DOI:
10.1039/C2RA01090A
(Paper)
RSC Adv., 2012,
2, 4377-4381
Electrochromic properties of ITO nanoparticles/viologen composite film electrodes†
Received
15th November 2011
, Accepted 29th February 2012
First published on 30th March 2012
Abstract
Nanostructured composite electrodes were prepared by dip-coating a mixed dispersion of ITO nanoparticles and 1,1′-didodecyl-4,4′-bipyridinium (DDV2+) dibromide on an ITO-coated glass plate, and their electrochromic properties were investigated in a 0.1 M Li2SO4 aqueous solution. The electrodes showed a color change from pale white to violet as they were oxidized and reduced, respectively, and good electrochromic performances; i.e., switching times of coloration (500 ms) and decoloration (380 ms), coloration efficiency of 140 cm2 C−1, long-term stability (>500 test cycles), and a memory life time greater than at least 24 h.
1. Introduction
Many attempts to construct electrochromic devices and electronic papers have been performed utilizing electrochromism.1–6 In these studies, attempts to develop electrochromic devices or electronic papers by the combination of a non-aqueous solvent and a nanoporous–nanocrystalline titanium dioxide (TiO2) electrode, in which a viologen compound is chemically modified, have been brought to our attention.7–17 The features of these electrode systems are: (1) because the nanoporous electrode has a high real specific surface area, the amount of viologen chemisorption can be increased and, as a result, a sufficient coloration is capable of being reached; (2) because the colored species are chemically adsorbed, the electrode reaction process is less influenced by diffusion and a high-speed response becomes possible; (3) because the electrochemical one-electron reduction reaction of the viologen compound is highly reversible, it excels in its coloration–decoloration cyclic characteristics. However, introducing a substituent, such as a phosphate group or carboxyl group, to viologen in order to chemically adsorb the viologen compound onto the surface of the titanium dioxide nanoparticles is required. The synthetic viologen process is a simple procedure involving only the quaternization reaction of 4,4′-bipyridine. Therefore, if this process of the substituent introduction can be eliminated, it is expected that the efficiency of the production process of such devices can be improved.
In this paper, indium–tin-oxide (ITO) nanoparticles have only been mixed with a hydrophobic viologen compound, then the mixture was simply applied on a substrate to make a film electrode for the electrochromic device, followed by characterization in an aqueous solution. The motive for the present study is that if the viologen compound in the state of +2 valence is water-insoluble, the +1 valence viologen cation radical, which is its one-electron reduction species and the coloration species, will be more hydrophobic18 and should be water-insoluble. Thus, a stable operation can be expected for the coloration–decoloration cycles of the electrode. The purpose of this study is not only to achieve the above described improvement in the production process, but also to obtain an electrochemical result as to whether the reversible redox of the hydrophobic viologen embedded in the nanoparticle film is possible in an aqueous solution.
2. Experimental
2.1 Preparation of composite electrodes
ITO nanoparticles (Nanotek® Powder ITO-R, average particle size: 30 nm) were purchased from C. I. Kasei Co., Ltd.,19 and were used as received. The viologen compound used in this study was 1,1′-didodecyl-4,4′-bipyridinium (DDV2+) dibromide,20,21 which was synthesized according to a literature method.22–24 This viologen compound is soluble in organic solvents (1-butanol, methanol, ethanol, 2-propanol, methylethylketone, chloroform and dimethylformamide) and almost colorless and transparent. However, it becomes a colored cation radical by a one-electron reduction reaction.21 It returns to the initial transparent/colorless state by the re-oxidation reaction. The DDV2+ is highly hydrophobic and is water-insoluble.20,21 ITO nanoparticles (1 g) and DDV2+·2Br− (0.05 g) were added to 1-butanol (9 g), and they were subjected to supersonic treatment in the bath-type sonicator (AS ONE Co., model USK-2R) for 30 min or more to prepare a slurry. The coating of the slurry on an ITO substrate was done by the dip-coating method. The film thickness of the ITO nanoparticle/DDV2+·2Br− composite film (hereafter, abbreviated to ITO/DDV composite film) was adjusted by repeating the dipping and drying process at room temperature.
2.2 Electrochemical tests
The electrochromic properties were examined in a 0.1 M lithium sulfate (Li2SO4·H2O, Wako Pure Chemicals, >99%) aqueous solution. An electrolytic cell consisting of a square main compartment and a cylindrical sub-compartment, which were separated by a G4 glass filter, was used (see ESI†). A working electrode (ITO/DDV composite film electrode) and a counter electrode (ring-shaped Pt electrode) were placed in the main compartment. On the other hand, a KCl agar bridge connected to a reference electrode (saturated calomel electrode, SCE) was placed in the sub-compartment. The electrochemical behavior of DDV2+ was examined by cyclic voltammetry using an electrochemical analyzer (BAS Co., model 750A). The UV-vis spectrum was measured using a UV-vis spectrophotometer (Ocean Optics, Inc., USB4000-UV-vis spectrometer and DH-2000 deuterium tungsten halogen light source) and an optical fiber system at the same time as the cyclic voltammetry.
3. Results and discussion
Parts a and b in Fig. 1 shows the cyclic voltammograms of the ITO/DDV composite film electrode, and the plots of potential vs. absorbance at 512 nm (the absorption maximum of the colored film), respectively. The absorbance was simultaneously measured during the cyclic voltammetry. The results of the cyclic voltammetry (Fig. 1a) indicated that the shape of the reduction current, which started flowing at around −0.35 V and the shape of the oxidation current, which started flowing at around −0.45 V, were extremely stable during the cyclic potential sweep and, therefore, the ITO/DDV composite film is an extremely stable screen electrode. It is assumed that DDV2+·2Br− is retained in the nanospace, which is formed by the ITO nanoparticles. This assumption allows us to speculate that the ions can move freely in and out of the nanospace because the electrolyte sufficiently infiltrates into the nanospace without being purged by the hydrophobic DDV2+·2Br− and, thus, the electrode oxidation/reduction becomes possible. It is likely that the hydrophilic property on the surface of the ITO particles allows the infiltration of water into the nanospace. Similar results were obtained from part b in Fig. 1. The change in absorbance at 512 nm, which increased from around −0.35 V and decreased from around −0.45 V, was extremely reversible even if the potential sweep was repeated. In addition, the decolored state always returned to the initial value and an incomplete vanishment (ghost image formation) did not occur.
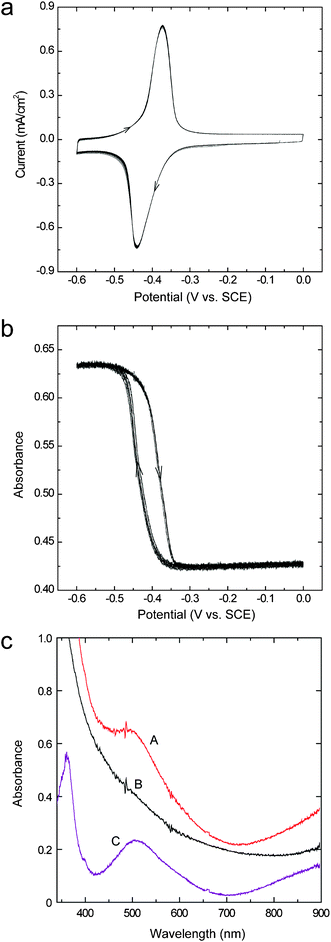 |
| Fig. 1 Successive cyclic voltammograms (a) and absorbance (λ = 512 nm)–potential relationship (b) of an ITO/DDV composite film electrode (2-μm thick) in a Li2SO4 aqueous solution (0.1 M). Sweep rate: 20 mV s−1. Part c shows the UV-vis absorption spectra of the colored state (A) and the decolored state of the ITO/DDV composite film electrode (2-μm thick) (B); curve C shows the difference spectrum obtained by subtracting curve B from curve A. | |
It is known that a water insoluble viologen cation radical is produced when the one-electron reduction of the viologen compound is carried out on a planar-type electrode in an aqueous solution, and that the dimerization reaction of the radical produces a purple deposit.25 The dimeric species undergoes re-crystallization to produce the deposit that is not completely available for a direct electrochemical oxidation. The result is that a high oxidation potential is needed, or the incomplete vanishment occurs due to an incomplete oxidation (ghost image formation).5,26–28 As for the above-described ITO/DDV composite film electrode, its coloration and decoloration are carried out in an extremely narrow potential range. Therefore, when a two-electrode-type cell is formed, it can be expected that the driving voltage of the coloration and decoloration will be very low. Moreover, because the incomplete vanishment does not occur, a high cyclic stability can be expected (see section 3.2). We consider that the reason why the incomplete vanishment does not occur is because DDV does not locally aggregate in the nanospace formed by the ITO nanoparticles and recrystallization does not occur.
Fig. 1c shows the UV-vis absorption spectra of the ITO/DDV composite film electrode in a colored state (A) and a decolored state (B). Spectrum C is the difference spectrum of spectrum A and spectrum B. The film thickness of the composite film was 2 μm. The colored state and decolored state were formed by applying a potential of −0.60 V and 0 V for five seconds, respectively. It is reported that the viologen cation radical dimer has absorption peaks at 360 nm and 520 nm, and that the viologen cation radical monomer has absorption peaks at 396 nm and 602 nm.29–31 Since spectrum C has peaks at 360 nm and 520 nm, it can be seen that DDV+ exists as a dimer. Presumably, because the dimerization is promoted by the fastener effect32 of the didodecyl group, it will be difficult for the cation radical monomer to exist. Additionally, the spectrum in the decolored state showed a curve specific to a light scattering phenomenon in which the absorbance increases as the wavelength decreases. This effect is desirable as the backgrounds of electrochromic devices and electronic papers.
3.2 Cyclic characteristics of coloration and decoloration
Part a in Fig. 2 shows the relation between the cycle number and the absorbance at 512 nm when the coloration and decoloration cycle was repeated using the ITO/DDV composite film electrode, and part b shows a partial enlargement of part a. It should be noted that the x-axis of part b is in not repeat count but time. The coloration potential and decoloration potential are −0.6 V and 0 V, respectively, and the time for applying the potential is 1 s. Both the attained absorbance of coloration and the attained absorbance of decoloration maintained almost the same value during the cyclic test and neither the ghost image formation nor deterioration of the film were observed. Deterioration has not been observed even after repeat cycles of 500, though a recording up to 150 cycles is indicated in Fig. 2a. In addition, it can be seen from Fig. 2b that the time required from the beginning to the end of the coloration (response time, tr) was 500 ms, and that the time required from the beginning to the end of the decoloration was 380 ms. These response times are comparable to the coloration (6 ms–3 s) and decoloration times (14.4 ms–3 s) of the TiO2 nanoparticles/chemically-modified viologen film electrodes,7–9,11,13–17 which were described in the introduction of this paper. The reason why such a high-speed response was achieved can be explained by the fact that the viologen was immobilized even when the viologen was merely mixed with the ITO nanoparticle film in which the electrochromic reaction takes place, and that the effect of the diffusion of the viologen and/or the ions that participate in the electrochromic reaction could be reduced by this immobilization. In addition, the memory lifetime in the colored state under a nitrogen atmosphere was at least 24 h or more.
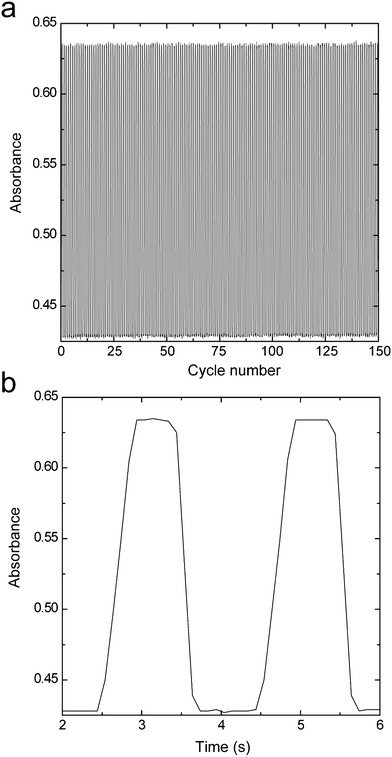 |
| Fig. 2 Coloration–decoloration cycles of the ITO/DDV composite electrode (2-μm thick) in a 0.1 M Li2SO4 aqueous solution between −0.60 V and 0 V at a switching interval of 2 s (a), and short term cycles extracted from part a (b). In part b, the absorbance is plotted as a function of time. | |
3.3 Effect of film thickness on electrochromic performances
In the previous sections, it was found that the effect of diffusion of the material could be reduced and a high-speed response could be made when a comparatively thin film of 2 μm was used. However, a thicker film is needed when it is required to increase the color density in the colored state and/or to increase the image contrast. Therefore, the effect of the diffusion of materials was examined for various film thicknesses. ITO/DDV composite film electrodes with film thicknesses of 2, 5, 9 and 13 μm were prepared and were subjected to cyclic voltammetry at various sweep rates, v. In general, when a material fixed on the electrode is subjected to cyclic voltammetry, it is known that the peak current Ip (in the present study, it corresponds to the reduction current of DDV2+ fixed in the composite film) is proportional to the nth power of the sweep rate, v. Also, when n = 1, the effect of diffusion of the materials can be neglected. On the other hand, the reaction rate is controlled by the diffusion of the materials when n = 0.5. Nomura et al. prepared composite film electrodes by mixing the polyion complex of the polymerized viologen, polystyrene sulfonic acid and SnO2/TiO2 fine particles and carried out their cyclic voltammetric measurements.33 They considered the coating state of the polyion complex by examining the relation between Ip and vn.
Part a in Fig. 3 shows the relation between Ip and vn for the ITO/DDV composite film electrode. The value of n was adjusted to have a proportional relation. The relation between the film thickness and the value of n calculated in this way is shown in part b. It can be seen from this figure that the value of n gradually approached 0.5 as the film thickness increased and that the reaction rate was gradually controlled by the diffusion of the materials. It may be that the rate control is caused by the diffusion of the counter ion species (Br− and/or SO42−) into and out of the film associated with the redox reaction of DDV. Based on these results, it was revealed that the effect of the ion diffusion into and out of the composite film became more significant as the film thickness increased. This suggests that the response speed will decrease as the film thickness decreases. The response time tr and coloration efficiency CE (amount of an increase in absorbance per unit charge injected) were then examined for the various film thicknesses. A coloration potential (−0.6 V) was applied to the composite films and the variation of the absorbance and the injected charge with time was measured (see ESI†). The value of tr was calculated from the time dependence of the absorbance. Next, the relation between the absorbance and the charge amount was derived from the time dependence of the absorbance and the time dependence of the applied electric charge and CE was calculated from its slope (part a in Fig. 4). Part b in Fig. 4 shows the film-thickness dependence of CE and tr obtained in this way. It is demonstrated that the coloration was completed within one second for a film thickness of 5 μm or less and that a high-speed response was achieved. However, the response time became one second or more when the film thickness exceeded 5 μm. This may be because the thicker the film is, the more pronounced the effect of the diffusion of the counter ion. The value of CE was 140 cm2 C−1 for the film thickness of 2 μm, which was comparable to the average value of CE for conventional TiO2 nanoparticle/chemically-modified viologen film electrodes.7–9,17 However, this value is smaller than that of CE = 912 cm2 C−1 obtained in the most-recently reported device, which had a cathode of a TiO2 nanoparticle/chemically-modified viologen film electrode and an anode of a Prussian Blue/antimony–tin oxide nanocomposite film electrode.16 The value of CE decreased with an increase in the film thickness. This is probably because a part of the DDV+ was shielded due to the light scattering by the pale white ITO nanoparticles.
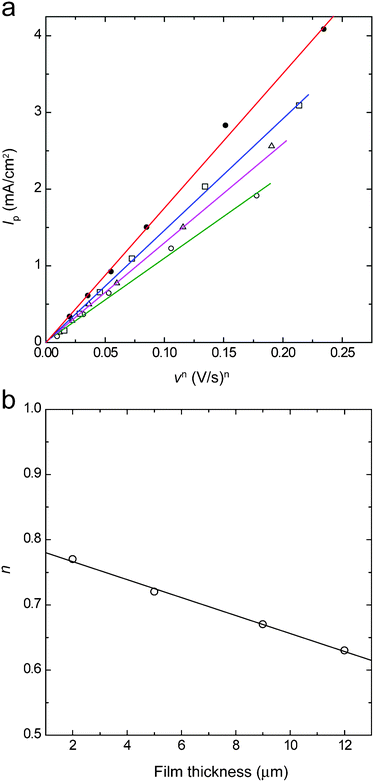 |
| Fig. 3 A plot of Ipvs. vn for the ITO/DDV composite electrodes with thicknesses of 2, 5, 9 and 13 μm (a) and the dependence of n on the film thickness (b). | |
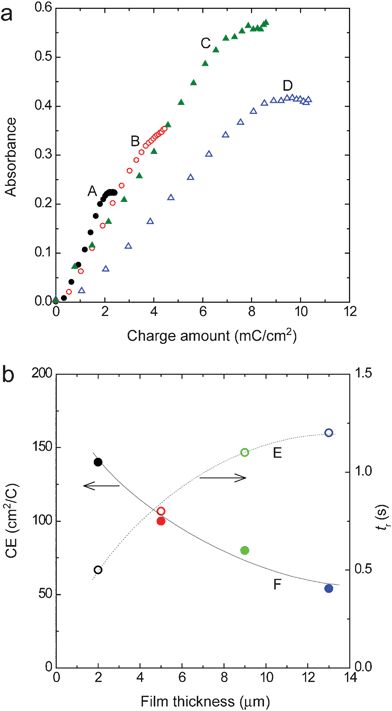 |
| Fig. 4 A plot of absorbance (λ = 512 nm) vs. charge amount for the ITO/DDV composite electrodes with thicknesses of 2 (A), 5 (B), 9 (C) and 13 μm (D) (a), and the dependence of tr (E) and CE (F) on the film thickness (b). | |
4. Conclusions
A nanocomposite film electrode was prepared only by mixing ITO nanoparticles with the hydrophobic viologen (DDV) and applying the mixture to a substrate. The electrochromic property of the electrode was then investigated in an aqueous solution. The electrode exhibited an electrochromism such that the color changed between pale white and purple. The coloration and decoloration occurred within a very small potential range and the incomplete vanishment (ghost image formation) did not occur (section 3.1). The nanocomposite film electrode had an extremely excellent coloration–decoloration cyclic durability and no deterioration was observed after the coloration–decoloration cyclic operation of at least 500 cycles. The nanocomposite film electrode with a thickness of 2 μm exhibited a high-speed response and the response time of the coloration and decoloration was 500 ms and 380 ms, respectively (section 3.2). The thickness dependence of the electrochemical behavior, the response time and coloration efficiency were examined on the nanocomposite film electrode. As the film thickness increased, the response time of the coloration increased and the coloration efficiency decreased. Based on the cyclic voltammetry results, it was found that the effect of the diffusion of the materials became significant as the film thickness increased. The decrease in the coloration efficiency was explained by assuming that a part of the DDV+ was shielded due to an increase in the light scattering by the pale white ITO nanoparticles along with an increase in the film thickness. The coloration efficiency of the 2-μm thick nanocomposite film electrode was 140 cm2 C−1 (section 3.3).
References
- C. J. Schoot, J. J. Ponjeé, H. T. van Dam, R. A. van Doorn and P. J. Bolwijn, Appl. Phys. Lett., 1973, 23, 64 CrossRef CAS.
- J. Bruinink, C. G. A. Kregting and J. J. Ponjeé, J. Electrochem. Soc., 1977, 124, 1854 CrossRef CAS.
- J. Bruinink and C. G. A. Kregting, J. Electrochem. Soc., 1978, 125, 1397 CrossRef CAS.
- S. Fletcher, L. Duff and R. G. Barradas, J. Electroanal. Chem., 1979, 100, 759 CrossRef CAS.
- R. J. Mortimer, Electrochim. Acta, 1999, 44, 2971 CrossRef CAS.
- R. J. Mortimer, A. L. Dyer and J. R. Reynolds, Displays, 2006, 27, 2 CrossRef CAS.
- R. Cinnsealach, G. Boschloo, S. N. Rao and D. Fitzmaurice, Sol. Energy Mater. Sol. Cells, 1999, 57, 107 CrossRef CAS.
- P. Bonhôte, E. Gogniat, F. Campus, L. Walder and M. Grätzel, Displays, 1999, 20, 137 CrossRef.
- D. Cummins, G. Boschloo, M. Ryan, D. Corr, S. N. Rao and D. Fitzmaurice, J. Phys. Chem. B, 2000, 104, 11449 CrossRef CAS.
- M. Grätzel, Nature, 2001, 409, 575 CrossRef.
- U. Bach, D. Corr, D. Lupo, F. Pichot and M. Ryan, Adv. Mater., 2002, 14, 845 CrossRef CAS.
- J. García-Cañadas, L. M. Peter and K. G. Upul Wijayantha, Electrochem. Commun., 2003, 5, 199 CrossRef.
- H. Pettersson, T. Gruszecki, L.-H. Johansson, M. O. M. Edwards, A. Hagfeldt and T. Matuszczyk, Displays, 2004, 25, 223 CrossRef.
- H. K. Jheong, Y. J. Kim, J. H. Pan, T.-Y. Won and W. I. Lee, J. Electroceram., 2006, 17, 929 CrossRef CAS.
- H. J. Kim, J. K. Seo, Y. J. Kim, H. K. Jeong, G. I. Lim, Y. S. Choi and W. I. Lee, Sol. Energy Mater. Sol. Cells, 2009, 93, 2108 CrossRef CAS.
- Y. Rong, S. Kim, F. Su, D. Myers and M. Taya, Electrochim. Acta, 2011, 56, 6230 CrossRef CAS.
- N. N. Dinh, N. M. Quyen, D. N. Chung, M. Zikova and V.-V. Truong, Sol. Energy Mater. Sol. Cells, 2011, 95, 618 CrossRef.
- K. Hoshino, H. Sasaki, K. Suga and T. Saji, Bull. Chem. Soc. Jpn., 1987, 60, 1521 CrossRef CAS.
-
http://www.cik.co.jp/product/nanotek/english/index.html
.
- K. Hoshino and T. Saji, Chem. Lett., 1988, 1439 Search PubMed.
- K. Hoshino, T. Karato, S.-K. Kim, Y. Yamada and T. Ebine, Electrochim. Acta, 2009, 53, 7655 CrossRef.
- M.-P. Pileni, A.M. Braun and M. Grätzel, Photochem. Photobiol., 1980, 31, 423 CrossRef CAS.
- M. Suzuki, M. Kimura, K. Hanabusa and H. Shirai, Macromol. Chem. Phys., 1998, 199, 945 CrossRef CAS.
- T. Abe, T. Fujita, K. Sekimoto, A. Tajiri and M. Kaneko, J. Mol. Catal. A: Chem., 2003, 201, 55 CrossRef CAS.
- C. L. Bard and A. T. Kuhn, Chem. Soc. Rev., 1981, 10, 49 RSC.
- A. Yasuda, H. Mori, Y. Takehana and A. Ohkoshi, J. Appl. Electrochem., 1984, 14, 323 CrossRef CAS.
- P. M. S. Monk, J. Electroanal. Chem., 1997, 432, 175 CrossRef CAS.
- K. Hoshino, Y. Oikawa, I. Sakabe and T. Komatsu, Electrochim. Acta, 2009, 55, 165 CrossRef CAS.
- C. Lee, M. S. Moon and J. W. Park, J. Electroanal. Chem., 1996, 407, 161 CrossRef.
- J. W. Park, N. H. Choi and J. H. Kim, J. Phys. Chem., 1996, 100, 769 CrossRef CAS.
- C. Lee, C. Kim and J. W. Park, J. Electroanal. Chem., 1994, 374, 115 CrossRef CAS.
- H. Inokuchi, G. Saito, K. Seki, P. Wu, T. B. Tanf, T. Mori, K. Imaeda, T. Enoki, Y. Higuchi, K. Inaka and N. Yasuoka, Chem. Lett., 1986, 1263 CrossRef CAS.
- K. Nomura, T. Masumi and K. Hirayama, Hyomen Kagaku, 1986, 7, 336 CrossRef CAS.
Footnote |
† Electronic supplementary information (ESI) available: Cell structure and coloration efficiency data. See DOI: 10.1039/c2ra01090a |
|
This journal is © The Royal Society of Chemistry 2012 |
Click here to see how this site uses Cookies. View our privacy policy here.