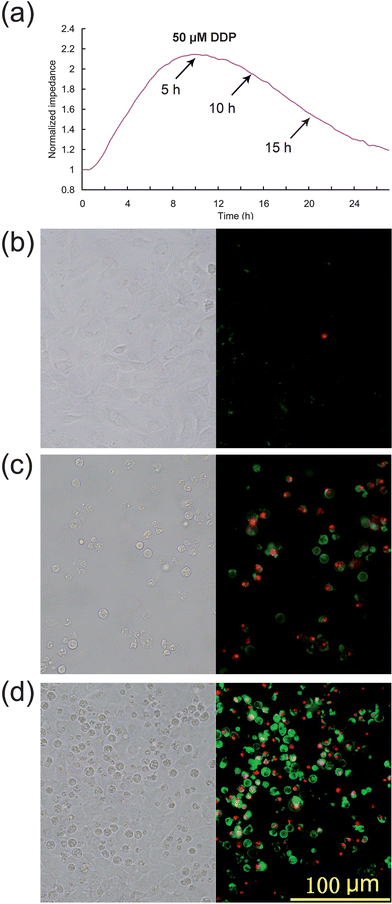 |
| Fig. 2 The independent confirmation of apoptosis by fluorescent labeling of cells. (a) Impedance variation for cisplatin-induced apoptosis. (b)–(d) Bright-field and fluorescence images of apoptosis induced (b) 5, (c) 10, and (d) 15 h after DDP treatment, respectively. | |
The therapeutic dose of DDP ranges from 20 mg m−2 to 120 mg m−2. Pharmacokinetics studies27,28 show that the peak concentrations of free plasma cisplatin are 4.7 ± 1.6 μM at dosage 30 mg m−2, 16 ± 8 μM at dosage 90 mg m−2. However renal tubular cells are exposed to far higher drug levels than that in the blood due to their role in concentrating and reabsorbing the glomerular filtrate.5 It is reported that the DDP concentration in proximal tubular epithelial cells is about 5 times the serum concentration.29 Therefore, the HK2 cells were exposed to DDP at concentrations ranging from 3 to 100 μM to study dose-dependent cell responses (Fig. 3a) which can be compared with discrete time point data obtained by FCA (Fig. 3b). The ECIS curves show that impedance is greatly reduced at DDP higher concentrations, indicating that that the speed of onset and severity of the cytotoxic effects increased with DDP concentration. In particular, the relative cellular impedance decreased to 1 within 24 h exposure to >50 μM DDP, indicating that no viable cells were attached to the chip surface. Flow cytometry produced similar results after the 24 h treatment, with the exception that in the 100 μM DDP treatment group the percentage of apoptotic and necrotic cells were unexpectedly decreased. This phenomenon is an artifact of the FCA assay: FCA calculates the percentage of dead or apoptotic cells in the entire population by relative fluorescence label. FCA counts cells with intact membranes, but most of the late stage apoptotic cells are extremely fragile and cell lysis occurs readily during sample preparation, altering the proportion of labeled cells assessed by FCA. Thus, using FCA the percentage of apoptotic cells appeared to decrease when the cells were exposed to drugs at very high concentrations. For this reason, ECIS may provide a more accurate estimation of apoptopic cell numbers than FCA, especially for highly toxic drugs, or drugs at high concentration, or at late stage apoptosis when many cells have elevated fragility.
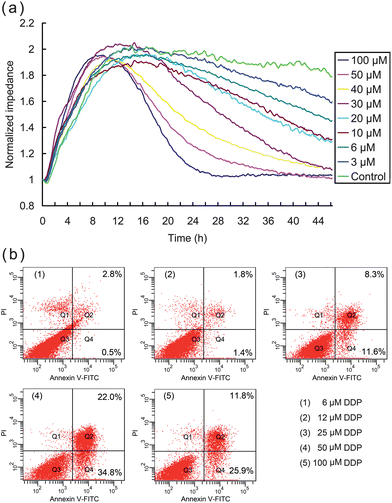 |
| Fig. 3 Impedance curves for DDP treatment with different dosages and validation by FCA. (a) ECIS impedance curves of HK2 cells in the presence of different concentrations of DDP. (b) Apoptosis of HK2 cells determined by FC after 24 h exposure to DDP at the indicated concentrations. | |
3.3 Correlation analysis between the impedance data and MTT assays
MTT assays were performed to determine the time-dependent and dose-dependent cytotoxicity of DDP and provide a comparison of the impedance results with a standard quantitative cytotoxicity assessment. The viability of HK2 cells exposed to six different concentrations of DDP was determined after various exposure times. The MTT assays and a correlation analysis between the MTT assays and the impedance data are presented in Fig. 4. For dose-dependent cytotoxicity comparison, the MTT values were normalized at 1 by establishing the control value (without DDP treatment). Fig. 4a shows the MTT values of HK2 cells exposed to different concentrations of drug for 24 h, and the correlation analysis between the MTT assays and the cellular impedance is shown in Fig. 4b. The high correlation efficiency (0.96) indicates a close agreement between the standard MTT assay and the ECIS system. For the time-dependent measurements, HK2 cells were exposed to 50 μM DDP, and MTT assays were performed at different time-points after treatment. Similar to the dose-dependent tests, we normalized the MTT values by setting the 0-h value as 1 (Fig. 4c) and performed correlation analysis (Fig. 4d). The decrease in cell viability with increasing treatment time or DDP concentration correlated well with the decreasing trend of cellular impedance. In Fig. 4c, it is clear that the measured MTT values did not decline immediately after DDP was added, but rather increased slightly until 4 h time point, and then decreased more markedly after longer exposure times. These results can be correlated with the cellular impedance changes shown in Fig. 2a, which began to decline approximately 5 h after the introduction of the drug. Thus, the effects of DDP are not immediately manifest with either impedance or MTT, and both assays register a commencement of a decline at approximately the same time points, which indicates that ECIS can directly monitor some of the MTT identified cell changes as they begin to occur.
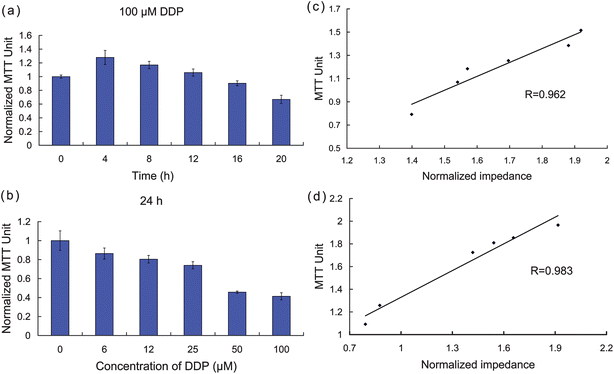 |
| Fig. 4 Confirmation of apoptosis by independent MTT assays. (a)–(b) MTT values measured with DDP at different concentrations and exposure times. (c)–(d) Correlation analysis between MTT assays and cellular impedance. | |
3.4 ECIS profiling of the cytotoxicity of CDP, 5-FU, and CTX
We independently assessed the cytotoxicity of CDP, 5-FU, and CTX using the ECIS system. The impedance response curves shown in Fig. 5 demonstrate the continuous changes of cell status in response to different doses of CDP, 5-FU, and CTX (from top to bottom, respectively). These data demonstrate clearly that CDP produces evident cytotoxicity at concentrations >1 mM, CTX demonstrated some cytotoxicity at >6 mM, while 5-FU demonstrated no obvious cytotoxicity at concentrations <6 mM, and only commenced to display cell toxic effects at higher concentrations of 25–50 mM. High anti-tumorogenic doses of 5-FU and CTX are well tolerated clinically,23,24,30 and the ECIS impedance measurements of HK2 cells showed no obvious changes in cellular attachment at similar drug concentrations over the period of study, which could be inferred that the ECIS indicates low toxicity in agreement with clinical observations.
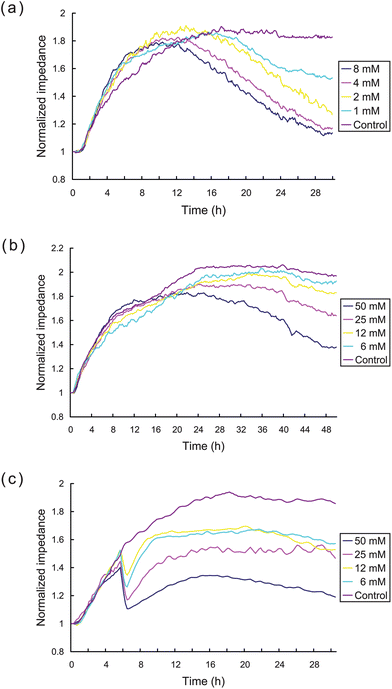 |
| Fig. 5 ECIS measurement of the cytotoxicity of CDP, 5-FU, and CTX. Impedance curves of HK2 cells exposed to different indicated concentrations of (a) CDP, (b) 5-FU, and (c) CTX, respectively. | |
It is clear that the impedance measurements with CTX displayed a different cytotoxic time-response pattern compared to the patterns seen with the other drugs (see Fig. 5c). The cellular impedance declined dramatically about 4.0–4.5 h after exposure to all concentrations CTX tested and then recovered slowly thereafter to a level which diminished with increasing concentrations. The dramatic loss of impedance at a particular time point after exposure indicates an acute effect of CTX on cell adhesion or membrane impedance. These drug specific observations illustrate the advantage of the continuous monitoring provided by ECIS measurements, as the standard end point measurement would not readily observe intermediate time events such as dramatic loss of impedance induced by CTX or the subsequent recovery of the HK2 cells after this initial loss.
3.5 Evaluation of drug antidotes by the ECIS system
Several routes might be undertaken to improve chemotherapy outcomes while minimizing the side effects of the treatment. One route is to attempt to identify and develop effective chemotherapeutic drugs with minimal adverse side effects. Alternatively, antagonists to some of the undesirable cellular events might be able to mitigate the side effects of current highly toxic anticancer drugs.
We assessed the cellular impedance effects of two common DDP detoxification compounds, STS and GSH using the ECIS system with HK2 cells, shown in Fig. 6. STS is a common antidote for heavy metal poisoning25 and GSH is an antioxidant which can alleviate cytotoxicity.26 The effects of GSH and STS on HK2 cells were initially evaluated (data not shown) and concentrations found that have no cytotoxic effects were used in these subsequent ECIS experiments. GSH and STS in DF12 medium containing 50 μM DDP, and DF12 with GSH or STS (max test concentrations) were used as positive and negative controls, respectively. As shown in Fig. 6a, the cells grown with a series of increasing concentrations of STS and 50 μM DDP had higher cellular impedance than cells exposed with 50 μM DDP alone, indicating that STS has a dose-dependent inhibitory effect on the level of DDP-induced apoptosis. In Fig. 6a, cells grown with a series of increasing concentrations of GSH and 50 μM DDP showed a marked difference response between GSH at 2 mM or higher to responses of cells grown at concentrations of 1mM GSH or lower, suggesting a threshold concentration of GSH is necessary to abrogate the effects of DDP (Fig. 6c). In contrast, HK2 cells treated with increasing concentrations of STS (Fig. 6b) showed a more dose-dependent inhibition coefficient profile (Fig. 6d). The impedance of HK2 cells treated with 0.5 mM STS and 50 μM DDP, or 2 mM GSH and 50 μM DDP were similar to that of the control group, and overall the inhibitory (anti-cytoxic) effects of both antagonists decline at their concentration deceased. While the ECIS observations confirm the effects of these two antidotes on DDP induced cytotoxicity reported by others,25,26 ECIS readily illustrates differences in the dose-dependent inhibition coefficient profiles of the two antidote compounds.
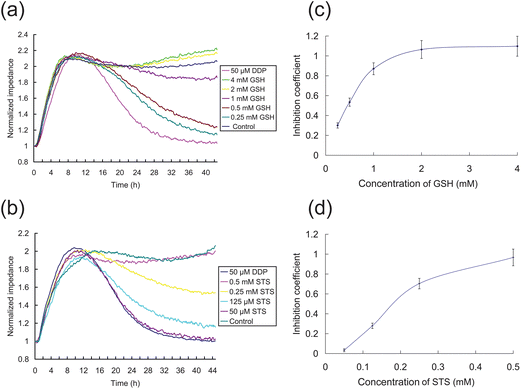 |
| Fig. 6 ECIS measurement of the effects of antagonists on DPP-induced toxicity of HK2 cells. (a)–(b) Impedance curves of detoxification by GSH and STS, respectively. The 50 μM DDP group in DF12 medium containing 50 μM DDP, and the control group is in DF12 medium containing 4 mM GSH (a) or 0.5 mM STS (b). The other groups are dissolved in the DF12 medium with 50 μM DDP. The GSH/STS concentrations are indicated. (c–d) The inhibition coefficients of GSH and STS after 24 h treatment are shown, respectively. | |
Our work demonstrates that an ECIS-based system can be used effectively for in vitro cytotoxicity testing and for the evaluation of antidote compounds in an in vitro human renal cell model assay for nephrotoxicity assessment. Furthermore, because combination drug therapy is currently used quite commonly in cancer treatments,23,24,30 it is increasingly necessary to explore a large number of potential drug-drug interactions between various active compounds. With ECRIS providing direct, continuous measurements of cell status, and being non-invasive/destructive as well as providing quantitative measurements, ECIS systems may prove to be a useful tool for the examination with other model cell systems of other types of drug–drug interactions, as well as for determination of the cellular effects of single test compounds.
4. Conclusions
In order to assess the cytotoxicity of anticancer drugs, we have developed an ECIS system for in vitro cellular assay of nephrotoxicity. This system was demonstrated to be capable of testing the toxic and antitoxic effects of clinically in-use drugs (single drug, or a combination of drugs). By comparing the measured impedance signals with the results of FCA and MTT analyses, we found that the ECIS results are consistent with the standard assays and show responses comparable to clinical reports. These findings suggest that the ECIS can be used to independently assess the nephrotoxicity of anticancer drugsin vitro. Moreover, ECIS profiles of GSH and STS protection from DDP cytotoxicity exposed different HK2 cell responses, providing insight into their different dose-dependence actions of the two compounds. Such assays with the ECIS system demonstrate its broad utility to rapidly and quantitatively assess anticancer drugs and to examine the activities of their antagonists and agonists in model in vitrocell nephrotoxicity assays.
The cell-based ECIS system allows convenient multiplication of identical test chambers and electrode sensors. The electrode parameters are identical and highly multiplexed and thus generate highly replicable and statistically reliable data which is collected automatically by computer: these various features make ECIS very amenable for high throughput screening uses, and hence it may find great use in applications such as drug toxicity testing. ECIS can also be used to assess the effects other, non-cancer drugs on the state of cell health and activities, as the monitoring of membrane electrophysiological status does not interfere with normal cell activity.
Acknowledgements
This study was supported by a grant from the National Hi-Tech Program of China (No. 2006AA020701).
References
- R. Bellomo, Curr. Opin. Crit. Care, 2006, 12, 557–560 CrossRef.
- H. S. Kohli, M. C. Bhaskaran, T. Muthukumar, K. Thennarasu, K. Sud, V. Jha, K. L. Gupta and V. Sakhuja, Nephrol., Dial., Transplant., 2000, 15, 212–217 CrossRef CAS.
- V. Launay-Vacher, S. Oudard, N. Janus, J. Gligorov, X. Pourrat, O. Rixe, J. F. Morere, P. Beuzeboc and G. Deray, Cancer, 2007, 110, 1376–1384 CrossRef CAS.
- R. A. Zager, Semin. Nephrol., 1997, 17, 3–14 CAS.
- M. A. Perazella, Expert Opin. Drug Saf., 2005, 4, 689–706 CrossRef CAS.
- N. Nakashima-Kamimura, T. Mori, I. Ohsawa, S. Asoh and S. Ohta, Cancer Chemother. Pharmacol., 2009, 64, 753–761 CrossRef CAS.
- I. Valentin, M. Philippe, J. C. Lhuguenot and M. C. Chagnon, Toxicology, 2001, 158, 127–139 CrossRef CAS.
- A. P. Li, C. Lu, J. A. Brent, C. Pham, A. Fackett, C. E. Ruegg and P. M. Siber, Chem.-Biol. Interact., 1999, 121, 17–35 CrossRef CAS.
- F. Mazzotti, E. Sabbioni, J. Ponti, M. Ghiani, S. Fortaner and G. L. Rossi, Altern. Lab. Anim., 2002, 30, 209–217 CAS.
- S. Arndt, J. Seebach, K. Psathaki, H. J. Galla and J. Wegener, Biosens. Bioelectron., 2004, 19, 583–594 CrossRef CAS.
- L. Wang, J. Zhu, C. Deng, W. L. Xing and J. Cheng, Lab Chip, 2008, 8, 872–878 RSC.
- L. Wang, L. Wang, H. Yin, W. Xing, Z. Yu, M. Guo and J. Cheng, Biosens. Bioelectron., 2010, 25, 990–995 CrossRef CAS.
- D. Opp, B. Wafula, J. Lim, E. Huang, J. C. Lo and C. M. Lo, Biosens. Bioelectron., 2009, 24, 2625–2629 CrossRef CAS.
- J. Z. Xing, L. Zhu, S. Gabos and L. Xie, Toxicol. in Vitro, 2006, 20, 995–1004 CrossRef CAS.
- R. A. Zager, C. M. Johnson, S. Lund and J. Randolph-Habecker, Am. J. Physiol.: Renal Physiol., 2007, 292, 304–312 CrossRef.
- J. Li, L. Zhang, Z. Jiang, B. Shu, F. Li, Q. Bao and L. Zhang, Toxicol. in Vitro, 2010, 24, 1092–1097 CrossRef CAS.
- I. Arany and R. L. Safirstein, Semin. Nephrol., 2003, 23, 460–464 CrossRef CAS.
- X. Yao, K. Panichpisal, N. Kurtzman and K. Nugent, Am. J. Med. Sci., 2007, 334, 115–124 CrossRef.
- R. Safirstein, J. Winston, M. Goldstein, D. Moel and S. Dikman, Am. J. Kidney Dis., 1986, 8, 356–367 CAS.
- A. H. Lau, Kidney Int., 1999, 56, 1295–1298 CrossRef CAS.
- N. Pinto, S. M. Ludeman and M. E. Dolan, Pharmacogenomics, 2009, 10, 1897–1903 CrossRef CAS.
- M. J. Mckeage, Drug Saf., 1995, 13, 228–244 CrossRef CAS.
- Y. F. Li, S. Fu, W. Hu, J. H. Liu, K. W. Finkel, D. M. Gershenson and J. J. Kavanagh, Int. J. Gynecol. Cancer, 2007, 17, 739–763 CrossRef CAS.
- D. H. Moore, F. Valea, L. A. Walton, J. Soper, D. Clarke-Pearson and W. C. Jr. Fowler, Gynecol. Oncol., 1995, 59, 267–272 CrossRef CAS.
- S. Onohara, H. Kobayashi, Y. Itoh and S. Shinohara, Acta Radiol., 1988, 29, 197–202 CrossRef CAS.
- J. B. Mitchell and A. Russo, Br. J. Cancer Suppl., 1987, 8, 96–104 CAS.
- J. Goodisman and A. K. Souid, J. Clin. Pharmacol., 2006, 46, 443–448 CrossRef CAS.
- M. J. Li, L. F. Chi and G. P. Tang, J. Zhejiang Univ. (Medicine Edition), 2001, 34, 150–152 Search PubMed.
- M. K. Kuhlmann, G. Burkhardt and H. Kohler, Nephrol., Dial., Transplant., 1997, 12, 2478–2480 CrossRef CAS.
- R. Wang, Q. Fan and Z. Wang, Zhonghua Zhong Liu Za Zhi, 1995, 17, 362–364 CAS.
Footnotes |
† This article is part of a web theme in Analyst and Analytical Methods on Future Electroanalytical Developments, highlighting important developments and novel applications. Also in this theme is work presented at the Eirelec 2011 meeting, dedicated to Professor Malcolm Smyth on the occasion of his 60th birthday. |
‡ These authors contributed equally to this work. |
|
This journal is © The Royal Society of Chemistry 2012 |
Click here to see how this site uses Cookies. View our privacy policy here.