DOI:
10.1039/C2RA00704E
(Review Article)
RSC Adv., 2012,
2, 2184-2190
Scaffold-free tissue engineering using cell sheet technology
Received
9th September 2011
, Accepted 18th November 2011
First published on 4th January 2012
Abstract
Cell sheet technology is a tissue engineering methodology requiring no scaffolds. Confluent cultured cells can be harvested as an intact cell sheet using a temperature-responsive polymer, poly(N-isoproplyacrylamide) (PIPAAm), grafted cell culture surface, offering noninvasive control of cell attachment and detachment only by changing the temperature across 32 °C, without any protease treatments. Avoiding protease treatment preserves complete cell–cell junctions, cell surface proteins, and the extracellular matrix in the cell sheet. Therefore, functional three-dimensional (3D) tissue can be easily fabricated by layering cell sheets without the use of scaffolds. Cell sheet technology has been applied in regenerative medicine for several tissues, and a number of clinical trials have already started. In addition, to fabricate more complex and functional 3D tissue, cell micro-patterning technology can be combined with cell sheet technology, and this interdisciplinary technology could produce interesting results. In this review, recent advances in temperature-responsive culture surfaces, cell sheet and cell micro-patterning technologies are summarized and discussed. In addition, the application of these technologies to regenerative medicine and the re-construction of various tissues including heterogeneous tissues are also discussed.
1. Introduction
Cell-based regenerative therapy has been progressing rapidly and a number of clinical trials have already started.1–10 Although cell therapy using the direct injection of isolated cells shows enormous potential, several problems still remain to be solved, the most severe of which is the significant loss and death of injected cells after transplantation.11 The poor survival of implanted cells reduces the expected therapeutic effects; to overcome these problems, tissue engineering has been used as the second generation of cell therapy.12,13 Tissue engineering is based on the concept that pre-made porous three dimensional (3D) scaffolds (for instance, collagen gel, fibrin gel, and aminotic membranes) can be used as an alternative to an extracellular matrix (ECM), and living cells are seeded into the scaffolds.14 These bioengineered tissues can be transplanted into patients to restore or improve their original tissue function, and clinical applications in regenerative medicine using various bioengineered tissues have already been used successfully.13,15–17
In contrast to scaffold-based tissue engineering, our laboratory originally developed tissue engineering without any scaffolds, called “cell sheet technology”. By using a culture surface grafted with a temperature-responsive polymer, poly(N-isoproplyacrylamide) (PIPAAm), cell attachment and detachment can be controlled by simple temperature changes.18,19 Clinical trials using cell sheets have already started in some tissues, and we are now planning to expand the clinical application to other tissues. There are also several new cell micro-patterning methods and temperature-responsive cell micro-patterning culture surfaces that have been recently developed.20–28 The current challenge is to fabricate more functionally, complex, and thicker heterogeneous tissues using various interdisciplinary technologies.
This review is a detailed summary of temperature-responsive culture surfaces, cell sheet technology, and its applications to regenerative medicine; in addition to several cell micro-patterning methods.
2.
Cell attachment/detachment controlled by a temperature-responsive culture surface
PIPAAm is known to exhibit temperature-responsive reversible hydrophobicity/hydrophilicity changes in aqueous solutions.29 Our laboratory succeeded in the fabrication of a PIPAAm-grafted cell culture dish, called a “temperature-responsive culture dish”.18,19 After applying a layer of N-isoproplyacrylamide (IPAAm) monomer, the surface of a normal cell culture dish is subjected to electron beam (EB) irradiation, leading to the covalent immobilization of the polymer onto the surface. An important characteristic of the PIPAAm-grafted layer is that it is reversible-temperature-responsive. At temperatures below 32 °C, which is the lower critical solution temperature (LCST) of PIPAAm molecules, the surface of the PIPAAm-grafted dish becomes hydrophilic, and above 32 °C, the surface becomes hydrophobic. Because cultured cells more readily attach to hydrophobic surfaces than hydrophilic surfaces, the cultured cells will attach and proliferate to confluent on the culture dish at 37 °C. Then the confluent cells can be detached as a biologically active and functional monolithic cell sheet by lowering the culture temperature to 20 °C (Fig. 1a–c).18,19,30–32 Temperature-dependent cell attachment/detachment has only been observed on a 20 nm thick PIPAAm-grafted surface, and cells rarely attach to grafted layers that are thicker than 30 nm.33 Thus, the thickness of the PIPAAm-grafted layers on the dish plays a crucial role in the temperature-dependent hydrophilic/hydrophobic changes and cell attachment/detachment. The thickness of grafted polymers can be controlled by the IPAAm monomer concentration and radiation energy. Since cell sheets can be harvested by simply changing the temperature without using protease treatments, the cell–cell junctions (which are susceptible to protease treatment) are preserved intact (Fig. 1c).30–32 In addition to the cell–cell junctions, ECM components mediating cell adhesion are also conserved by using this method.34 Because cell sheets containing ECM can be attached to other cell sheets, 3D tissues can be easily fabricated by simply layering cell sheets without any scaffolds, resulting in cell-dense tissues (Fig. 1d, e).30–32 An important advantage to cell sheets is that they can be transplanted directly onto host tissues without sutures. Cell sheets prepared on temperature-responsive culture dishes can be applied to the re-construction of various tissues in regenerative medicine; the details of which are discussed in the following chapter.
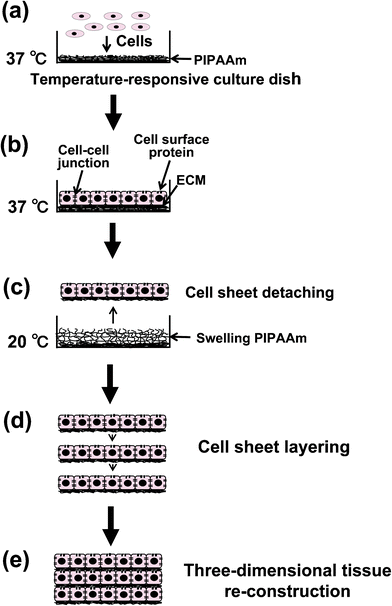 |
| Fig. 1 Schematic illustrations of the fabrication of a cell-dense three-dimensional tissue by cell sheet layering. By lowering the temperature; cultured cells on a poly(N-isoproplyacrylamide) (PIPAAm)-grafted temperature-responsive culture dish are harvested as a contiguous cell sheet, preserving cell–cell junctions, cell surface proteins, and the extracellular matrix (ECM). | |
3. The applications of cell sheet technology for regenerative medicine
3.1. Corneal epithelial
Limbal stem-cell deficiency from severe trauma (thermal or chemical burns) or disease (Stevens–Johnson syndrome or ocular pemphigoid) causes corneal opacification and vision loss. Under normal circumstances, the conventional course of treatment is corneal transplantation using donated eyes; however, there is a high risk of graft rejection and a shortage of donor eyes. A novel therapy using autologous epithelial cell sheets was developed to improve the visual acuity of patients with either unilateral or bilateral diseases by surrogating the damaged tissues with implanted cell sheets.35,36 In patients with unilateral disease, autologous epithelial cells can be isolated from the healthy contralateral eye by a simple biopsy. In patients with complete bilateral corneal epithelial stem cell deficiencies, an alternative autologous therapy using the patient's own oral mucosa as the source of epithelial stem cells was established.36 Using a temperature-responsive culture dish, these autologous epithelial cells can be recovered as a monolithic epithelial cell sheet having both cell–cell junctions and deposited ECM on the basal surface.35,36 The presence of ECM allows the cell sheet to attach rapidly and stabilize onto the host eye without any sutures.35,36 The treated corneal surfaces were completely protected from fluorescein penetration immediately after the transplantation by the implanted cell sheet because they contained intact cell–cell junctions.35,37 The implanted autologous oral mucosal epithelial cell sheet showed (1) a similar histological structure to corneal epithelium and (2) an adequate modulation in keratin expression profiles towards a corneal phenotype.37 Clinical results have shown good results for the long-term maintenance of healthy ocular surfaces with significant recoveries in all patients who had lost vision.36
3.2. Esophageal ulceration
The recent significant progress of endoscopic mucosal resection and endoscopic submucosal dissection (ESD) techniques now enable the effective removal of early cancers of the gastrointestinal tract without the need for a conventional open surgery.38–40 On the other hand, the resection of cancerous epithelial and mucosal tissues removes the normal epithelial barrier function in the operated areas. The loss of the epithelial barrier induces (1) postoperative inflammation and (2) stenosis that can negatively affect the patient's quality of life.41,42 To mitigate postoperative esophageal ulceration, an innovative method combining ESD with endoscopic transplantation of autologous oral mucosal epithelial cell sheets was developed.43 In a large animal model, autologous oral mucosal epithelial cell sheets were attached directly to the esophageal ulcer using endoscopic forceps without the need for sutures or additional adhesive agents and the cell sheets successfully substituted for the lost epithelial barrier.43 The implanted cells survived on the underlying muscle layers in the ulcer sites, providing an intact and stratified epithelium.43 The transplantation of autologous cell sheets significantly accelerated post-ESD wound healing and inhibited stenosis.43 A clinical trial for mitigating postoperative esophageal ulceration using autologous cell sheets has already started.
3.3. Cardiac tissue
Cardiac disease is still a major cause of morbidity and mortality especially in developed countries, even though there are various therapies available.44 Understandably, cell-based therapy and tissue engineering are believed to provide hope for improving cardiac disease. Pulsatile cardiac cells, cardiomyocytes, which assist the heart pump function, are difficult to propagate in vivo or in vitro, so an alternative cell source is necessary to apply this therapy. Possible cell types, include skeletal myoblasts, bone marrow-derived cells, and endothelial progenitor cells, which are being investigated as possible clinical cell sources and clinical trials using these cells have already been started.45–47 Moreover, cell sheet technology has already been applied for improving damaged heart functions.48 In this study, autologous skeletal myoblast sheets, adipose-derived and menstrual blood-derived mesenchymal stem cell sheets, and cardiac progenitor cell sheets were used.48–56 The transplantation of these cell sheets was confirmed to improve damaged heart function in several animal models, mainly by the paracrine effects of some factors, including cytokines/chemokines produced by the implanted cell sheets.48–56 The therapeutic effects from transplantation of cell sheets were found to be more effective and reliable than the injection of dissociated cells, because of the higher higher efficiency of the transplantation of cell sheets without cell loss. These encouraging results are the basis for a clinical trial using autologous cell sheets that is currently underway.
3.4. Periodontal tissues
Periodontitis is an inflammation of the gums and gingival pocket areas, causing the subsequent destruction of periodontal tissues, especially the periodontal ligament (PDL) and alveolar bone loss, eventually leading to tooth loss. In addition, periodontitis is also associated with several other diseases including cardiovascular disease, strokes, and diabetes.57Cell sheet technology was applied to the regeneration of damaged periodontal tissue.58–61PDL cells can be harvested from a temperature-responsive culture dish as a contiguous cell sheet with deposited ECM, and these PDL cell sheets have been successfully transplanted onto dental root surfaces in several animal experiments.58–61 The transplantation of a single PDL cell sheet leads to the formation of new immature fibers which are obliquely anchored to the dentin surface in a similar way to native PDL fibers. Furthermore, the transplantation of a triple-layered PDL cell sheet induces the regeneration of complete periodontal tissue, including alveolar bone, cementum, and well-oriented collagen fibres.61 These results show that the PDL cell sheets have a great potential for regeneration or replacement of lost periodontal tissue, and preventing tooth loss. Pre-clinical experiments, including the safety assessment and efficacy of human PDL cell sheets produced in a cell processing center, showed that in vitro human PDL cell sheets had a high alkaline phosphatase activity and periostin expression, which are known PDL markers, without any microorganism contamination. PDL cell sheets induced the formation of cementum and PDL-like tissue in immunodeficient mice and presented no evidence of malignant transformation.62 A clinical trial for periodontal tissue regeneration using cell sheet technology is starting shortly.
3.5. Sealing of lung air leak
Postoperative air leaks from visceral pleural injury during lung resection are an unavoidable complication and can cause thoracic emphysema leading to extended chest tube durations, prolonging hospitalization and reducing the quality of life of patients.63–65 Although there have been improvements in surgical techniques and development of various biological sealants, each of these have their own problems and no perfect therapy is available. A novel lung air leak sealant was recently developed using cell sheet technology.66,67 An autologous dermal fibroblast sheet prepared on a temperature-responsive culture dish, was evaluated in visceral pleural injury animal models (rat, rabbit, and porcine).66,67 The autologous dermal fibroblast sheets were easy to handle and apply during surgical procedures.66,67 In contrast to conventional biological sealants, dermal fibroblast sheets can seal lung air leaks immediately and permanently without suture.66,67 A water seal test revealed the stable closure of the air leaks against an airway pressure of 25 cm H2O.67 Dermal fibroblast sheets were sufficiently responsive to lung respiration movement and no recurrence of the air leak was observed during a four-week follow-up period after transplantation.67 This confirmed that implanted fibroblast sheets can play an important role as a sealant for lung air leaks. Experiments using large animal models have already been completed, and a clinical trial for treating lung air leak using cell sheets is currently being prepared.
3.6. Pancreatic islet tissue
Diabetes mellitus commonly produces a high blood glucose level that can cause various symptoms (ketoacidosis, cardiac disease, stroke, renal failure, and vision loss).68 Several therapies have been attempted, and there are some new therapies under development.68,69 One new development is using cell therapy as a promising approach for treating diabetes mellitus; our laboratory has established a novel tissue engineered approach using cell sheet technology.70 A rat pancreatic islet cell sheet can be harvested on a temperature-responsive culture dish pre-coated with laminin-5.70 The cell sheet retained the functional activity of native islet cells (the production of insulin and glucagon, and glucose-dependent insulin secretion).70 Thus, the transplantation of pancreatic islet cell sheets is expected to be a surrogate for the damaged host islet. The transplantation of pancreatic islet cell sheets into the subcutaneous space of rats produced an islet structure which retained the biological functions of producing and secreting insulin.70 This successful result demonstrates that cell sheet technology can be a good foundation for creating a novel tissue engineering-based cell therapy for diabetes mellitus.
4. Fabrication trial of complicated heterogeneous tissues
Mimicking the cell environment in tissue is important for analyzing isolated cell functions and re-constructing various tissues. Almost all tissues and organs are composed of heterogeneous cells, and direct interactions and communication among those cells are critical to the proper function and survival of tissues and organs. Therefore, the establishment of a heterogeneous cell culture system is important for fabricating or re-constructing native tissue/organ-like 3D constructs. To mimic a heterogeneous cell environment in vitro, cell micro-patterning technologies coupled with cell sheet technology were developed.
4.1. Heterotypic cell–cell interaction in several co-culture systems
Hepatocytes cultured in vitro rapidly decrease in cell number and specific function within several days,71,72 and this is the fundamental problem facing liver tissue engineering. Hepatocyte–nonparenchymal cell communication was investigated in an in vitro random co-culture system, and some nonparenchymal cells were found to assist in the functional survival of hepatocytes.73–75 In an attempt to resolve the problem, a co-culture system using hepatocytes and endothelial cells (ECs) in a combination of cell sheet technology and cell micro-patterning technology was investigated. A double-layered co-culture system was successful by layering an EC sheet prepared on a temperature-responsive culture dish, directly onto a hepatocyte layer.76 In the double-layered cell structure, ECs and hepatocytes are in close contact with each other, and the hepatocytes maintained their differentiated cell shape and albumin secreting function for more than 40 days; whereas the secreting function stopped within 10 days when hepatocytes were cultured alone.76 The long-term functional maintenance and survival of hepatocytes were shown to be induced by the direct contact with ECs.76 Furthermore, we developed more “intelligent culture surfaces”, which enables the co-culture of patterned heterogeneous cells.20,21,23,27 The copolymer combining IPAAm and n-butyl methacrylate (BMA) shows a lower LCST than that of PIPAAm (32 °C),20 and the differences in their LCST characteristics allow a patterned dual temperature-responsive surface to be created. A PIPAAm-grafted surface having pattered P(IPAAm-BMA) co-grafted islands, namely a patterned dual temperature-responsive surface, can be fabricated by using sequential EB irradiation and a porous metal mask.21,23 At 27 °C, seeded hepatocytes attached exclusively onto the hydrophobic, dehydrated P(IPAAm-BMA) co-grafted domains, while the cells were unable to attach onto neighboring hydrated PIPAAm domains.21,23 Subsequently seeded ECs then attached exclusively to the hydrophobic PIPAAm regions by increasing the culture temperature to 37 °C, thus achieving patterned co-cultures.21,23 An immunofluorescent assay using albumin antibody showed the successful co-culture of hepatocytes and ECs without any invasion of either cell type into different domains.21,23 The completed heterotypic cell sheet containing exactly patterned ECs and hepatocytes can then be harvested by simply reducing the temperature to 20 °C.21,23 The harvested heterotypic cell sheet can then be layered onto another cell sheet. Our laboratory also successfully developed a micro-patterned co-culture system containing hepatocytes and ECs with a microcontact printing system using elastomeric polydimethylsiloxane stamps and the cell adhesion protein fibronectin.27 The cell density of hepatocytes increases at the periphery in the patterned co-cultured system, which suggests that heterotypic cell–cell interactions in a combination of hepatocytes and ECs lead to a proliferation of hepatocytes. These results suggest that DNA synthesis in hepatocytes is stimulated by direct cell–cell interaction, which may be important in repairing liver damage and liver regeneration. These novel co-culture methods are shown to work in liver tissue and can also be used to re-construct other complex heterogeneous tissues. For example, the brain–blood barrier, small intestinal mucosa, and kidney glomerulus, which are all pharmacologically and pharmacokinetically important, may be re-constructed and could provide optimal in vitro tissue models for drug development. Furthermore, prevascularized tissue can also be fabricated as described in the following chapter.
4.2. Micro-patterned ECs and the fabrication of pre-vascularized tissues
At present, cell sheet technology for fabricating 3D tissues has some limitations in scaling-up. While the fabrication of a large two-dimensional tissue can be easily overcome by using larger temperature-responsive culture surfaces, thicker 3D tissues in vivo and in vitro are unable to survive for very long because of insufficient nutrients and the accumulation of waste products. Actually the thickness limit for layered cell sheets in vivo is approximately 70–80 μm.77 For in vivo application, a polysurgery method using cell sheets is one solution to the problem since 1 mm thick cell-dense tissue can be easily fabricated.77 To fabricate thicker tissue in vitro, one strategy is the creation of blood capillary-like networks into tissue-engineered constructs. Our laboratory developed a maskless photolithography device to fabricate cell micro-patterned surfaces by modifying a commercially available liquid crystal display (LCD) projector.22,25 A linearly micro-patterned temperature-responsive cell culture surface [PIPAAm/polyacrylamide (PAAm)] was developed by using the maskless photolithography device and a two-step polymerization method (Fig. 2).24 Only the PIPAAm-grafted regions on the micro-patterned surfaces show temperature-dependent contact angle changes, which are hydrophobic at 37 °C and hydrophilic at 20 °C, and the PAAm regions are very hydrophilic at both temperatures.24 When ECs were seeded onto the 20 μm wide micro-patterned surfaces at 37 °C, the cells only attached to the PIPAAm-grafted surface; they also elongated and aligned with their own long axis to be parallel to the micro-pattern.24 Though the patterned ECs can also be detached by lowering the temperature to 20 °C, the morphology of ECs changes from elongated to spherical, and the pattered ECs eventually aggregate and lift off the PIPAAm-grafted domains.24 The aggregation and shrinkage of patterned ECs after detaching could be solved by using a gelatin gel-coated plunger-like cell sheet layering manipulator.24,78–80 A multi-layered tissue construct containing patterned ECs can be fabricated by alternating recovered cell sheets and patterned ECs.24 After a few days of cultivation, the patterned ECs communicated with each other and self-organized into an EC patterned-microvasculature-like network within the 3D cell construct.24 In contrast, using patterned ECs alone they were unable to form a network and migrated randomly. A tubular-like structure of native microvasculature can be formed within 3D tissues containing ECs fabricated using different several co-culture systems.78–80 In addition, EC networks in the multi-layer cell sheet construct can anastomose to the host vessels after in vivo transplantation, resulting in a neovascularization that allows the graft to survive.79,81 Moreover, the transplantation of the pre-vascularized cell sheet constructs onto infarcted rat hearts induced significant improvement in heart functions.81 These techniques and findings should encourage further studies for fundamental cell–cell communication and angiogenesis mechanisms in re-constructed 3D environments as well as the fabrication of complicated, vascularized, and functional tissues required in tissue engineering and regenerative medicine. These methods should enable many strategic design breakthroughs for the next generation of tissue engineering and regenerative medicine.
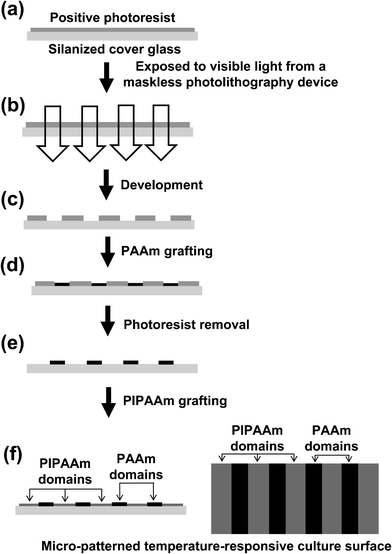 |
| Fig. 2 Schematic illustrations for the fabrication of a temperature-responsive micro-patterned surface. A photoresist pattern on a silanized cover glass surface is fabricated by using a maskless photolithography device (a–c). The cover glass is placed into acrylamide (AAm) solution, and then PAAm is selectively covalently grafted onto the non-photoresis-coated regions of the glass surface (d). After removing the photoresist from the cover glass, the substrate is placed into IPAAm solution and the PIPAAm is then covalently grafted onto the non-PAAm-grafted regions of the glass surface (e, f). | |
In the near future, in vitro fabrication of millimetre-order functional and heterogeneous organ-like tissue constructs having micro-vascular networks could be produced by a combination of several technologies. Combining the fabrication of heterotypic cell sheets using cell micro-pattering technology and the cell sheet stacking technology described in this review may be the basis for future fabrication of “bioengineered heart, liver, and kidney”. However, it is still a distant goal that will require greater development and even breakthroughs in many technologies, including interdisciplinary fields.
5. Conclusions
Cell sheet and cell micro-patterning technologies have been developed and advanced by chemical science, including several interdisciplinary fields. These technologies have enormous and wide-ranging potentials for the re-construction of various tissues, including complicated heterogeneous and vascularised tissues, and clinical trials using cell sheet technology have been performed in several tissues.
Acknowledgements
We appreciate the useful comments and technical critique of Dr Norio Ueno (Institute of Advanced Biomedical Engineering and Science, TWIns, Tokyo Women's Medical University). This work was supported by grants from the Center of Excellence (COE) Program for the 21st Century, the Global COE Program, Multidisciplinary Education and Technology and Research Center for Regenerative Medicine (MERCREM), Innovation Center for Fusion of Advanced Technologies in the Special Coordination Funds for Promoting Science, and the High-Tech Research Center Program from the Ministry of Education, Culture, Sports Science, and Technology (MEXST), Japan, and the Japan Society for the Promotion of Science (JSPS) through the “Funding Program for World-Leading Innovative R&D on Science and Technology (FIRST Program),” initiated by the Council for Science and Technology Policy (CSTP).
References
- G. B. Boncoraglio, A. Bersano, L. Candelise, B. A. Reynolds and E. A. Parati, Cochrane Database Syst. Rev., 2010, 8, CD007231 Search PubMed.
- A. M. Mozid, S. Arnous, E. C. Sammut and A. Mathur, Br. Med. Bull., 2011, 98, 143–159 Search PubMed.
- W. Y. Zhang, A. D. Ebert, J. Narula and J. C. Wu, J. Cardiovasc. Transl. Res., 2011, 4, 514–522 Search PubMed.
- T. Leclerc, C. Thepenier, P. Jault, E. Bey, J. Peltzer, M. Trouillas, P. Duhamel, L. Bargues, M. Prat, M. Bonderriter and J. J. Lataillade, Cell Prolif., 2011, 44(Suppl 1), 48–54 Search PubMed.
- N. Forraz and C. P. McGuckin, Cell Prolif., 2011, 44(Suppl 1), 60–69 Search PubMed.
- J. W. Lee, X. Fang, A. Krasnodembskaya, J. P. Howard and M. A. Matthay, Stem Cells, 2011, 29, 913–919 Search PubMed.
- C. Del Corsso and A. C. Campos de Carvalho, Braz. J. Med. Biol. Res., 2011, 44, 388–393 Search PubMed.
- A. Jablonska and B. Lukomska, Acta Neurobiol. Exp. (Wars), 2011, 71, 74–85 Search PubMed.
- E. Jones and X. Yang, Injury, 2011, 42, 562–8 Search PubMed.
- M. E. Bernardo, D. Pagliara and F. Locatelli, Bone Marrow Transplant., 2011 Apr 11 Search PubMed.
- M. Hofmann, K. C. Wollert, G. P. Meyer, A. Menke, L. Arseniev, B. Hertenstein, A. Gasner, W. H. Knapp and H. Drexler, Circulation, 2005, 111, 2198–2202 CrossRef.
- G. Vunjak-Novakovic, K. O. Lui, N. Tandon and K. R. Chien, Annu. Rev. Biomed. Eng., 2010, 13, 245–267 Search PubMed.
-
A. Atala, R. Lanza, J.A. Thomson and R. Nerem, Principles of Regenerative Medicine. 2nd Ed. ed. (Academic Press, 2011 Search PubMed.
- R. Langer and J. P. Vacanti, Science, 1993, 260, 920–926 CrossRef CAS.
- T. Shinoka and C. Breuer, J. Biol. Med., 2008, 81, 161–166 Search PubMed.
- J. Iwasa, L. Engebretsen, Y. Shima and M. Ochi, Knee Surg. Sports Traumatol. Arthrosc., 2009, 17, 561–577 Search PubMed.
- K. Lee, C. K. Chan, N. Patil and S. B. Goodman, J. Biomed. Mater. Res. B. Appl. Biomater., 2009, 89, 252–263 Search PubMed.
- N. Yamada, T. Okano, H. Sakai, F. Karikusa, Y. Sawasaki and Y. Sakurai, Makromol. Chem. Rapid Commun., 1990, 11, 571–576 CrossRef CAS.
- T. Okano, H. Yamada, H. Sakai and Y. Sakurai, J. Biomed. Mater. Res., 1993, 27, 1243–1251 CrossRef CAS.
- Y. Tsuda, A. Kikuchi, M. Yamato, Y. Sakuri, M. Umezu and T. Okano, J. Biomed. Mater. Res., 2004, 69A, 70–78 Search PubMed.
- Y. Tsuda, A. Kikuchi, M. Yamato, A. Nakao, Y. Sakuri, M. Umezu and T. Okano, Biomaterials, 2005, 26, 1885–1893 CrossRef CAS.
- K. Itoga, J. Kobayashi, M. Yamato, A. Kikuchi and T. Okano, Biomaterials, 2006, 27, 3005–3009 CrossRef CAS.
- Y. Tsuda, A. Kikuchi, Y. Yamato, G. Chen and T. Okano, Biochem. Biophys. Res. Commun., 2006, 348, 937–944 CrossRef CAS.
- Y. Tsuda, T. Shimizu, M. Yamato, G. Chen, T. Okano, A. Kikuchi, T. Sasagawa, S. Sekiya, J. Kobayashi, G. Chen and T. Okano, Biomaterials, 2007, 28, 4939–4946 CrossRef CAS.
- K. Itoga, J. Kobayashi, Y. Tsuda, M. Yamato and T. Okano, Anal. Chem., 2008, 80, 1323–2327 CrossRef CAS.
- I. E. Hannachi, M. Yamato and T. Okano, Biofabrication, 2009, 1, 022002 Search PubMed.
- I. E. Hannachi, K. Itoga, Y. Kumashiro, J. Kobayashi, M. Yamato and T. Okano, Biomaterials, 2009, 30, 5427–5432 CrossRef CAS.
- I. E. Hannachi, M. Maeda, M. Yamato and T. Okano, Biomaterials, 2010, 31, 8974–8979 CrossRef CAS.
- M. Heskins, J. E. Guillet and E. James, J. Macromol. Chem., 1968, A2, 1441–1445 Search PubMed.
- N. Matsuda, T. Shimizu, M. Yamato and T. Okano, Adv. Mater., 2007, 19, 3089–3099 CrossRef CAS.
- S. Masuda, T. Shimizu, M. Yamato and T. Okano, Adv. Drug Delivery Rev., 2008, 60, 277–285 Search PubMed.
- J. Yang, M. Yamato, T. Shimizu, H. Sekine, K. Ohashi, M. Kanzaki, T. Ohki, K. Nishida and T. Okano, Biomaterials, 2007, 28, 5033–5043 CrossRef CAS.
- Y. Akiyama, A. Kikuchi, M. Yamato and T. Okano, Langmuir, 2004, 20, 5506–5511 CrossRef CAS.
- A. Kushida, M. Yamato, C. Konno, A. Kikuchi, Y. Sakurai and T. Okano, J. Biomed. Mater. Res., 1999, 45, 355–362 CrossRef CAS.
- K. Nishida, M. Yamato, Y. Hayashida, K. Watanabe, N. Maeda, H. Watanabe, K. Yamamoto, S. Nagai, A. Kikuchi, Y. Tano and T. Okano, Transplantation, 2004, 77, 379–385 CrossRef.
- K. Nishida, M. Yamato, Y. Hayashida, K. Watanabe, K. Yamamoto, E. Adachi, S. Nagai, A. Kikuchi, N. Maeda, H. Watanabe, T. Okano and Y. Tano, N. Engl. J. Med., 2004, 351, 1187–1196 CrossRef CAS.
- Y. Hayashida, K. Nishida, M. Yamato, K. Watanabe, N. Maeda, H. Watanabe, A. Kikuchi, T. Okano and Y. Tano, Invest. Ophthalmol. Visual Sci., 2005, 46, 1632–1639 Search PubMed.
- R. Soetikno, T. Kaltenbach, R. Yeh and T. Gotoda, J. Clin. Oncol., 2005, 23, 4490–4498 Search PubMed.
- T. Oyama, A. Tomori, K. Hotta, S. Morita, K. Kominato, M. Tanaka and Y. Miyata, Clin. Gastroenterol. Hepatol., 2005, 3, S67–70 Search PubMed.
- H. Yamamoto, Clin. Gastroenterol. Hepatol., 2005, 3, S74–76 Search PubMed.
- C. Katada, M. Muto, T. Manabe, N. Boku, A. Ohtsu and S. Yoshida, Gastrointest. Endosc., 2003, 57, 165–169 Search PubMed.
- S. Seewald, T. Akaraviputh, U. Seitz, B. Brand, S. Groth, G. Mendoza, X. He, F. Thonke, M. Stolte, S. Schroeder and N. Soehendra, Gastrointest. Endosc., 2003, 57, 854–859 Search PubMed.
- T. Ohki, M. Yamato, D. Murakami, R. Takagi, J. Yang, H. Namiki, T. Okano and K. Takasaki, Gut, 2006, 55, 1704–1710 CrossRef CAS.
-
D. Lloyd-Jones, R. J. Adams, T. M. Brownet al., Circulation, 2010, 121, pp. 948–954 Search PubMed.
- P. Menasche, A. A. Hagege, M. Scorsin, B. Pouzet, M. Desnos, D. Duboc, K. Schwartz, J. T. Vilquin and J. P. Marolleau, Lancet, 2001, 357, 279–280 CrossRef CAS.
- K. Jujo, M. Ii and D. W. Losordo, J. Mol. Cell. Cardiol., 2008, 45, 530–544 Search PubMed.
- M. A. Alaiti, M. Ishikawa and M. A. Costa, Transl. Res., 2010, 156, 112–129 Search PubMed.
- T. Shimizu, H. Sekine, M. Yamato and T. Okano, Curr. Pharm. Des., 2009, 15, 2807–2814 Search PubMed.
- I. A. Memon, Y. Sawa, N. Fukushima, G. Matsumiya, S. Miyagawa, S. Taketani, S. K. Sakakida, H. Kondoh, A. N. Aleshin, T. Shimizu, T. Okano and H. Matsuda, J. Thorac. Cardiovasc. Surg., 2005, 130, 1333–1341 Search PubMed.
- H. Kondoh, Y. Sawa, S. Miyagawa, S. Sakakida-Kitagawa, I. A. Memon, N. Kawaguchi, N. Matsuura, T. Shimizu, T. Okano and H. Matsuda, Cardiovasc. Res., 2006, 69, 466–475 Search PubMed.
- H. Hata, G. Matsumiya, S. Miyagawa, H. Kondoh, N. Kawaguchi, N. Matsuura, T. Shimizu, T. Okano, H. Matsuda and Y. Sawa, J. Thorac. Cardiovasc. Surg., 2006, 132, 918–924 Search PubMed.
- Y. Miyahara, N. Nagaya, M. Kataoka, B. Yanagawa, K. Tanaka, H. Hao, K. Ishino, H. Ishida, T. Shimizu, K. Kangawa, S. Sano, T. Okano, S. Kitamura and H. Mori, Nat. Med., 2006, 12, 459–465 CrossRef CAS.
- N. Hida, N. Nishiyama, S. Miyoshi, S. Kira, K. Segawa, T. Uyama, T. Mori, K. Miyado, Y. Ikegami, C. Cui, T. Kiyono, S. Kyo, T. Shimizu, T. Okano, M. Sakamoto, S. Ogawa and A. Umezawa, Stem Cells, 2008, 26, 1695–1704 Search PubMed.
- K. Matsuura, A. Honda, T. Nagai, N. Fukushima, K. Iwanga, M. Tokunaga, T. Shimizu, T. Okano, H. Kasanuki, N. Hagiwara and I. Komouro, J. Clin. Invest., 2009, 119, 2204–2217 Search PubMed.
- N. Sekiya, G. Matsumiya, S. Miyagawa, A. Saito, T. Shimizu, T. Okano, N. Kawaguchi, N. Matsuura and Y. Sawa, J. Thorac. Cardiovasc. Surg., 2009, 138, 985–993 Search PubMed.
- S. Miyagawa, A. Saito, T. Sakaguchi, Y. Yoshikawa, T. Yamauchi, Y. Imanishi, N. Kawaguchi, N. Teramoto, N. Matsuura, H. Iida, T. Shimizu, T. Okano and Y. Sawa, Transplantation, 2010, 90, 364–372 Search PubMed.
- B. L. Pihlstrom, B. S. Michalowicz and N. W. Johnson, Lancet, 2005, 366, 1809–1820 Search PubMed.
- M. Hasegawa, M. Yamato, A. Kikuchi, T. Okano and I. Ishikawa, Tissue Eng., 2005, 11, 469–478 CrossRef CAS.
- T. Akizuki, S. Oda, M. Komaki, H. Tsuchioka, N. Kawakatsu, A. Kikuchi, M. Yamato, T. Okano and I. Ishikawa, J. Periodontal Res., 2005, 40, 245–251 CrossRef.
- M. G. Flores, M. Hasegawa, M. Yamato, R. Takagi, T. Okano and I. Ishikawa, J. Periodontal Res., 2008, 43, 364–371 CrossRef CAS.
- T. Iwata, M. Yamato, H. Tsuchioka, R. Takagi, S. Mukobata, K. Washio, T. Okano and I. Ishikawa, Biomaterials, 2009, 30, 2716–2723 CrossRef CAS.
- K. Washio, T. Iwata, M. Mizutani, T. Ando, M. Yamato, T. Okano and I. Ishikawa, Cell Tissue Res., 2010, 341, 397–404 Search PubMed.
- D. B. Loran, K. J. Woodside, R. J. Cerfolio and J. B. Zwischenberger, Chest. Surg. Clin. N. Am., 2002, 12, 477–488 Search PubMed.
- J. Belda-Sanchís, M. Serra-Mitjans, M. Iglesias Sentis and R. Rami, Cochrane Database Syst. Rev., 2010, 1, CD003051 Search PubMed.
- G. Malapert, H. A. Hanna, P. B. Pages and A. Bernard, Ann. Thorac. Surg., 2010, 90, 1779–1785 Search PubMed.
- M. Kanzaki, M. Yamato, J. Yang, H. Sekine, C. Kohno, R. Takagi, H. Hatakeyama, T. Isaka, T. Okano and T. Onuki, Biomaterials, 2007, 28, 4294–4302 CrossRef CAS.
- M. Kanzaki, M. Yamato, J. Yang, H. Sekine, R. Tagaki, T. Isaka, T. Okano and T. Onuki, Eur. J. Cardio-Thorac. Surg., 2008, 34, 864–869 CrossRef.
- T. L. van Belle, K. T. Coppieters and M. G. von Herrath, Physiol. Rev., 2011, 91, 79–118 CrossRef CAS.
- C. E. Couri and J. C. Voltarelli, Diabetol. Metab. Syndr., 2009, 1, 19 Search PubMed.
- H. Shimizu, K. Ohashi, R. Utoh, K. Ise, M. Gotoh, M. Yamato and T. Okano, Biomaterials, 2009, 30, 5943–5949 CrossRef CAS.
- C. Guguen-Guillouzo and A. Guillouzo, Mol. Cell. Biochem., 1983, 53–54, 35–56 Search PubMed.
- K. Ohashi, F. Park and M. A. Kay, J. Mol. Med., 2001, 79, 617–630 Search PubMed.
- S. N. Bhatia, M. L. Yarmush and M. Toner, J. Biomed. Mater. Res., 1997, 34, 189–199 CrossRef CAS.
- S. Bhatia, U. Balis, M. Yarmush and M. Toner, FASEB J., 1999, 13, 1883–1900 CAS.
- R. Bhandari, L. Riccalton, A. Lewis, J. R. Fry, A. H. Hammond, S. J. Tendler and K. M. Shakesheff, Tissue Eng., 2001, 7, 345–357 CrossRef CAS.
- M. Harimoto, M. Yamato, M. Hirose, C. Takahashi, Y. Isoi, A. Kikuchi and T. Okano, J. Biomed. Mater. Res., 2002, 62, 464–470 CrossRef CAS.
- T. Shimizu, H. Sekine, J. Yang, Y. Isoi, M. Yamato, A. Kikuchi, E. Kobayashi and T. Okano, FASEB J., 2006, 20, 708–710 CAS.
- S. Sekiya, M. Muraoka, T. Sasagawa, T. Shimizu, M. Yamato and T. Okano, Microvasc. Res., 2010, 80, 549–551 CrossRef.
- T. Sasagawa, T. Shimizu, S. Sekiya, Y. Haraguchi, M. Yamato, Y. Sawa and T. Okano, Biomaterials, 2010, 31, 1646–1654 Search PubMed.
- N. Asakawa, T. Shimizu, Y. Tsuda, S. Sekiya, T. Sasagawa, M. Yamato, F. Fukai and T. Okano, Biomaterials, 2010, 31, 3903–3909 Search PubMed.
- H. Sekine, T. Shimizu, K. Hobo, S. Sekiya, J. Yang, M. Yamato, H. Kurosawa, E. Kobayashi and T. Okano, Circulation, 2008, 118, S145–152 CrossRef CAS.
|
This journal is © The Royal Society of Chemistry 2012 |
Click here to see how this site uses Cookies. View our privacy policy here.