DOI:
10.1039/C2RA21453A
(Paper)
RSC Adv., 2012,
2, 9106-9113
Influence of curcumin on the Al(III)-induced conformation transition of silk fibroin and resulting potential therapy for neurodegenerative diseases†‡
Received
13th July 2012
, Accepted 7th August 2012
First published on 24th August 2012
1. Introduction
There are some important neurodegenerative diseases including Alzheimer's disease, prion disease and Parkinson's disease seriously threatening human health.1 These diseases involve the disorder of protein folding in which normally soluble proteins are deposited as abnormally insoluble amyloid fibrils that disrupt tissue structure and cause disease.2 It is well known that the amyloid fibrils mainly consisting of cross-β structure3 have a core formed by the antiparallel β-strand sheets perpendicular to the long axis of fibril.4 Prevention of the amyloid fibrillation and disaggregation of the pre-formed amyloid fibrils would be efficient approaches to treat neurodegenerative diseases.
Metal ions including Cu2+, Zn2+, Al3+, Fe3+ and Mn2+ have been widely demonstrated to be implicated as potential risk cofactors in neurodegenerative disorders.5 Postmortem analysis of brain tissues from patients with neurodegenerative disorders confirmed the involvement of those metals.6 These metals were demonstrated to trigger structural transformations, aggregation, and fibrillation of human α-synuclein and amyloid-β peptide in vitro.7,8 Among those metal ions, Al(III) is the most harmful metal to accelerate considerably the formation of amyloid-β peptide or α-synuclein fibrils.7,8 Therefore, understanding of the protein folding modulated by metal ions would be significant to develop an approach to inhibit the fibrillation.
To date, some small molecules, peptides and glycosaminoglycan analogues have been explored to treat neurodegenerative diseases.9–11 Among those compounds, curcumin (Fig. 1), a natural product, has potent antioxidant and anti-inflammatory activities, potential as a chemotherapeutic agent of cancer and neurodegenerative diseases.12,13 Previous studies have shown that curcumin can interact with amyloid-β peptide and α-synuclein, thus inhibiting their aggregation, deposition, and neurotoxicity.14,15 In addition, curcumin can readily chelate metal ions,16–21 such as Cu2+, Zn2+, Al3+, Fe3+ and Mn2+, to form the metal–curcumin complexes which have inhibitory potency on metal-induced neurotoxicity, and play the protective roles against stress, free radical and neuronal damage.15,16,18,21 Though curcumin has been demonstrated safe and efficient as a chemotherapeutic agent, its bioavailability is severely curtailed and its pharmaceutical application is also restricted because of its low water solubility, inadequate tissue absorption and degradation at alkaline pH.22 Since those disadvantages of curcumin, modification of curcumin structure and development of potential carriers for curcumin delivery are important approaches to improve its aqueous solubility, stability and bioavailability.23,24
Silk fibroin (SF) from insects such as spiders and Bombyx mori silkworm has been applied for drug delivery devices because of its good mechanic and bioactive properties.25,26 On account of its special primary and secondary structures, SF can be used as a sophisticated carrier.27 SF herein from Bombyx mori silkworm is dominantly comprised of the heavy-chain fibroin in addition to the light-chain fibroin including sericin and P25.28,29 Four types of repetitive sequence are mainly found in the heavy-chain silk fibroin, i.e., GAGAGS, GAGAGY, GAGAGVGY, forming hydrophobic domains, and GTGSSGFGPYVA(N/H)GGYSGYEYAWSSESDFGT forming fairly conserved hydrophilic spacers.30,31 The amino acid sequences enable SF to form an antiparallel β-pleated sheet structure which is remarkably analogous to that of the amyloid fibrils in neurodegenerative diseases. Nucleation-dependent mechanism was suggested for the aggregation and fibrillation of SF in our previous work,32 which is also extremely similar to that of the neurodegenerative disease-related proteins.33 In fact, the typical hydrophobic sequences of VGGVV existing in amyloid-β 42/40 peptide and VGGAVVAGV existing in α-synuclein are similar to the sequence of GAGVGAG abundant in SF.31 All of these peptide sequences have been identified essential and sufficient for the protein fibrillation,34 leading to the formation of antiparallel β-pleated sheet in the fiber.35,36 Furthermore, metal ions, such as Ca2+, Cu2+ and Zn2+, at certain concentrations37–39 are also found to promote the conformation transition of SF from random coil to β-sheet and accelerate the aggregation of SF in the same way as that of neurodegenerative proteins. Therefore SF, easily available from large amounts of silkworm fibers, could be used to serve as a model protein to explore the assembly of those proteins involved in neurodegenerative disorders.11
In the present study, we attempt to develop an approach treating neurodegenerative diseases on the molecular basis by investigations of the potency of curcumin on Al(III)-induced SF conformation transition using spectroscopic methods including attenuated total reflection-Fourier transform infrared spectroscopy (ATR-FTIR), solid-state 13C cross-polarization/magic-angle spinning (CP/MAS) NMR, and solid-state 1H combined rotation and multiple pulse spectroscopy (solid-state 1H CRAMPS) NMR.
2. Materials and methods
2.1 Material
Cocoon of Bombyx mori silkworm was purchased from Zhouquan Yuxing Textile Mill, China. Curcumin and trishydroxymethyl aminomethane (Tris) were purchased from Sigma-Aldrich agent, China. Lithium bromide (LiBr), silver nitrate (AgNO3), anhydrous sodium carbonate (Na2CO3), hydrochloric acid (HCl) and aluminum chloride (AlCl3) were purchased from Sinopharm, China.
2.2 Preparation of regenerated SF
Raw silk fibers from cocoons were boiled 40 min twice in 0.5 wt% Na2CO3 solution to remove sericin, and then thoroughly rinsed with ultra-pure deionized water (resistivity ∼18.2 MΩ·cm) and dried at 60 °C in drying oven overnight. The degummed silk fibers (6 g) were dissolved in 100 mL 9.3 M LiBr aqueous solution. After the silk fibers were dissolved completely, the solution was filtered and dialyzed against ultra-pure deionized water using a 14
000 g mol−1 dialysis tube to remove LiBr which was probed by 0.1 M AgNO3 aqueous solution. The final concentration of the aqueous SF solution was about ∼1.5 wt%.
2.3 Preparation of mixture samples of SF, Al(III) and curcumin
For study of Al(III) interaction with SF, SF solutions were mixed with 10 mM AlCl3 aqueous solution (pH = 4.5) of various volumes and then diluted by Tris-HCl aqueous solution (20 mM, pH = 7.4) to 15 mL mixture at SF concentration of 5 mg mL−1 and Al(III) concentration of 0, 5, 10, 20, 40, 60, 80, 100, 120, 160, 200 μM, respectively. Because of the low water solubility of curcumin, 5% (v/v) ethanol was added into each curcumin-contained sample for increasing the solubility of curcumin but without denaturalizing the protein.40 For preparation of curcumin/SF samples, 5 mg mL−1 SF solutions were mixed with curcumin of 0, 2.5, 5, 7.5, 10, 15, 20, 30, 40, 50, 60 μM, respectively. For preparation of Al(III)/curcumin/SF samples, 5 mg mL−1 SF solutions were mixed with 80 μM Al(III), and curcumin of 0, 20, 40, 60, 80, 100, 120, 140, 160, 180, 200 μM, respectively. When preparation of Al/curcumin/SF samples, SF solutions were incubated with Al(III) for 3 h to allow it interacting with Al(III) firstly, and then curcumin was added into the Al(III)/SF solutions. In addition, for preparation of Al(III)/curcumin/SF samples with [Al(III)]/[curcumin] ratio at 1
:
1, 5 mg mL−1 SF solutions were mixed with Al(III)/curcumin mixtures with equal Al(III) and curcumin concentrations of 0, 20, 30, 40, 50, 60, 70, 80 μM, respectively. Similarly, Al(III)/curcumin/SF samples with [Al(III)]/[curcumin] ratios at 1
:
1.5, 1
:
2 and 1
:
3 were prepared. When preparation of Al(III)/curcumin/SF samples with different [Al(III)]/[curcumin] ratio, curcumin was incubated with Al(III) for 2 h to allow it interacting with Al(III) firstly, and then the Al(III)/curucmin mixture was added into SF solutions. All of the above samples were incubated for an additional 6 h at room temperature, and then the resulting solutions were cast onto 30 × 30 mm polyester dishes at room temperature for 2 days in a fume hood for preparation of the silk-based films.
2.4 ATR-FTIR spectrum
ATR-FTIR were recorded using a Nicolet Nexus 470 infrared spectrometer equipped with an attenuated total reflection crystal cell of ZnSe in multiple-reflection mode and 128 scans at a resolution of 4 cm−1, wavenumber from 4000 to 675 cm−1.
2.5 Solid-state 13C CP/MAS NMR
Solid-state 13C CP/MAS NMR spectra of SF-based films were recorded on Varian Infinity-Plus 300 spectrometer with 1H resonance frequency of 300 MHz. The solid state samples were loaded into a 4 mm diameter ZrO2 spin rotor. All spectra were recorded using 1H and 13C cross polarization pulse sequence with magic-angle spinning. NMR measurements were performed using cross polarization contact time of 2.5 ms, pulse repeat delay of 3.0 s, 1H 90° pulse width of 4 μs, rotor spinning rate of 10 kHz and the scanning accumulations of 1000. The adamantane was used as an external reference.
2.6 Solid-state 1H CRAMPS NMR
Solid-state 1H CRAMPS NMR spectra were recorded on Varian Infinity-Plus 300 spectrometer with 1H resonance frequency of 300 MHz. A conventional 4 mm double-resonance HX CP/MAS NMR probe was used for all CRAMPS experiments, and the sample was placed in a 4 mm diameter ZrO2 spin rotor. DUMBO-1 pulse sequence41 was used for 1H homonuclear decoupling with cycle time of 32 μs divided by 64 discrete phase steps with 500 ns duration each, 1H RF field strength of 100 kHz, magic angle spinning rate of 9.8 ± 2 Hz. 1H chemical shift was calculated using an experimentally determined scaling factor of 0.58 for alanine.
3. Results
3.1 Effect of Al(III) on the conformation transition of SF
The infrared absorption frequency of amide groups in proteins is quite sensitive to the secondary structure. Infrared spectral region within 1700∼1600 cm−1, namely amide I, showing high intensity and little interference from other group vibrations compared with amide II (1600∼1500 cm−1) and amide III (1330∼1220 cm−1), thus it is widely used to analyze the conformation change of proteins. In amide I band, the adsorption peaks are generally assigned as: 1653 ± 4 cm−1 to helix, 1645 ± 4 cm−1 to random coil, 1625 ± 5 cm−1 and 1675 ± 5 cm−1 to β-sheet, 1663 ± 4 cm−1 to β-turn.42Fig. 2A shows the spectra of SF samples incubated with Al(III) of various concentrations, which were normalized to 1652 cm−1 corresponding to helix or random coil conformers. It was found that the absorption intensity at 1625 cm−1 corresponding to antiparallel β-sheet increased firstly and then decreased as the Al(III) concentration increased over 100 μM.
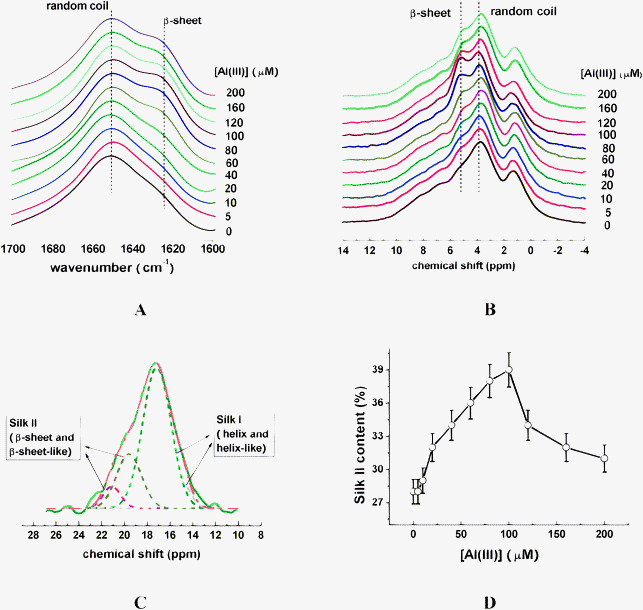 |
| Fig. 2 FT-IR (A), and 1H CRAMPS (B) spectra of silk fibroin in the presence of Al(III) at various concentrations. (C) One of the deconvoluted 13C CP/MAS NMR spectra of Ala Cβ using Gaussian line-shape, solid curve (in olive) and dash dot curve (in orange) represent the experimental and simulated spectra, respectively. The shot dashed curves represent the deconvoluted peaks for different secondary structures. (D) Dependence of Silk II content containing β-sheet-like and β-sheet conformers on Al(III) concentration. | |
It is well known that the chemical shifts of 1H and 13C nuclei are strongly influenced by the local conformation of a protein. 1H CRAMPS is one efficient technique used to obtain high-resolution solid-state 1H NMR spectra. Shoji and Kimura studied the correlation between 1H chemical shifts and conformations of polypeptides and SF on 1H CRAMPS NMR.43,44 They found that Hα chemical shifts of the fibroin in helix or random coil are about 3.9 ppm whereas those of the fibroin in β-sheet are about 5.0 ppm. Furthermore, they found that 1H chemical shift of Ala Cβ in helix conformation was different from that in β-sheet conformation. Fig. 2B shows 1H CRAMPS NMR spectra of the SF samples incubated with Al(III) of various concentrations, which were normalized to 3.9 ppm corresponding to helix or random coil conformers. It was found that the absorption intensity at 5.0 ppm corresponding to β-sheet increased firstly and then decreased as the Al(III) concentration increased over 100 μM.
In addition, 13C chemical shifts and signal intensity are sensitive to the secondary structures of proteins. For SF, Cβ chemical shift of Ala residue is sensitive to the secondary structural change from Silk I (helix and helix-like conformers) to Silk II (β-sheet and β-sheet-like conformers).45 Zhou et al. had calculated Cβ chemical shifts of Ala in GAGAGS sequence under various conformations by density functional theory (DFT),46 and indicated that helix, helix-like, β-sheet and β-sheet-like conformers in SF have chemical shifts of 17 ± 0.5, 15 ± 0.5, 20 ± 0.5, and 22 ± 0.5 ppm, respectively. Fig. 2C is one of the deconvoluted 13C NMR spectra in the range of 10∼28 ppm using Gaussian line-shape to quantitatively analyze the relevant contents of secondary structures of SF. Effect of Al(III) on the Silk II (β-sheet and β-sheet-like conformers) content is shown in Fig. 2D. It was found that the Silk II content increased from 28% to 38% as Al(III) concentrations increased from 0 to 100 μM, while further addition of Al(III) inhibited the formation of Silk II, leading to the Silk II content reduced from 38% to 31%.
3.2 Effect of curcumin on the conformation transition of SF
For SF samples incubated with curcumin of various concentrations, it was found that the signal intensity of SF at 1625 cm−1 corresponding to Silk II somewhat increased as the concentrations of curcumin increased from 0 to 30 μM (Fig. 3A), while it increased dramatically when the concentration of curcumin was higher than 30 μM (Fig. 3A); meanwhile, it was found that the signal intensity of SF at chemical shift of 5.0 ppm has similar change trend, as shown in Fig. 3B.
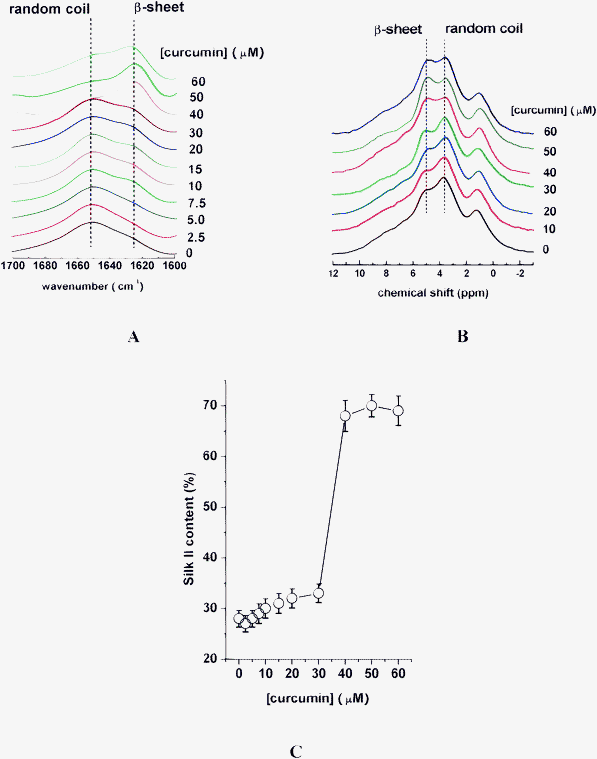 |
| Fig. 3 FT-IR (A), and 1H CRAMPS (B), spectra of silk fibroin in the presence of curcumin of various concentrations. (C) Dependence of Silk II content on curcumin concentration. | |
In addition, based on the deconvolution of 13C NMR spectra, the Silk II content somewhat increased as curcumin concentrations increased from 0 to 30 μM, while it dramatically increased from 30% to the saturation of 68% with further addition of curcumin, as shown in Fig. 3C.
3.3 Effect of curcumin on the Al(III)-induced conformation transition of SF
For SF samples incubated with Al(III) of constant concentration of 80 μM and curcumin of various concentrations, it was found that the signal intensity of SF at 1625 cm−1 gradually decreased as the concentrations of curcumin increased from 0 to 160 μM (Fig. 4A); while, it dramatically increased when the concentration of curcumin was higher than 160 μM (Fig. 4A); meanwhile, it was found that the signal intensity of SF at chemical shift of 5.0 ppm has a similar change trend, as shown in Fig. 4B.
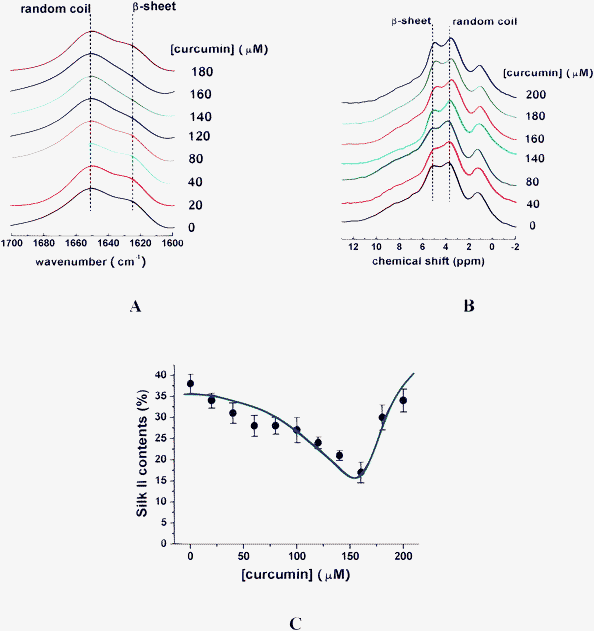 |
| Fig. 4 FT-IR (A), and 1H CRAMPS (B), spectra of silk fibroin in the presence of various concentrations of curcumin and a constant Al(III) concentration of 80 μM. (C) Dependence of Silk II content on curcumin of various concentrations, with Al(III) of constant concentration of 80 μM. | |
In addition, based on the deconvolution of 13C NMR spectra, the Silk II content greatly decreased from 38% to 17% as curcumin concentrations increased from 0 to 160 μM (Fig. 4C). The Silk II content of 17% in the presence of 160 μM curcumin and 80 μM Al(III) in the SF sample was even lower than that in SF alone, indicating the reversion of SF conformation from Silk II to Silk I induced by Al(III)–curcumin complexes; however, the Silk II content dramatically increased from 17% to 34% with further addition of curcumin from 160 μM to 200 μM (Fig. 4C).
3.4 Effect of combined action of Al(III) and curcumin on the conformation transition of SF
In order to reveal the effect of Al(III)–curcumin complexes on SF conformation transition, SF was mixed with Al(III)–curcumin complexes at [Al(III)]/[curcumin] ratios of 1
:
1, 1
:
1.5, 1
:
2 and 1
:
3. Based on the deconvolution of 13C CP/MAS NMR spectra, it was found that Silk II content changed little as curcumin concentrations increased from 0 to 80 μM, when [Al(III)]/[curcumin] ratio was 1
:
1 (Fig. 5A). Intriguingly, Silk II content decreased gradually from 27% to 19% as curcumin concentrations increased from 0 to 120 μM, when [Al(III)]/[curcumin] ratio was 1
:
1.5 (Fig. 5B). Moreover, Silk II content firstly decreased from 27% to 18% as curcumin concentrations increased from 0 to 40 μM, and then increased gradually from 18% to 27% as curcumin concentrations increased from 40 to 160 μM, when [Al(III)]/[curcumin] ratio was 1
:
2 (Fig. 5C). Furthermore, the Silk II content increased gradually from 27% to 33% as curcumin concentrations increased from 0 to 240 μM, when [Al(III)]/[curcumin] ratio was 1
:
3 (Fig. 5D).
![Dependence of Silk II content on [Al(iii)]/[curcumin] ratios of 1 : 1 (A), 1 : 1.5 (B), 1 : 2 (C) and 1 : 3 (D).](/image/article/2012/RA/c2ra21453a/c2ra21453a-f5.gif) |
| Fig. 5 Dependence of Silk II content on [Al(III)]/[curcumin] ratios of 1 : 1 (A), 1 : 1.5 (B), 1 : 2 (C) and 1 : 3 (D). | |
4. Discussion
In combination with the results from ATR-FTIR, solid-state 1H CRAMPS NMR and solid-state 13C CP/MAS NMR, the experiments reported here provide an insight into the role of Al(III), curcumin, particularly Al(III)–curcumin complexes affecting the conformation transition of SF. In the present work, Al(III) of low concentrations could promote the formation of β-sheet conformation in SF, while when the concentration of Al(III) exceeded 100 μM, the excessive Al(III) would lead to the decrease of β-sheet content (Fig. 2). Al(III) can bind –COO– group in Glu and Asp residues and –OH group in Ser and Tyr residues47,48 which are abundant in the SF molecular chain.49 We therefore speculate that Al(III) could interact with oxygen atoms in the amino acids to form octahedral structures, neutralizing the negative charges in SF chain and reducing the electrostatic repulsions which prevent SF chains forming the self-interactions;50 on the other hand, Al(III) might act as a “disulfide linkage” to link two SF chains,20,51 leading to the reduced distance between two SF molecular chains, thus allowing them to approach to each other easily and form inter-H-bonds which promote the conformation transition from random coil to β-sheet. Therefore, finding an approach to inhibit the Al(III)-induced conformation transition is of significance.
Recently, curcumin has been paid particular attention because of its advantages in reduction of the risk of neurodegenerative disorders.52 In the present work, it was found that Silk II content of SF incubated with curcumin was changed a little when the curcumin concentration was lower than 30 μM, while the Silk II content was dramatically increased by curcumin of high concentration (Fig. 3). Curcumin (Fig. 1) is a linear flat molecule like amyloid dyes, such as Congo red and thioflavin-T, capable of binding and inserting itself to protein chains.53–56 Its rigid aromatic frame might block the inter-hydrogen-bonding of proteins, thus inhibiting the formation of β-sheets. However, curcumin at high concentration might precipitate firstly because of its low water solubility and act as a “seed” or “nucleus” for SF aggregation, therefore, curcumin at high concentration would promote the conformation transition of SF into β-sheet, as proven in the SF-based film systems.
Intriguingly, when curcumin was present in the Al(III)/SF samples, the Al(III)-induced conformation transition of SF into β-sheet was inhibited (Fig. 4); moreover, the Al(III)–curcumin mixtures at [Al(III)]/[curcumin] ratio of 1
:
1.5 could even reverse the conformation of SF from β-sheet into random coil (Fig. 4 and Fig. 5). Our previous work demonstrated that curcumin was capable of chelating Al(III) stably via its active enol-H groups to form three types of complexes in molar ratios of 1
:
2, 1
:
1 and 2
:
1.20 Al(III) in Al(III)–curcumin complexes not only chelated curcumin, but also interacted with water molecules, leading to the formed Al(III)–curcumin complexes becoming more hydrophilic.20 Furthermore, interaction of curcumin with Al(III) allowed the curcumin to become more rigid, making its influence on protein more effective.57 The soluble Al(III)–curcumin complexes thus interact with SF chains through diplex functions of hydrophobic curcumin and hydrophilic Al(III), allowing their interaction to be more effective than curcumin alone and leading to the inhibition of β-sheet formation and even the reversion of preformed β-sheet structure to random coil one. In addition, our previous work demonstrated that when the [Al(III)]/[curcumin] ratio is higher than 1
:
1, the formed Al(III)–curcumin complexes are mainly [Al(III)]2[curcumin]2 and [Al(III)]2[curcumin], while when the [Al(III)]/[curcumin] ratios vary from 1
:
1 to 1
:
2, the formed Al(III)–curcumin complexes are mainly [Al(III)][curcumin]2.20 We thus speculate that [Al(III)][curcumin]2 complexes, formed at [Al(III)]/[curcumin] ratio of 1
:
1.5, are more effective to reverse the β-sheet conformation into random coil one, possibly due to one Al(III) ion along with two curcumin molecules inserting into the protein chain and loosing the preformed ordered structure. However, when Al(III) and curcumin were mixed at [Al(III)]/[curcumin] ratio of 1
:
3, the excessive curcumin attenuated the effect of Al(III)–curcumin complexes and somewhat increased the β-sheet content of SF (Fig. 5D).
On the basis of the above analysis, Al(III)–curcumin complexes are called derivatives of curcumin, which are more water soluble than curcumin, and could be considered as an inhibitor of β-sheet formation; meanwhile, Al(III)–curcumin complexes could be used as one of the disintegrators to refold the β-sheet fibrils into random coil conformers, thus potentially developing as a therapeutic agent against neurodegenerative disorders.
Conclusion
This work demonstrates that Al(III)–curcumin complexes could effectively inhibit the β-sheet formation and even reverse the conformation of SF from β-sheet into random coil, which suggested that Al(III)–curcumin complexes could be used as a novel agent for preventing proteins from the formation of amyloid fibrils and even removing the pre-formed amyloid deposits.
Acknowledgements
The work was supported by the Natural Science Foundation of China (Nos. 10475017, 20673022, 21074025), the Science and Technology Innovation Program of Shanghai Municipal Education Commission (No. 11DZ1971802), and the Innovation Program of Shanghai Municipal Education Commission (No. 2012Z102460).
References
- M. B. Pepys, Philos T Roy Soc B, 2001, 356, 203–210 CrossRef CAS.
- S. Y. Tan, M. B. Pepys and P. N. Hawkins, Am J Kidney Dis, 1995, 26, 267–285 CrossRef CAS.
- G. G. Glenner, E. D. Eanes, H. A. Bladen, R. P. Linke and J. D. Termine, J Histochem Cytochem, 1974, 22, 1141–1158 CrossRef CAS.
- M. Sunde, L. C. Serpell, M. Bartlam, P. E. Fraser, M. B. Pepys and C. C. F. Blake, J Mol Biol, 1997, 273, 729–739 CrossRef CAS.
- P. Zatta, R. Lucchini, S. J. van Rensburg and A. Taylor, Brain Res Bull, 2003, 62, 15–28 CrossRef CAS.
- D. T. Dexter, A. Carayon, F. Javoyagid, Y. Agid, F. R. Wells, S. E. Daniel, A. J. Lees, P. Jenner and C. D. Marsden, Brain, 1991, 114, 1953–1975 CrossRef.
- C. Exley, E. House, J. Collingwood, A. Khan, O. Korchazkina and G. Berthon, J. Alzheimers Dis., 2004, 6, 291–301 Search PubMed.
- V. N. Uversky, J. Li and A. L. Fink, J Biol Chem, 2001, 276, 44284–44296 CrossRef CAS.
- J. Bieschke, J. Russ, R. P. Friedrich, D. E. Ehrnhoefer, H. Wobst, K. Neugebauer and E. E. Wanker, P Natl Acad Sci USA, 2010, 107, 7710–7715 CrossRef CAS.
- H. T. Li, D. H. Lin, X. Y. Luo, F. Zhang, L. N. Ji, H. N. Du, G. Q. Song, J. Hu, J. W. Zhou and H. Y. Hu, Febs J., 2005, 272, 3661–3672 CrossRef CAS.
- Y. H. Zhang, T. Jiang, Y. W. Zheng and P. Zhou, Soft Matter, 2012, 8, 5543–5549 RSC.
- G. J. Kelloff, J. A. Crowell, E. T. Hawk, V. E. Steele, R. A. Lubet, C. W. Boone, J. M. Covey, L. A. Doody, G. S. Omenn, P. Greenwald, W. K. Hong, D. R. Parkinson, D. Bagheri, G. T. Baxter, M. Blunden, M. K. Doeltz, K. M. Eisenhauer, K. Johnson, G. G. Knapp, D. G. Longfellow, W. F. Malone, S. G. Nayfield, H. E. Seifried, L. M. Swall and C. C. Sigman, J Cell Biochem, 1996, 72–321 Search PubMed.
- J. E. Galvin, N. Pandey, J. Strider, W. C. Nolan and S. X. Yan, Acta Neuropathol, 2008, 115, 479–489 CrossRef.
- S. Mishra and K. Palanivelu, Ann Indian Acad Neur, 2008, 11, 13–19 CrossRef.
- T. Jiang, X. L. Zhi, Y. H. Zhang, L. F. Pan and P. Zhou, Bba-Mol Basis Dis, 2012, 1822, 1207–1215 CrossRef CAS.
- K. I. Priyadarsini, A. Barik, B. Mishra, L. Shen, H. Mohan, R. M. Kadam, S. Dutta and H. Y. Zhang, Free Radical Bio Med, 2005, 39, 811–822 CrossRef.
- X. Z. Zhao, T. Jiang, L. Wang, H. Yang, S. Zhang and P. Zhou, J Mol Struct, 2010, 984, 316–325 CrossRef CAS.
- D. H. Xu, X. T. Mei, S. K. Xu, Y. P. Zheng and S. B. Xu, Pharmacol Biochem Be, 2011, 99, 66–74 CrossRef.
- M. B. H. Youdim, S. Mandel, T. Amit and O. Bar-Am, Prog Neurobiol, 2007, 82, 348–360 CrossRef.
- T. Jiang, L. Wang, S. Zhang, P. C. Sun, C. F. Ding, Y. Q. Chu and Z. Ping, J Mol Struct, 2011, 1004, 163–173 CrossRef CAS.
- O. Vajragupta, P. Boonchoong, H. Watanabe, M. Tohda, N. Kummasud and Y. Sumanont, Free Radical Bio Med, 2003, 35, 1632–1644 CrossRef CAS.
- B. B. Aggarwal, P. Anand, A. B. Kunnumakkara and R. A. Newman, Mol Pharmaceut, 2007, 4, 807–818 CrossRef.
- B. B. Aggarwal, P. Anand, S. G. Thomas, A. B. Kunnumakkara, C. Sundaram, K. B. Harikumar, B. Sung, S. T. Tharakan, K. Misra, I. K. Priyadarsini and K. N. Rajasekharan, Biochem Pharmacol, 2008, 76, 1590–1611 CrossRef.
- C. Mohanty and S. K. Sahoo, Biomaterials, 2010, 31, 6597–6611 CrossRef CAS.
- A. Lammel, M. Schwab, M. Hofer, G. Winter and T. Scheibel, Biomaterials, 2011, 32, 2233–2240 CrossRef CAS.
- A. S. Lammel, X. Hu, S. H. Park, D. L. Kaplan and T. R. Scheibel, Biomaterials, 2010, 31, 4583–4591 CrossRef CAS.
- M. Heim, D. Keerl and T. Scheibel, Angewandte Chemie-International Edition, 2009, 48, 3584–3596 CrossRef CAS.
- K. Tanaka, N. Kajiyama, K. Ishikura, S. Waga, A. Kikuchi, K. Ohtomo, T. Takagi and S. Mizuno, Bba-Protein Struct M, 1999, 1432, 92–103 CrossRef CAS.
- P. Couble, M. Chevillard, A. Moine, P. Ravelchapuis and J. C. Prudhomme, Nucleic Acids Res, 1985, 13, 1801–1814 CrossRef CAS.
- Y. Shen, M. A. Johnson and D. C. Martin, Macromolecules, 1998, 31, 8857–8864 CrossRef CAS.
- C. Z. Zhou, F. Confalonieri, N. Medina, Y. Zivanovic, C. Esnault, T. Yang, M. Jacquet, J. Janin, M. Duguet, R. Perasso and Z. G. Li, Nucleic Acids Res, 2000, 28, 2413–2419 CrossRef CAS.
- G. Y. Li, P. Zhou, Z. Z. Shao, X. Xie, X. Chen, H. H. Wang, L. J. Chunyu and T. Y. Yu, Eur J Biochem, 2001, 268, 6600–6606 CrossRef CAS.
- Y. R. Chen, C. L. Ni, H. P. Shi, H. M. Yu and Y. C. Chang, Faseb J, 2011, 25, 1390–1401 CrossRef.
- H. N. Du, L. Tang, X. Y. Luo, H. T. Li, J. Hu, J. W. Zhou and H. Y. Hu, Biochemistry-Us, 2003, 42, 8870–8878 CrossRef CAS.
- A. Martel, M. Burghammer, R. J. Davies, E. D. Cola, C. Vendrely and C. Riekel, J Am Chem Soc, 2008, 130, 17070–17074 CrossRef CAS.
- S. A. Fossey, G. Nemethy, K. D. Gibson and H. A. Scheraga, Biopolymers, 1991, 31, 1529–1541 CrossRef CAS.
- X. H. Zong, P. Zhou, Z. Z. Shao, S. M. Chen, X. Chen, B. W. Hu, F. Deng and W. H. Yao, Biochemistry-Us, 2004, 43, 11932–11941 CrossRef CAS.
- P. Zhou, X. Xie, F. Deng, Z. Ping, X. Xun and D. Feng, Biochemistry-Us, 2004, 43, 11302–11311 CrossRef CAS.
- L. Zhou, X. Chen, Z. Z. Shao, Y. F. Huang and D. P. Knight, J Phys Chem B, 2005, 109, 16937–16945 CrossRef CAS.
- Y. H. Yang, Z. Z. Shao, X. Chen and P. Zhou, Biomacromolecules, 2004, 5, 773–779 CrossRef CAS.
- B. Li, L. Xu, Q. Wu, T. Chen, P. Sun, Q. Jin, D. Ding, X. Wang, G. Xue and A. C. Shi, Macromolecules, 2007, 40, 5776–5786 CrossRef CAS.
- D. M. Byler and H. Susi, Biopolymers, 1986, 25, 469–487 CrossRef CAS.
- A. Shoji, H. Kimura, T. Ozaki, H. Sugisawa and K. Deguchi, J Am Chem Soc, 1996, 118, 7604–7607 CrossRef CAS.
- H. Kimura, S. Kishi, A. Shoji, H. Sugisawa and K. Deguchi, Macromolecules, 2000, 33, 9682–9687 CrossRef CAS.
- A. E. Terry, D. P. Knight, D. Porter and F. Vollrath, Biomacromolecules, 2004, 5, 768–772 CrossRef CAS.
- P. Zhou, G. Y. Li, Z. Z. Shao, X. Y. Pan and T. Y. Yu, J Phys Chem B, 2001, 105, 12469–12476 CrossRef CAS.
- G. D. Fasman, Coordin Chem Rev, 1996, 149, 125–165 CAS.
- T. Miura, K. Suzuki, N. Kohata and H. Takeuchi, Biochemistry-Us, 2000, 39, 7024–7031 CrossRef CAS.
- ExPASy, P05790, E. P. A. S.; P07856.
- P. Zatta, F. Ricchelli, D. Drago, B. Filippi and G. Tognon, Cell Mol Life Sci, 2005, 62, 1724–1733 CrossRef.
- J. W. Akitt and W. Gessner, J Chem Soc Dalton, 1984, 147–148 RSC.
- F. M. Tortia, H. Hatcher, R. Planalp, J. Cho and S. V. Torti, Cell Mol Life Sci, 2008, 65, 1631–1652 CrossRef.
- F. S. Yang, G. P. Lim, A. N. Begum, O. J. Ubeda, M. R. Simmons, S. S. Ambegaokar, P. P. Chen, R. Kayed, C. G. Glabe, S. A. Frautschy and G. M. Cole, J Biol Chem, 2005, 280, 5892–5901 CrossRef CAS.
- M. R. H. Krebs, E. H. C. Bromley and A. M. Donald, J Struct Biol, 2005, 149, 30–37 CrossRef CAS.
- W. E. Klunk, J. W. Pettegrew and D. J. Abraham, J Histochem Cytochem, 1989, 37, 1273–1281 CrossRef CAS.
- G. Romhanyi, Virchows Arch A, 1971, 354, 209 CrossRef CAS.
- A. A. Reinke and J. E. Gestwicki, Chem Biol Drug Des, 2007, 70, 206–215 CAS.
Footnotes |
† The authors declare that there are no conflicts of interest. |
‡ Conceived the proposal and designed the procedures: PZ, TJ. Performed the experiments: TJ, GRZ, QMD, PCS. Analyzed the data: TJ, GRZ, QMD, PCS. Wrote the paper: PZ, TJ, YHZ. |
|
This journal is © The Royal Society of Chemistry 2012 |
Click here to see how this site uses Cookies. View our privacy policy here.