DOI:
10.1039/C1RA01060C
(Paper)
RSC Adv., 2012,
2, 1502-1507
Glucose-triggered co-enzymatic hydrogelation of aqueous polymer solutions
Received
10th November 2011
, Accepted 11th November 2011
First published on 20th December 2011
Abstract
H2O2 generation by glucose oxidase (GOx)-catalysed oxidation of glucose was used to supply H2O2 in the horseradish peroxidase (HRP)-catalysed hydrogelation of aqueous solutions of polymers bearing phenolic hydroxyl (PH) moieties. The feasibility of the glucose-triggered hydrogelation was evaluated using an alginate derivative bearing PH moieties (Alg-PH). The initial rate of cross-link formation between the PH moieties and the gelation time of the Alg-PH aqueous solution increased and decreased, respectively, with increasing GOx and glucose concentrations. Hydrogels with comparable cross-link densities and gelation times to those formed using a conventional direct H2O2 addition system could be obtained by altering these parameters. The mechanical properties of the resultant hydrogels were also controllable. The hydrogel obtained from a 1.1% (w/v) Alg-PH solution at 1 u mL−1 HRP, 0.8 u mL−1 GOx, and 4.6 mM glucose showed repulsion forces toward compression more than four times those shown by hydrogels obtained with 1 u mL−1 HRP and 4.6 mM H2O2. These results indicate that the glucose-triggered hydrogelation system has potential for a wide range of biomedical applications such as fabricating substrates for tissue engineering, carriers for drug and protein delivery systems, and wound dressings.
Introduction
Enzymatic cross-linking has attracted attention as a possible environmentally friendly route for polymerizing molecules.1,2 The mild reaction conditions of enzymes are attractive for biomedical and pharmaceutical applications such as encapsulations of biomacromolecules,3 use in living cells,4–6 and drugs in hydrogels. Hydrogels are composed of physically or chemically cross-linked polymeric networks that can absorb and retain a considerable amount of water with maintenance of shape. As a result of this feature, hydrogels provide a suitable environment for biomacromolecules and living cells. In addition, the cross-linked polymer network allows sustained release of enclosed molecules, e.g.drugs.7
Application of horseradish peroxidase (HRP)-catalysed hydrogelation systems in biomedical fields has been studied intensely since the report by Kurisawa et al.8 of the subcutaneous gelation in mice of aqueous solutions containing a hyaluronic acid derivative possessing phenolic hydroxyl (PH) moieties. The system has been reported to be useful for drug delivery,3 tissue repairing,9 and tissue engineering6,10,11 because hydrogelation occurs at the injected site rapidly enough to prevent uncontrolled diffusion of gel precursors, drug molecules, and cells to the surrounding tissues. HRP catalyses the oxidation of donors using H2O2, resulting in polyphenols linked at the aromatic ring by C–C and C–O coupling between PH moieties. As far as we know, direct addition of H2O2 molecules dissolved in aqueous solution into the reaction system has been the only way to supply the H2O2 necessary for achieving enzymatic hydrogelation.8,12–14
In the present study, we indirectly supplied H2O2 to the HRP-catalysed hydrogelation system using an enzymatic oxidation–reduction reaction. We used glucose oxidase (GOx) for this purpose. In living organisms, H2O2 is generated through a variety of enzymatic oxidation–reduction reactions, including GOx-catalysed reactions. GOx is an oxidoreductase that catalyses the oxidation of glucose to H2O2 and glucono-δ-lactone. This enzyme, coupled to a peroxidase-catalysed reaction that colourimetrically visualizes the H2O2 formed, has been used for determining glucose in body fluids, foods, and agricultural products. These previous applications also indicate that the glucose originally existing in living organisms can be a substrate for HRP-catalysed hydrogelation. The main motivation of the present study is to assess the features of the glucose-triggered co-enzymatic hydrogelation of aqueous solutions of polymers bearing PH moieties using GOx and HRP, as an alternative process to hydrogelation by HRP triggered by directly added H2O2 aqueous solution. The point of using the GOx-catalysed reaction for supplying H2O2 rather than the conventional method of adding H2O2 directly as an aqueous solution is that a certain amount of H2O2 is supplied gradually as the enzymatic reaction progresses. In this paper, the two methods of supplying H2O2 to the hydrogelation process, i.e. by a GOx-catalysed reaction converting glucose to H2O2 and by direct addition of H2O2, were designated as “indirect H2O2 addition” and “direct H2O2 addition”, respectively. It is expected that a larger amount of the total H2O2 can be supplied to the reaction system with less inactivation of HRP using the indirect H2O2 addition method. It is known that exposure to a high concentration of aqueous H2O2 inactivates HRP.15,16 Furthermore, it has been reported that the gelation profiles of polymeric solutions and the mechanical properties of the resultant hydrogels were governed by the concentration of H2O2 added directly as aqueous solutions as well as the concentrations of HRP and polymers.12,17–19 Based on these previous reports, we predicted that the hydrogelation behaviours and characteristics of the hydrogels obtained by direct addition of H2O2 and those obtained by supplying H2O2 through a GOx-catalysed reaction would differ from each other, even if conditions resulting in the supply of the same amounts of H2O2 were used. In this paper, we first demonstrate the dependence of the cross-linking profiles of the PH moieties on the concentrations of HRP, GOx, and glucose. Then we demonstrate the effects of these conditions on the time necessary for gelation and on the mechanical properties of the resultant hydrogels. Throughout these experiments, we compared these characteristics with the hydrogelation and resultant hydrogels obtained by direct H2O2 addition. In these comparisons, the H2O2 concentrations in individual experiments were decided based on the stoichiometry that one H2O2 molecule is obtained from one glucose molecule in a GOx-catalysed reaction.20 A sodium alginate derivative bearing PH moieties (Alg-PH)19 was used as a model polymer cross-linkable by the HRP-catalysed reaction.
Experimental
Materials
GOx from Aspergillus niger (241 u mg−1) was purchased from SERVA Electrophoresis GmbH (Heidelberg, Germany). HRP (150 u mg−1), H2O2 aqueous solution (30% (w/w)), and D(+)-glucose were purchased from Wako Pure Chemical Industries (Osaka, Japan). I-1G sodium alginate with a high content of guluronic acid and molecular weight of 70
000 was purchased from Kimica (Tokyo, Japan). Alg-PH containing 2 PH moieties per 100 repeat units of uronic acid was synthesized, based on a previously reported method,19 by conjugation with tyramine hydrochloride (Sigma, St. Louis, MO, USA) using aqueous-phase carbodiimide activation chemistry using 1-ethyl-3-(3-dimethylaminopropyl)carbodiimide (Peptide Institute, Osaka, Japan) and N-hydroxysulfosuccinimide (Wako Pure Chemical Industries).
Dityrosine cross-link formation
The degrees of cross-linking between PH moieties were measured by determining the fluorescence intensity specific to dityrosine cross-links (excitation 315 nm, emission 410 nm)20 using a fluorescence plate reader (SpectraMax Gemini EM, Molecular Devices Inc., Sunnyvale, CA, USA). In a preliminary study, we confirmed that the fluorescence intensity is proportional to the density of dityrosine. An aqueous solution of 1.5% (w/v) Alg-PH, 500 μL, was poured into a 5 mL plastic tube. Then an aqueous solution (111 μL) containing HRP and GOx was added to the plastic tube. After mixing well, 56 μL of a glucose aqueous solution were added. Immediately after mixing using a vortex mixer, 200 μL of the resultant mixture solution were transferred to a 96-well plate to be read by the fluorescence plate reader. The time from the addition of glucose solution to setting the 96-well plate for determining the fluorescence intensity was 30 ± 5 s for all specimens. In the case using H2O2 solution, a solution containing only HRP, but the same amount (111 μL) was added instead of the solution containing both HRP and GOx. The transitions of the fluorescence intensities of each specimen were measured for 280 min. Initial fluorescence intensity increase rates (IFIRs) were calculated from the transitions of the intensity attributed to dityrosine cross-links during the first 2 min of reaction. The concentrations of Alg-PH in the final solutions were fixed at 1.1% (w/v). To determine the effects of the operating parameters, the concentration ranges of HRP, GOx, and glucose were varied as follows: HRP: 1–15 u mL−1; GOx: 0.8–8.3 u mL−1; and glucose and H2O2: 4.6–92.5 mM. As controls, solutions containing HRP and H2O2 were used instead of the HRP and GOx mixture solution and glucose solution, respectively. Unless otherwise noted, all experiments were performed at room temperature.
The gelation time of the Alg-PH aqueous solution was determined based on a previously reported method.17 Briefly, 1.5% (w/v) Alg-PH aqueous solution was poured into a 24-well plate at 450 μL/well (1.56 cm in diameter). Subsequently, 100 μL of the solution containing HRP and GOx was poured into each well and stirred gently using a magnetic stirrer bar (10 mm in length, 4 mm in diameter). Then, 50 μL of glucose solution was poured into the well during stirring. The formation of a gel state was signalled when the magnetic stirring was hindered and the surface of the solution swelled. The examined ranges of the parameters were as follows: HRP = 1–15 u mL−1, GOx = 0.8–8.3 u mL−1, and glucose = 1.4–46.3 mM. As controls, the solutions containing HRP and H2O2 were used instead of the HRP and GOx mixture solution and glucose solution, respectively.
Mechanical properties
The mechanical properties of the Alg-PH hydrogels were determined for the specimens obtained under conditions giving obviously different features with respect to cross-link formation and gelation time. The measurements were performed based on a previously reported method using a table-top materials tester (EZ-test-5N, Shimadzu, Kyoto, Japan) equipped with a compression probe 10 mm in diameter.17 Briefly, 1.5% (w/v) Alg-PH aqueous solution was poured into a 24-well plate at 1.5 mL/well. After adding 0.32 mL of the solution containing HRP and GOx into each well, 0.16 mL of glucose solution was added. After stirring using a magnetic stirrer bar for 10 s, the stirring bar was removed from the well. The 24-well plate containing the hydrogels was placed in the atmosphere at 37 °C. Compression–repulsion force profiles of the resultant gels were determined at a compression rate of 5 mm min−1. The effect of the standing period after mixing the chemicals for gelation was evaluated for the gels after 1 h and 24 h of standing. In the experiments to determine the effect of glucose and GOx concentrations, the gels after 24 h of standing were used. The examined ranges of the parameters were as follows: HRP: 1 u mL−1; GOx: 0.8–8.3 u mL−1; and glucose: 4.6–46.3 mM. Because of a technical problem, the compression–repulsion force profiles of the specimens obtained by direct addition of 46.2 mM and 92.5 mM H2O2 were too weak to measure, so we used the specimen obtained by direct addition of 4.6 mM H2O2 at 1 u mL−1 HRP as a control.
Results
Co-enzymatic cross-link formation
As shown in Fig. 1a, there is the possibility of hydrogelation of aqueous solutions of polymers bearing PH moieties by the co-enzymatic process triggered by glucose. This was first evaluated by mixing a Alg-PH solution with two or three of GOx, HRP, and glucose. Only the mixture containing GOx, HRP, and glucose yielded a hydrogel in a similar way to the control system containing HRP and H2O2 (Fig. 1b).
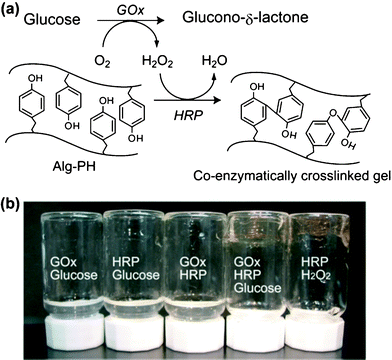 |
| Fig. 1 (a) Scheme for the glucose-triggered hydrogelation of Alg-PH by co-enzymatic GOx and HRP reaction. (b) Photograph of upturned vessels containing solutions containing GOx (0.8 u mL−1), HRP (15 u mL−1), glucose (4.6 mM), or H2O2 (4.6 mM), with Alg-PH solution (0.75%). The components contained in each vessel are shown in the photograph. | |
Based on these results, the dependencies of cross-link formation between PH moieties on GOx, HRP, and glucose concentrations were evaluated by measuring the change of the fluorescence intensity with time attributed to dityrosine cross-links. Fig. 2a shows IFIRs calculated from the increase in the fluorescence intensities during the first 2 min after mixing. The trends observed for the IFIRs were independent of HRP concentration in the examined range of 1–15 u mL−1 (Nos. 1–3) at fixed concentrations of GOx (0.8 u mL−1) and glucose (4.6 mM). In contrast, GOx and glucose concentrations strongly influenced the IFIR: the IFIRs increased 5.9 and 7.2 times when the concentration of GOx increased from 0.8 u mL−1 (No. 3) to 8.3 u mL−1 (No. 5), and when that of glucose increased from 4.6 mM (No. 3) to 46.2 mM (No. 6), i.e. a 10-fold increase in concentration in each case.
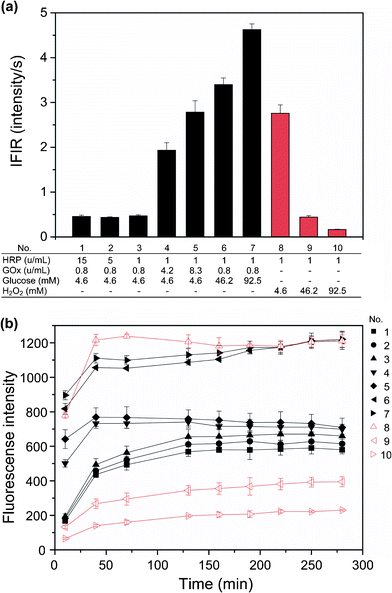 |
| Fig. 2 Effects of HRP, GOx, glucose, and H2O2 concentrations on (a) initial fluorescence intensity increase rate (IFIR), and (b) transition of fluorescence intensity for 280 min after mixing chemicals for hydrogelation. The run numbers shown in (b) are the same as those in (a). Alg-PH concentration was fixed at 1.1% (w/v) in all specimens. Bars: standard deviations (n = 3). | |
In the cases of direct H2O2 addition, the IFIRs decreased with increasing H2O2 concentration (Nos. 8–10).
Fig. 2b shows the transitions of the fluorescence intensities under the same conditions as shown in Fig. 2a for an experimental time of 280 min. Under fixed concentrations of GOx (0.8 u mL−1) and HRP (1 u mL−1), the times required to reach plateau fluorescence intensities decreased with increasing GOx (Nos. 3–5) and glucose (Nos. 3, 6, and 7) concentrations. Comparing the runs with a 4.6 mM glucose addition (Nos. 1–5) and those with an addition of the same amount of H2O2 (No. 8), the highest fluorescence intensity, indicating the largest amount of dityrosine cross-links, was detected in the direct H2O2 addition run. The plateau fluorescence intensities attributed to dityrosine cross-links increased with increasing glucose concentration (Nos. 3, 6, and 7), but with decreasing H2O2 concentration (Nos. 8–10). The trend was the same as that observed for the IFIRs. With respect to the effect of HRP concentration, the periods of time required for reaching a plateau were almost the same for 1 u mL−1, 5 u mL−1, and 15 u mL−1 HRP, but the plateau fluorescence intensities slightly decreased with increasing HRP concentration for fixed concentrations of GOx and glucose (Nos. 1–3).
Fig. 3a shows the effects of glucose and HRP concentrations on the gelation times of the Alg-PH mixture solutions (fixed at 1.1% (w/v)) obtained by supplying H2O2 indirectly using GOx (fixed at 0.8 u mL−1). The gelation times were independent of HRP concentration but dependent on glucose concentration: the gelation times decreased from around 800 s to 60 s with increasing glucose concentration from 1.4 mM to 46.3 mM. In contrast, the gelation time increased with increasing H2O2 concentration in the direct H2O2 addition process containing 1 u mL−1 HRP from 90 s (1.4 mM H2O2) to 426 s (13.8 mM). A further increase in H2O2 concentration to 42.6 mM did not give hydrogelation within 30 min.
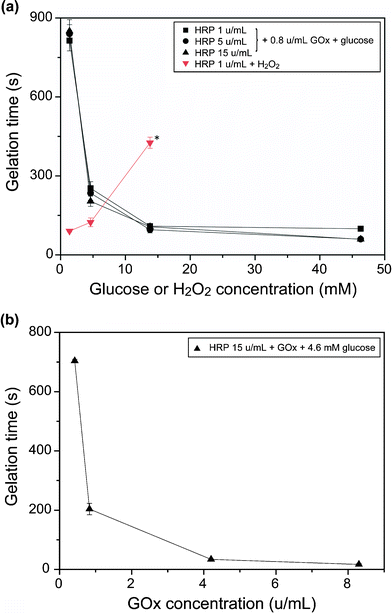 |
| Fig. 3 Effects of (a) HRP and glucose concentrations at fixed concentration of GOx (0.8 u mL−1), and (b) GOx concentration at fixed concentrations of HRP (15 u mL−1) and glucose (4.6 mM) on gelation times of 1.1% (w/v) Alg-PH solution. (a) The gelation times in the runs of direct H2O2 addition were measured as controls. *Gelation did not occur within 30 min of experiment when H2O2 concentration increased to 46.2 mM. Bars: standard deviations (n = 3). | |
Fig. 3b shows the effect of GOx concentration on the gelation time using fixed concentrations of HRP (15 u mL−1) and glucose (4.6 mM). The gelation time decreased with increasing GOx concentration. The shortest gelation time was 17 s at 8.3 u mL−1 GOx.
Mechanical properties
We evaluated the mechanical properties of the hydrogels by measuring the repulsion force toward compression. Fig. 4a shows the effect of the standing period after mixing the chemicals for gelation on the compression–repulsion force profiles of the resultant hydrogels. The profiles detected for the specimen obtained by direct addition of 4.6 mM H2O2 at 1 u mL−1 HRP were almost the same at 1 h and 24 h. In contrast, the repulsion forces detected for the specimen obtained by addition of 4.6 mM glucose and the same amount of HRP, i.e. 1 u mL−1, at each compression point showed a more than six-fold elevation at 24 h compared with those detected at 1 h. The repulsion force detected for the specimen obtained by the co-enzymatic reaction at 2 mm compression was six times larger than that obtained by direct H2O2 addition. Next, we evaluated the effects of glucose (Fig. 4b) and GOx (Fig. 4c) concentrations. The trends were such that the repulsion forces toward compression decreased with increasing concentrations of glucose and GOx.
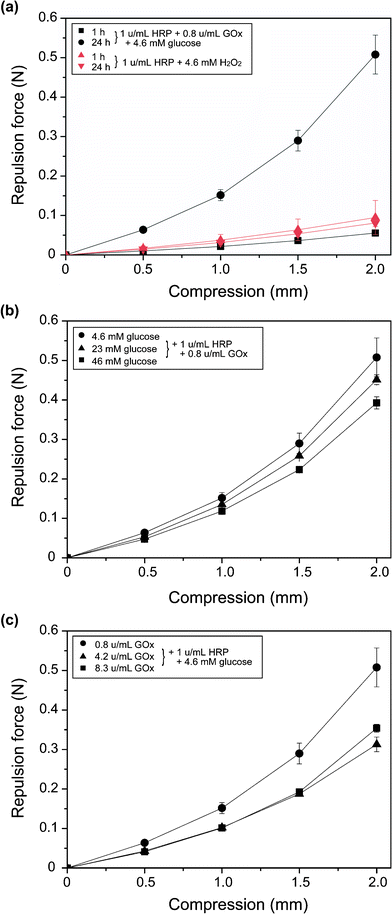 |
| Fig. 4 Effect of (a) standing time after mixing chemicals for hydrogelation, (b) glucose concentration, and (c) GOx concentration on compression–repulsion force profiles of each specimen obtained from 1.1% (w/v) Alg-PH solution. The hydrogels in (b) and (c) were evaluated at 24 h of standing after mixing chemicals for hydrogelation. Bars: standard deviations (n = 5). | |
Discussion
The focus of our research was to assess the features of the hydrogelation of aqueous solutions of polymers bearing PH moieties by co-enzymatic reactions of GOx and HRP triggered by glucose addition. To accomplish this objective, we determined the factors affecting cross-link formation and hydrogelation, and the mechanical properties of the resultant hydrogels. The information obtained from the shorter time experiments, IFIR (Fig. 2a) and gelation time (Fig. 3), and longer time experiments, plateau fluorescence intensity attributed to dityrosine cross-links (Fig. 2b) and mechanical property (Fig. 4), are useful for understanding the possibility of in situgelation and properties of the resultant hydrogels, respectively. In addition, we compared the features with those of conventional HRP-catalysed hydrogelation by direct H2O2 addition.8,13,14
Cross-link formation and hydrogelation
The first stage of our investigation was evaluation of the behaviours of glucose-triggered cross-link formation between PH moieties, including hydrogelation. The main trends were that the initial rate of cross-link formation and gelation time increased and decreased, respectively, with increasing GOx and glucose concentrations (Fig. 2a, 3). These trends can be explained by the general theory of enzyme kinetics with no inhibition, in which the initial reaction rate increases with increasing enzyme and substrate concentrations. With respect to the effect of HRP concentration, no obvious differences in IFIRs and gelation times were detected for fixed concentrations of 0.8 u mL−1 GOx and 4.6 mM glucose (Fig. 2a, Nos. 1–3, Fig. 3a); this indicates that the GOx-catalysed reaction was a rate-limiting step under these conditions. The reason for the slight decrease in the plateau fluorescence intensities with increasing HRP concentration under the conditions for Nos. 1–3 in Fig. 2b is not yet clear. A possible explanation is that there is an increase in cross-link formation between the activated PH moieties in the Alg-PH and the amino groups on HRP with increasing HRP concentration, resulting in decreased cross-link formation between the PH moieties. It is known that phenols activated by enzymatic oxidation form cross-links with amino groups on proteins.21,22
The distinct difference between the systems using glucose and H2O2 as the trigger molecules for hydrogelation was that cross-link formation was enhanced and hindered, respectively, by increasing the concentration of the trigger molecule. The increase in gelation time with increasing concentration of directly added H2O2 has been explained by inactivation of HRP by H2O2.18,19 The inactivation of HRP results from attack by free-radical intermediates generated by HRP-catalysed reactions and excess H2O2.15,16,23 In view of the dependence of the inactivation of HRP on H2O2 concentration, the opposite trend observed in the glucose-triggered hydrogelation system was interpreted as being a consequence of less accumulation of H2O2 in the reaction system. In the glucose-triggered system, H2O2 molecules are gradually generated from glucose by GOx, and sequentially consumed by HRP-catalysed reaction for cross-link formation. The gradual increase in the fluorescence intensities attributed to dityrosine cross-links during the first 150 min at 0.8 u mL−1 GOx and 4.6 mM glucose (Fig. 2b, Nos. 1–3), compared with the intensities in the system using direct addition of 4.6 mM H2O2 (No. 8), supports this explanation.
These results demonstrate that the cross-link formation behaviour of the glucose-triggered co-enzymatic hydrogelation system is clearly different from that of the conventional HRP plus directly added H2O2 system. In addition, the results also demonstrate that gelation times and cross-link densities comparable to those obtained by the conventional system can be achieved in the co-enzymatic gelation system by controlling the HRP, GOx, and glucose concentrations.
Mechanical properties
The next stage of our investigation was evaluation of the mechanical properties of hydrogels obtained by indirect H2O2 addition. With respect to the effect of the standing period after adding glucose on hydrogelation (Fig. 4a), the significant increase (6-fold) in repulsion force toward compression on increasing the period of time from 1 h to 24 h can be explained by the increase in cross-link density between PH moieties. The increase in cross-link density after the first 1 h of standing is shown in Fig. 2b for No. 3. It is worth noting that a higher repulsion force toward compression was detected for the hydrogel obtained by indirect H2O2 addition using GOx (1 u mL−1 HRP, 0.83 u mL−1 GOx, and 4.6 mM glucose) than that obtained by direct H2O2 addition (1 u mL−1 HPR and 4.6 mM H2O2) at 24 h of standing after hydrogelation (Fig. 4a), despite the cross-link density being about one-half (Fig. 2b, Nos. 3 and 8). A possible explanation for this result is the formation of gels with more uniform microscopic structures in the indirect H2O2 addition system. In the current study, it is intuitive that a smaller amount of phenoxy radicals was produced during the first short period of time in the indirect H2O2 addition system than in the direct H2O2 addition system. This explanation is consistent with the results for the effects of glucose and GOx concentrations on the mechanical properties of the hydrogels (Fig. 4b, c): lower concentrations of glucose and GOx induced slower gelation (Fig. 3), and the resultant hydrogels showed larger repulsion forces toward compression. The influence of gelation rates on the physical properties, including the mechanical properties, of the resultant hydrogels has been reported for a variety of materials:24–26 Achilli and Mantovani26 reported that slower gelation of a collagen solution at pH 10, achieved by lowering the temperature, induced gels with higher resistance to compression. They suggested that this was the result of thicker fibril formation viaself-assembly of collagen molecules at lower temperatures. Viela et al.24 reported that faster gelation of a soy protein isolate and gellan gum mixture solution by direct addition of calcium ions to the solution resulted in gels with more heterogeneous microscopic structures as a result of the induction of thermodynamic incompatibility between the protein and the polysaccharide. In contrast, slower gelation by indirect addition of calcium ions allowed a longer time for greater organization of polymer molecules, inducing a more homogeneous microscopic structure and resulting in higher resistance to compression.
The results obtained in the mechanical property studies demonstrated the possibility of controlling the mechanical properties of hydrogels by modifying the gelation rate as well as the cross-link density. The number of operating parameters in the co-enzymatic indirect H2O2 addition system is greater than that in conventional direct H2O2 addition. Control of the gelation time and hydrogel properties is crucial for many biomedical applications, including tissue engineering, drug delivery, and wound dressings. The increase in the number of operating parameters demonstrates the feasibility of the current system using GOx for H2O2 supply because it gives enhanced control of the hydrogel characteristics. Studies of these applications are currently in progress and will be reported in the future.
Conclusions
The aim of this study was to investigate the feasibility of a HRP-catalysed hydrogelation system for aqueous solutions of polymers using H2O2 supplied by a GOx-catalysed reaction consuming glucose. Hydrogels were successfully obtained by this method, demonstrating the feasibility of control of cross-link formation behaviour between PH moieties and of gelation time of the aqueous solutions by altering the HRP, GOx, and glucose concentrations. Comparable cross-link densities and gelation times to those obtained using conventional HRP plus direct H2O2 addition could be obtained by altering the operating conditions. The shortest gelation time for a 1.1% (w/v) Alg-PH solution detected in this study was 17 s at 15 u mL−1 HRP, 8.3 u mL−1 GOx, and 4.6 mM glucose. In addition, it was demonstrated that it is possible to control the mechanical properties of the resultant hydrogels by altering the cross-linking formation behaviour, especially the gelation time. The Alg-PH hydrogel obtained at 1 u mL−1 HRP, 0.8 u mL−1 GOx, and 4.6 mM glucose showed a higher repulsion force toward compression than that obtained at 1 u mL−1 HRP and 4.6 mM H2O2 after 1 d of standing.
Considering the previous reports on the possibility of using hydrogels obtained by conventional direct H2O2 addition for a variety of biomedical applications such as drug delivery,3cell delivery,4 tissue repairing,9 and tissue engineering,6,10,11 we concluded that the glucose-triggered co-enzymatic hydrogelation of aqueous solutions of polymers bearing PH moieties has great potential for these biomedical applications.
References
- R. A. Gross, A. Kumar and B. Kalra, Chem. Rev., 2001, 101, 2097 CrossRef CAS.
- S. Kobayashi, H. Uyama and S. Kimura, Chem. Rev., 2001, 101, 3793 CrossRef CAS.
- F. Lee, J. E. Chung and M. Kurisawa, J. Controlled Release, 2009, 134, 186 CrossRef CAS.
- S. Sakai, I. Hashimoto, Y. Ogushi and K. Kawakami, Biomacromolecules, 2007, 8, 2622 CrossRef CAS.
- A. Ito, A. Mase, Y. Takizawa, M. Shinkai, H. Honda, K. Hata, M. Ueda and T. Kobayashi, J. Biosci. Bioeng., 2003, 95, 196 CAS.
- R. Jin, L. S. Teixeira, P. J. Dijkstra, C. A. van Blitterswijk, M. Karperien and J. Feijen, Biomaterials, 2010, 31, 3103 CrossRef CAS.
- A. S. Hoffman, Adv. Drug Delivery Rev., 2002, 54, 3 CrossRef CAS.
- M. Kurisawa, J. E. Chung, Y. Y. Yang, S. J. Gao and H. Uyama, Chem. Commun., 2005,(34), 4312 RSC.
- Y. S. Pek, M. Kurisawa, S. Gao, J. E. Chung and J. Y. Ying, Biomaterials, 2009, 30, 822 CrossRef.
- S. Sakai, Y. Ogushi and K. Kawakami, Acta Biomater., 2009, 5, 554 CrossRef CAS.
- S. Sakai, S. Ito, Y. Ogushi, I. Hashimoto, N. Hosoda, Y. Sawae and K. Kawakami, Biomaterials, 2009, 30, 5937 CrossRef CAS.
- F. Lee, J. E. Chung and M. Kurisawa, Soft Matter, 2008, 4, 880 RSC.
- R. Jin, C. Hiemstra, Z. Zhong and J. Feijen, Biomaterials, 2007, 28, 2791 CrossRef CAS.
- S. Sakai, T. Matsuyama, K. Hirose and K. Kawakami, Biomacromolecules, 2010, 11, 1370 Search PubMed.
- J. Hernandez-Ruiz, M. B. Arnao, A. N. P. Hiner, F. Garcia-Canovas and M. Acosta, Biochem. J., 2001, 354, 107 Search PubMed.
- K. J. Bayntona, J. K. Bewtrab, N. Biswasb and K. E. Taylor, Biochim. Biophys. Acta, 1994, 1206, 272 Search PubMed.
- S. Sakai, K. Hirose, K. Taguchi, Y. Ogushi and K. Kawakami, Biomaterials, 2009, 30, 3371 CrossRef CAS.
- Y. Ogushi, S. Sakai and K. Kawakami, Macromol. Biosci., 2009, 9, 262 Search PubMed.
- S. Sakai and K. Kawakami, Acta Biomater., 2007, 3, 495 CrossRef CAS.
- M. Meyer, G. Wohlfahrt, J. Knablein and D. Schomburg, J. Comput.-Aided Mol. Des., 1998, 12, 425 CrossRef CAS.
- C. Muzzarelli and R. A. A. Muzzarelli, Trends Glycosci. Glycotechnol., 2002, 14, 223 Search PubMed.
- M. Jimenez, F. Garcia Carmona, F. Garcia Canovas, J. L. Iborra, J. A. Lozano and F. Martinez, Arch. Biochem. Biophys., 1984, 235, 438 CrossRef CAS.
- K. Nazari, A. Mahmoudi, M. Shahrooz, R. Khodafarin and A. A. Moosavi Movahedi, J. Enzyme Inhib. Med. Chem., 2005, 20, 285 Search PubMed.
- J. A. P. Viela, Â. L. F. Cavallieri and R. L. Cunha, Food Hydrocolloids, 2011, 25, 1710 Search PubMed.
- C. K. Kuo and P. X. Ma, Biomaterials, 2001, 22, 511 CrossRef CAS.
- M. Achilli and D. Mantovani, Polymers, 2010, 2, 664 Search PubMed.
|
This journal is © The Royal Society of Chemistry 2012 |
Click here to see how this site uses Cookies. View our privacy policy here.