DOI:
10.1039/C2RA00667G
(Review Article)
RSC Adv., 2012,
2, 2200-2204
Potential applications of SPR in early diagnosis and progression of Alzheimer's disease
Received
3rd September 2011
, Accepted 19th December 2011
First published on 9th January 2012
Abstract
Alzheimer's disease (AD) is the most common chronic, progressive neurodegenerative disease, which affects an increasing number of elderly patients. So far, there is no cure for the disease. Early diagnosis to predict dementia outcome is of great importance. Biomarkers, as physiological indicators of AD, hold great promise for clinical diagnosis and drug discovery. Sensitive and selective methods that are amenable to the detection of biomarkers are much preferred. Surface plasmon resonance (SPR) is a sensitive optical technique capable of measuring analyte concentrations as well as kinetics of biomolecular interactions. It exhibits a variety of fascinating properties, such as high sensitivity, label-free and real-time analysis, and low sample consumption. In this review, we address the recent development on sensitive detection of a series of biomarkers for AD using SPR. Furthermore, amyloid-β (Aβ) fibril formation and interaction monitored by SPR have been documented. The challenges and perspectives inherent in the detection of the biomarkers are also highlighted.
1. Introduction
Alzheimer's disease (AD) is a severe neurodegenerative disorder characterized by the presence of senile plaques in brains of AD patients, loss of memory and cognitive decline.1–3 The major constituent of the senile plaques is amyloid-β (Aβ) peptide with 39–43 amino acid residues, which has been shown to play a central role in the pathogenesis of AD.3AD is a leading cause of dementia in elderly patients. However, no significant cognitive or functional impairment at the earliest stages is detectable by conventional clinical methods. For early diagnosis and treatment of AD, it is highly desirable to establish reliable methods for detection of biomarkers in body fluids (e.g., plasma, urine, and cerebrospinal fluid (CSF)), which can closely reflect the progression of the disease. Polyacrylamide gel electrophoresis, immunoprecipitation, mass spectrometry, fluorescent staining, and enzyme-linked immunosorbent assay (ELISA) have been employed to detect Aβ species from body fluids and cell media.4,5 Among them, ELISA has the best sensitivity, selectivity, and versatility.6–9 However, a typical sandwiched ELISA involves the use of a relatively expensive enzyme-linked antibody and carcinogenic substrate and requires 1–2 days.10 In comparison with these methods, surface plasmon resonance (SPR) holds great potential for early diagnosis of AD in that it is capable of measuring analyte concentrations as well as the kinetics and stoichiometry of biomolecular interactions. Its attractive features include high sensitivity, label-free and real-time measurement, a relatively simple procedure, and low sample consumption.11–13 The applications of SPR in biological interactions have covered a series of topics, such as antibody/antigen, DNA/DNA, DNA/protein, receptor/ligand, peptide/membrane, and protein/membrane binding.14–17 In this contribution, we focus on the recent development of SPR on sensitive determination of a series of biomarkers for AD and document the applications of SPR on Aβfibril formation and molecular interaction.
2. Principles of SPR
Many review articles and books concerning SPR fundamentals, chip design, analytical figures of merit, and signal amplification by nanoparticles or enzymes have been published.11,12,18–22 For a thorough understanding the potential of SPR for the detection of AD biomarkers and Aβ interactions, first we described briefly the basic principles of SPR.23SPR is a surface sensitive optical technique that can be divided into localized SPR and propagating SPR (usually denoted as SPR). The principles of localized SPR are different from those of propagating SPR. Typically, localized SPR occurs when surface plasmons are confined to metal nanostructures, which lead to highly localized electromagnetic fields.24,25 While in propagating SPR, the surface plasmons propagate along the planar metal-dielectric interface. As shown in Fig. 1, a typical SPR system consists of a light source, prism, sensor chip, flow channel, and a detector. P-polarized light emitted by the light source is reflected on the gold-coated sensor chip. At a specific angle of incidence, the total reflectance induces the SPR phenomenon. Due to the energy transfer from the incident light to free electrons on the metal surface, the SPR phenomenon leads to an attenuated reflected light. The intensity of the reflected light is measured against the incident light angle with the detector. The angle at which the reflectivity minimum is achieved is called an SPR resonance angle. This critical angle is sensitive to the events occurring at the metal film. The change in the refractive index on the sensor chip can be detected as a shift in the resonance angle. The shift in the resonance angle can be monitored over time to gain information on the affinity and kinetics of the molecular interactions.
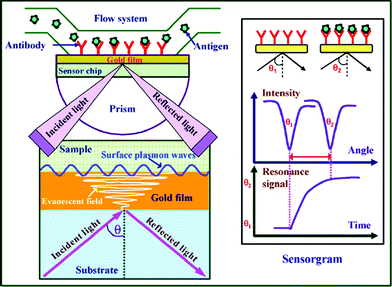 |
| Fig. 1 Schematic view of the surface plasmon resonance immunoassay technique. (From ref. 23, with permission. Copyright © 2007 Elsevier Science B. V.). | |
Although great efforts to screen biomarkers of AD have been made, to date only three biomarkers in CSF, including Aβ, total tau, and phosphorylated tau (Fig. 2), have the greatest diagnostic potential for AD.26Aβ40 (the 40-residue form) and Aβ42 (the 42-residue variant) are two predominant proteolytic cleavage products from amyloid precursor protein (APP) by β- and γ-secretase (Fig. 2) and have been considered as promising biomarkers for AD in spite of their controversial metabolisms.27Aβ42 exhibits greater tendency to form amyloid fibrils than the much more abundant Aβ40.3,28
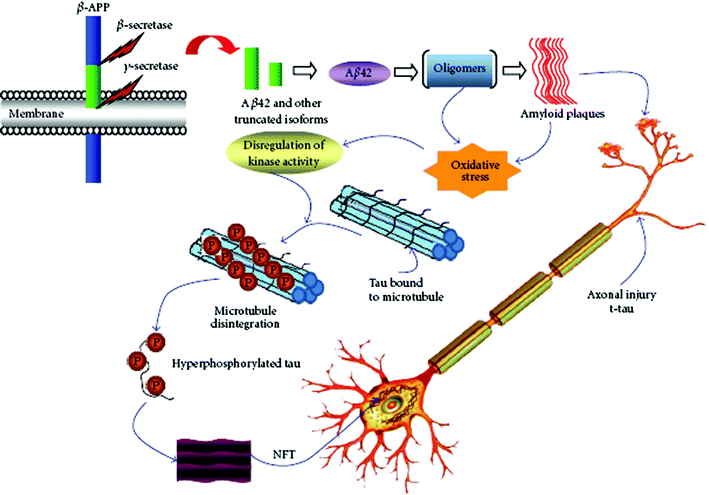 |
| Fig. 2 Pathological cascades and potential biomarkers of AD. (From ref. 26, http://www.hindawi.com/journals/ijad/2010/606802/). | |
Lee et al. used a gold nanoparticle–antibody complex for amplified SPR detection of synthetic Aβ40 peptide in buffer solution.29 In that work, unmodified gold films were used for immobilization of the capture antibody. The extent of nonspecific adsorption and selectivity of the method were not examined. Many studies have suggested that the content of Aβ42 in CSF samples from AD patients has significantly decreased due to the deposition of Aβ42 in senile plaques, with lower levels diffusing into CSF.7,30–32 However, the use of Aβ42 alone cannot accurately diagnose AD due to the similarities between AD and other neurological diseases. It has therefore been suggested that the concentration ratio between Aβ40 and Aβ42 can be more pronounced for AD diagnosis.31,33,34 Recently, we used a dual channel SPR to determine the concentration ratio of Aβ40versusAβ42 in CSF samples by immobilizing antibodies that specifically recognize the respective peptides onto the two fluidic channels (Fig. 3).35Via signal amplification by a conjugate formed between streptavidin and a biotinylated monoclonal antibody that is selective to the N-terminus of both Aβ40 and Aβ42 peptides, concentrations as low as 20 pM of the Aβpeptides can be readily determined. The range of concentration measurable by this method spans almost 4 orders of magnitude. The concentration ratio between Aβ40 and Aβ42 in CSF samples from AD patients has been found to be almost twice as high as that from healthy persons. The proposed method is advantageous over ELISA in that it obviates the need of an enzyme for signal amplification and a carcinogenic substrate for chemiluminescent detection. Using the sensing protocol, multiple samples can be assayed on a single chip with high reproducibility. Thus, SPR could be potentially utilized as an effective means for facile and sensitive clinical analyses of important biomarkers of neurodegenerative diseases.
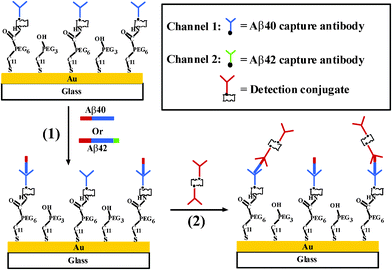 |
| Fig. 3 Schematic diagram showing the simultaneous SPR detection of Aβ40 and Aβ42. (From ref. 35, with permission. Copyright © 2010 American Chemical Society). | |
The most recently discovered biomarkers for AD are total tau and phosphorylated tau proteins, which constitute the major components of neurofibrillary tangles.36–39 The increased levels of total tau and phosphorylated tau proteins have been documented in AD patients using ELISA.39,40 Because total tau is also present in other dementias with high concentrations, the specificity of total tau for AD diagnosis is not that high.37,39,40 With the combined technique of SPR with atomic force spectroscopy, tau–tau interaction has been investigated.41 However, to date no work about SPR detection of total tau and phosphorylated tau proteins has been reported.
The proposed molecular and biochemical markers exhibit certain diagnostic specificity for AD. On the other hand, the characterization of Aβfibril formation and interaction may unravel the pathogenesis of AD, aiding the early diagnosis of the disease.
4. Study of Aβfibril formation and interaction with SPR
The aggregation process of Aβ has been considered as the possible cause of AD. The study of Aβfibril formation and interaction has a potential implication to the interpretation about the mechanism of AD pathogenesis. Techniques to study Aβfibril formation include circular dichroism, fluorescence spectroscopy, electron microscopy and dynamic light scattering. Recently, SPR has been utilized to investigate the mechanism inherent in Aβfibril formation and the effect of various inhibitors on the aggregation process. Tjernberg et al., for the first time, employed SPR to measure the specific interaction between Aβ and peptide fragments that are responsible for the fibril formation.42 The binding process of small peptides with Aβ may form the basis for evaluating potential inhibitors of Aβ aggregation.43 Hasegawa et al. and Cannon et al. used SPR to examine the kinetic models of Aβfibril extension and dissociation.44,45 Using in situSPR and ex situatomic force microscopy, the aggregation of Aβ42 from fresh monomers to fully grown fibrils was examined.46 Compared with conventional methods, SPR is label-free and capable of measuring the length of both the initial seed fibrils and growing fibrils, determining affinity or dissociation constants of the interaction, and screening drugs that can inhibit Aβ aggregation in real time. Recent studies suggest that Aβ aggregation and toxicity are facilitated by metal ions.47,48 Hu et al. illustrated the kinetics of Aβ aggregation induced by metal ions using a SPR sensor.49 The results indicate that metal ions, such as Zn(II), Cu(II), Ca(II), Fe(II), and Fe(III), promoted Aβ aggregation with various propensities and the promotion process could be effectively disrupted by a metal-ion chelator. These proof-of-concept experiments demonstrate that SPR can potentially serve as a viable alternative to investigate the mechanism inherent in AD pathogenesis.
A large number of studies have shown that Aβ aggregation and polymerization could be inhibited by various Aβ-binding proteins, such as transthyretin,50 cystatin C,51lipocalin-type prostaglandin D synthase (L-PGDS)/β-trace,5217β-hydroxysteroid dehydrogenase type 10 (17β-HSD10) enzyme53,54 and apolipoprotein E (apoE).55 These proteins are abundant in CSF and play an important role in sequestering Aβ and preventing amyloid formation. Therefore, Aβ-binding proteins also serve as potential AD biomarkers. As a powerful technique, SPR has been utilized to determine the Aβ-binding proteins in an effort to gain insight into the role of these proteins in the pathology of AD.52–59 Bohrmann et al. studied the interaction of a range of plasma proteins with Aβ to explore the possible mechanism by which these proteins may influence Aβpolymerization.58,59 Kanekiyo et al. examined the strong binding (KD = 18∼50 nM) of Aβ monomers and fibrils with L-PGDS/β-trace, the most abundant protein in CSF.52 The binding site is located within residues 25–28 in Aβ. ApoE in CSF is closely related to AD development. One of the earliest studies is to examine the interaction of apoE3, apoA-I and apoA-II with Aβ using SPR.57 The KD values of the interaction were determined to be in the nanomolar range. In another work, the effect of heparin and sialic acid moieties of apoE on the binding between apoE and Aβ40 or Aβ42 has been demonstrated using SPR.55,60 The above results demonstrated the potential of SPR for studying the interactions between Aβ and Aβ-binding proteins and for screening potential agents that modulate these interactions. On the other hand, specific binding between Aβ and Aβ-binding proteins has afforded a new strategy for the detection of these proteins in AD. For example, Homola and co-workers employed SPR to determine 17β-HSD10 enzyme and a peptide analog in artificial CSF samples.53 A multi-channel SPR sensor functionalized with Aβ40 has been constructed and 17β-HSD10 enzyme could be measured with a detection limit of 5 ng ml−1 in artificial CSF samples (Fig. 4).
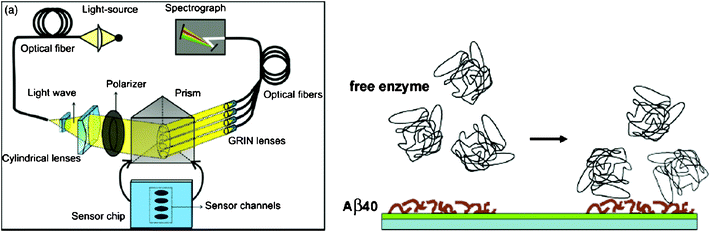 |
| Fig. 4 (Left) Scheme of the four-channel SPR sensor. Polychromatic light beam is directed through prism at a fixed angle and excites surface plasmon waves at the metal–dielectric interface. (Right) Scheme of detection of free 17β-HSD10 enzyme on the Aβ40-immobilized surface. (From ref. 53, with permission. Copyright © 2009 Elsevier Science B. V.). | |
Recently, the production of antibody-based vaccines for the treatment of AD has been focused.61 Again, SPR has been successfully used to characterize the binding specificity between the antibody and Aβ or APP that was immobilized onto the sensor chip. The binding specificity between monoclonal or polyclonal antibodies and the immobilized Aβ40, Aβ42 or APP may aid the unravelling of the intraneural staining profile of Aβpeptides in the transgenic mouse model that expresses a mutant APP and presenilin.62 Ramakrishnan et al. determined the binding kinetics of monoclonal antibodies with the monomeric or fibrillar Aβ40 species using SPR.56 The difference in the kinetic binding between monomeric and fibrillar forms could be utilized to distinguish monoclonal antibodies that might be useful for immunotherapy. Thus, SPR has been proved to be a valuable tool for the development of immunisation strategies for the treatment of AD because SPR can provide information on binding kinetics in real time, being advantageous over other techniques.
5. Conclusions and outlook
Modern, highly sensitive and selective analytical methods have become extremely important in clinical diagnosis, drug discovery and disease treatment. From the examples described above, it is evident that SPR can serve as a viable alternative for early diagnosis and progression of AD owning to its fascinating properties. It is capable of measuring biomarker concentrations in its native state at remarkably low detection levels (pM or even fM) as well as kinetics of biomolecular interactions in real time. To further enhance the sensitivity of SPR, highly aligned nanoarrays or nanopatterns for biological applications have also been developed. However, due to the clinical complexity, assay of only one biomarker might not be able to discriminate between AD and other types of dementia. The use of multi-channel SPR is expected to improve the sample throughput, enabling the accurate determination of several biomarkers on a single chip. The multiplexed and parallel monitoring of a large number of biomarkers with SPR imaging is particularly appealing for biomarkers profiling in body fluids. Furthermore, the combination of SPR with other techniques, such as fluorescence spectroscopy,13mass spectrometry,63scanning electrochemical microscopy,64circular dichroism spectroscopy65 and Fourier transform infrared spectroscopy66 could improve the diagnostic specificity and provide more detailed insight into the molecular mechanism of AD and other neurodegenerative diseases.
Acknowledgements
Partial support of this work by the National Natural Science Foundation of China (Nos. 21175156, 20975114), Program for New Century Excellent Talents in University (NCET-10-0796), Specialized Research Fund for the Doctoral Program of Higher Education (No. 20100162110018), and the Graduate Degree Thesis Innovation Foundation of Hunan Province (CX2011B082) is gratefully acknowledged.
References
- J. Hardy and D. J. Selkoe, Science, 2002, 297, 353 CrossRef CAS
.
- C. L. Masters, G. Simms, N. A. Weinman, G. Multhaup, B. L. McDonald and K. Beyreuther, Proc. Natl. Acad. Sci. U. S. A., 1985, 82, 4245 CrossRef CAS
.
-
G. Thinakaran, E. H. Koo, Alzheimer's Disease, Springer, New York, 2007 Search PubMed
.
- T. E. Golde, C. B. Eckman and S. G. Younkin, Biochim. Biophys. Acta, 2000, 1502, 172 CAS
.
- L. A. Munishkina and A. L. Fink, Biochim. Biophys. Acta, 2007, 1768, 1862 CAS
.
- S. A. Gravina, L. B. Ho, C. B. Eckman, K. E. Long, L. Otvos, L. H. Younkin, N. Suzuki and S. G. Younkin, J. Biol. Chem., 1995, 270, 7013 CrossRef CAS
.
- P. D. Mehta, T. Pirttila, B. A. Patrick, M. Barshatzky and S. P. Mehta, Neurosci. Lett., 2001, 304, 102 CrossRef CAS
.
- P. Seubert, C. Vigopelfrey, F. Esch, M. Lee, H. Dovey, D. Davis, S. Sinha, M. Schlossmacher, J. Whaley, C. Swindlehurst, R. McCormack, R. Wolfert, D. Selkoe, I. Lieberburg and D. Schenk, Nature, 1992, 359, 325 CrossRef CAS
.
- N. Suzuki, T. T. Cheung, X. D. Cai, A. Odaka, L. Otvos, C. Eckman, T. E. Golde and S. G. Younkin, Science, 1994, 264, 1336 CAS
.
-
D. L. Nelson and M. M. Cox, Lehninger Principles of Biochemistry, W. H. Freeman, New York, 2004 Search PubMed
.
-
J. Homola, Surface Plasmon Resonance Based Sensors, Springer, Berlin, 2006 Search PubMed
.
- J. Homola, Chem. Rev., 2008, 108, 462 CrossRef CAS
.
- K. S. Phillips and Q. Cheng, Anal. Bioanal. Chem., 2007, 387, 1831 CrossRef CAS
.
- M. I. Aguilar and D. H. Small, Neurotoxic. Res., 2005, 7, 17 CrossRef CAS
.
- L. He, M. D. Musick, S. R. Nicewarner, F. G. Salinas, S. J. Benkovic, M. J. Natan and C. D. Keating, J. Am. Chem. Soc., 2000, 122, 9071 CrossRef CAS
.
- L. A. Lyon, M. D. Musick and M. J. Natan, Anal. Chem., 1998, 70, 5177 CrossRef CAS
.
- X. Yu, D. Xu and Q. Cheng, Proteomics, 2006, 6, 5493 CrossRef CAS
.
- A. Abbas, M. J. Linman and Q. Cheng, Biosens. Bioelectron., 2011, 26, 1815 CrossRef CAS
.
- D. Samanta and A. Sarkar, Chem. Soc. Rev., 2011, 40, 2567 RSC
.
- P. K. Jain, X. Huang, I. H. El-Sayed and M. A. El-Sayed, Plasmonics, 2007, 2, 107 CrossRef CAS
.
- J. Homola, Anal. Bioanal. Chem., 2003, 377, 528 CrossRef CAS
.
- J. Homola, S. S. Yee and G. Gauglitz, Sens. Actuators, B, 1999, 54, 3 CrossRef
.
- D. R. Shankaran, K. V. Gobi and N. Miura, Sens. Actuators, B, 2007, 121, 158 CrossRef
.
- J. C. Riboh, A. J. Haes, A. D. McFarland, C. R. Yonzon and R. P. Van Duyne, J. Phys. Chem. B, 2003, 107, 1772 CrossRef CAS
.
- C. R. Yonzon, E. Jeoung, S. Zou, G. C. Schatz, M. Mrksich and R. P. Van Duyne, J. Am. Chem. Soc., 2004, 126, 12669 CrossRef CAS
.
- A. Anoop, P. K. Singh, R. S. Jacob and S. K. Maji, Int. J. Alzheimers Dis., 2010, 2010, 606802 Search PubMed
.
- D. J. Selkoe and J. Hardy, Science, 2002, 298, 963 CrossRef CAS
.
- C. J. Barrow and M. G. Zagorski, Science, 1991, 253, 179 CAS
.
- J. H. Lee, D. Y. Kang, T. Lee, S. U. Kim, B. K. Oh and J. W. Choi, J. Nanosci. Nanotechnol., 2009, 9, 7155 CAS
.
- P. D. Mehta, T. Pirttila, S. P. Mehta, E. A. Sersen, P. S. Aisen and H. M. Wisniewski, Arch. Neurol., 2000, 57, 100 CrossRef CAS
.
- M. Shoji, E. Matsubara, M. Kanai, M. Watanabe, T. Nakamura, Y. Tomidokoro, M. Shizuka, K. Wakabayashi, Y. Igeta, Y. Ikeda, K. Mizushima, M. Amari, K. Ishiguro, T. Kawarabayashi, Y. Harigaya, K. Okamoto and S. Hirai, J. Neurol. Sci., 1998, 158, 134 CrossRef CAS
.
- A. Tamaoka, N. Sawamura, T. Fukushima, S. Shoji, E. Matsubara, M. Shoji, S. Hirai, Y. Furiya, R. Endoh and H. Mori, J. Neurol. Sci., 1997, 148, 41 CrossRef CAS
.
- R. Fukuyama, T. Mizuno, S. Mori, K. Nakajima, S. Fushiki and K. Yanagisawa, Eur. Neurol., 2000, 43, 155 CrossRef CAS
.
- O. Hansson, H. Zetterberg, P. Buchhave, U. Andreasson, E. Londos, L. Minthon and K. Blennow, Dementia Geriatr. Cognit. Disord., 2007, 23, 316 CrossRef CAS
.
- N. Xia, L. Liu, M. G. Harrington, J. Wang and F. Zhou, Anal. Chem., 2010, 82, 10151 CrossRef CAS
.
- K. Blennow and H. Hampel, Lancet Neurol., 2003, 2, 605 CrossRef CAS
.
- K. Ishiguro, H. Ohno, H. Arai, H. Yamaguchi, K. Urakami, J. M. Park, K. Sato, H. Kohno and K. Imahori, Neurosci. Lett., 1999, 270, 91 CrossRef CAS
.
- M. Vandermeeren, M. Mercken, E. Vanmechelen, J. Six, A. Vandevoorde, J. J. Martin and P. Cras, J. Neurochem., 1993, 61, 1828 CrossRef CAS
.
- E. Vanmechelen, H. Vanderstichele, P. Davidsson, E. Van Kerschaver, B. Van Der Perre, M. Sjogren, N. Andreasen and K. Blennow, Neurosci. Lett., 2000, 285, 49 CrossRef CAS
.
- K. Blennow, A. Wallin, H. Agren, C. Spenger, J. Siegfried and E. Vanmechelen, Mol. Chem. Neuropathol., 1995, 26, 231 CrossRef CAS
.
- A. Barrantes, J. Sotres, M. Hernando-Pérez, M. J. Benítez, P. J. de Pablo, A. M. Baró, J. Avila and J. S. Jiménez, J. Alzheimers Dis., 2009, 18, 141 CAS
.
- L. O. Tjernberg, J. Naslund, F. Lindqvist, J. Johansson, A. R. Karlstrom, J. Thyberg, L. Terenius and C. Nordstedt, J. Biol. Chem., 1996, 271, 8545 CrossRef CAS
.
- C. W. Cairo, A. Strzelec, R. M. Murphy and L. L. Kiessling, Biochemistry, 2002, 41, 8620 CrossRef CAS
.
- K. Hasegawa, K. Ono, M. Yamada and H. Naiki, Biochemistry, 2002, 41, 13489 CrossRef CAS
.
- M. J. Cannon, A. D. Williams, R. Wetzel and D. G. Myszka, Anal. Biochem., 2004, 328, 67 CrossRef CAS
.
- J. Ryu, H.-A. Joung, M.-G. Kim and C. B. Park, Anal. Chem., 2008, 80, 2400 CrossRef CAS
.
- H. Kozlowski, A. Janicka-Klosb, J. Brasunb, E. Gaggelli, D. Valensinc and G. Valensinc, Coord. Chem. Rev., 2009, 253, 2665 CrossRef CAS
.
- P. Faller, ChemBioChem, 2009, 10, 2837 CrossRef CAS
.
- W.-P. Hu, G.-L. Chang, S.-J. Chen and Y.-M. Kuo, J. Neurosci. Methods, 2006, 154, 190 CrossRef CAS
.
- L. Liu and R. M. Murphy, Biochemistry, 2006, 45, 15702 CrossRef CAS
.
- S. A. Kaeser, M. C. Herzig, J. Coomaraswamy, E. Kilger, M. L. Selenica, D. T. Winkler, M. Staufenbiel, E. Levy, A. Grubb and M. Jucker, Nat. Genet., 2007, 39, 1437 CrossRef CAS
.
- T. Kanekiyo, T. Ban, K. Aritake, Z. L. Huang, W. M. Qu, I. Okazaki, I. Mohri, S. Murayama, K. Ozono, M. Taniike, Y. Goto and Y. Urade, Proc. Natl. Acad. Sci. U. S. A., 2007, 104, 6412 CrossRef CAS
.
- K. Hegnerová, M. Bocková, H. Vaisocherová, Z. Krištofiková, J. Říčný, D. Řípová and J. Homola, Sens. Actuators, B, 2009, 139, 69 CrossRef
.
- Z. Krištofiková, M. Bocková, K. Hegnerová, A. Bartoš, J. Klaschka, J. Říčný, D. Řípová and J. Homola, Mol. BioSyst., 2009, 5, 1174 RSC
.
- M. Sugano, K. Yamauchi, K. Kawasaki, M. Tozuka, K. Fujita, N. Okumura and H. Ota, Clin. Chim. Acta, 2008, 388, 123 CrossRef CAS
.
- M. Ramakrishnan, K. K. Kandimalla, T. M. Wengenack, K. G. Howell and J. F. Poduslo, Biochemistry, 2009, 48, 10405 CrossRef CAS
.
- V. V. Shuvaev and G. Siest, FEBS Lett., 1996, 383, 9 CrossRef CAS
.
- B. Bohrmann, M. Adrian, J. Dubochet, P. Kuner, F. Muller, W. Huber, C. Nordstedt and H. Dobeli, J. Struct. Biol., 2000, 130, 232 CrossRef CAS
.
- B. Bohrmann, L. Tjernberg, P. Kuner, S. Poli, B. Levet-Trafit, J. Naslund, G. Richards, W. Huber, H. Dobeli and C. Nordstedt, J. Biol. Chem., 1999, 274, 15990 CrossRef CAS
.
- V. V. Shuvaev and G. Siest, Neurosci. Lett., 2000, 280, 131 CrossRef CAS
.
- A. Monsonego and H. L. Weiner, Science, 2003, 302, 834 CrossRef CAS
.
- O. Wirths, G. Multhaup, C. Czech, N. Feldmann, V. Blanchard, G. Tremp, K. Beyreuther, L. Pradier and T. A. Bayer, Brain Pathol., 2002, 12, 275 CrossRef CAS
.
- J. Borch and P. Roepstorff, Mol. Biotechnol., 2006, 33, 179 CrossRef CAS
.
- J. Xiang, J. Guo and F. Zhou, Anal. Chem., 2006, 78, 1418 CrossRef CAS
.
- I. Lieberman, G. Shemer, T. Fried, E. M. Kosower and G. Markovich, Angew. Chem., Int. Ed., 2008, 47, 1 CrossRef
.
- V. Yashunsky, V. Lirtsman, M. Golosovsky, D. Davidov and B. Aroeti, Biophys. J., 2010, 99, 4028 CrossRef CAS
.
|
This journal is © The Royal Society of Chemistry 2012 |
Click here to see how this site uses Cookies. View our privacy policy here.