DOI:
10.1039/C2RA21888G
(Paper)
RSC Adv., 2012,
2, 12909-12914
Enzymatically triggered multifunctional delivery system based on hyaluronic acid micelles†
Received
22nd August 2012
, Accepted 18th October 2012
First published on 6th November 2012
Abstract
Tumor targetability and stimuli responsivity of drug delivery systems (DDS) are key factors in cancer therapy. Implementation of multifunctional DDS can afford targetability and responsivity at the same time. Herein, cholesterol molecules (Ch) were coupled to hyaluronic acid (HA) backbones to afford amphiphilic conjugates that can self-assemble into stable micelles. Doxorubicin (DOX), an anticancer drug, and superparamagnetic iron oxide (SPIO) nanoparticles (NPs), magnetic resonance imaging (MRI) contrast agents, were encapsulated by Ch–HA micelles and were selectively released in the presence of hyaluronidase (Hyals) enzyme. Cytotoxicity and cell uptake studies were done using three cancer cell lines (HeLa, HepG2 and MCF7) and one normal cell line (WI38). Higher Ch–HA micelles uptake was seen in cancer cells versus normal cells. Consequently, DOX release was elevated in cancer cells causing higher cytotoxicity and enhanced cell death.
Introduction
Polymeric drug delivery systems (DDSs) can deliver anti-cancer drugs into tumor tissues efficiently by the enhanced permeation retention (EPR) effect.1–3 As a classical DDS, polymeric micelles can enhance the solubility of hydrophobic drugs in water. Their polymeric layers provide drugs with longer blood circulation times. A conventional micellar DDS consists of degradable polymers (polyesters, polycarbonates) which can be broken down inside human body from days to weeks. Thus, a degradable polymeric micellar DDS can be designed and used for long term release of encapsulated drugs.4,5 However, this class of DDS delivers drugs nonselectively and only through the EPR effect, which leads to lower therapeutical efficacy and side effects.6
Hyaluronic acid (HA), one of the most promising biomaterials, is attracting much attention due to its nature as a natural polysaccharide and its relatively fast biodegradable half-life.7–9 HA can bind to CD44, which is a receptor that is overexpressed on various tumor cells.8,10–14 Thus HA has higher biodistribution in tumor tissues versus normal ones. In the presence of hyaluronidase (Hyals) which is also overexpressed in cancer cells,15,16 HA can degrade into low molecular weight fragments. Therefore, HA degrades more rapidly in tumor tissues, and increased amounts of low molecular weight HA fragments are found in tumor tissues.12–14 This has great significance on the early diagnosis of cancer. Moreover, the conjugates can be easily prepared because HA has several functional groups (–COOH, –OH) for chemical modification.17,18 Several drug-conjugated HA examples have already been developed as macromolecular prodrugs.14,19–21
Superparamagnetic iron oxide (SPIO) nanoparticles (NPs) were proved to have promising biomedical applications because of their biologically friendly properties and high chemical stability. They are used as contrast agents for magnetic resonance imaging (MRI) in clinical diagnostics.22,23 Thus, a multifunctional tumor-targeted drug delivery system (DDS), combining anti-cancer drug and MRI contrast agent, can bring synchronous diagnosis and therapy into reality. Meanwhile, biocompatible materials used as DDS corona can improve the biocompatibility of SPIO.24–26
In this study, we developed new cholesterol-conjugated HA micelles (Ch–HA) as active and multifunctional DDS. These amphiphilic conjugates can self-assemble into stable micelles with the encapsulation of doxorubicin (DOX), an anticancer drug, and SPIO NPs as the MRI contrast agents. The magnetic properties of DOX/SPIO-loaded Ch–HA (DSCH) micelles are evaluated and cellular uptake of DSCH into cancer (HeLa, HepG2, and MCF7) and normal (WI38) cell lines is characterized.
Experimental section
Materials
Sodium hyaluronate (Sigma-Aldrich) was used after dialysis against distilled water, followed by lyophilization before using. Cholesterol (Ch), tetraethylene glycol (TEG), N-hydroxysuccinimide (NHS), 1-ethyl-3-(3-dimethylaminopropyl)carbodiimide (EDC), hyaluronidase (Hyal), phosphate buffered solutions (PBS, pH 7.4) and all the other organic solvents were purchased from Sigma-Aldrich and used without any further purification. Doxorubicin hydrochloride (DOX·HCl) was purchased from Sigma-Aldrich and treated with triethylamine to precipitate from water. It was then dissolved in DMF before use. The human cervical tumor cell line (HeLa), liver cancer cell line (HepG2), breast tumor cell line (MCF7) and human lung fibroblast cell line (WI38) were purchased from ATCC (USA). Eagle's MEM medium (EMEM) and fetal bovine serum (FBS) penicillin-streptomycin and 4,6-diamino-2-phenylindole (DAPI) were purchased from Invitrogen (USA). Insulin was obtained from Sigma-Aldrich.
Excess p-toluenesulfonyl chloride (3 g, 15.8 mmol) was added to a pyridine (15 mL) solution of cholesterol (5 g, 12.9 mmol) and stirred overnight at room temperature (Scheme 1). After adding water (25 mL), the mixture was extracted three times with diethyl ether. The combined organic layers were dried over anhydrous sodium sulfate, and concentrated in vacuo to give a white powder. Finally, the crude cholesteryl tosylate was recrystallized in petroleum ether.
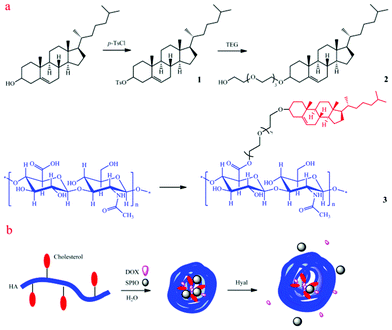 |
| Scheme 1 Schematic illustration of (a) synthesis of cholesterol-conjugated hyaluronic acid (ch-HA) and (b) the formation of DOX/SPIO-loaded ch-HA micelles and enzyme-triggered release. Dox: doxorubicin; SPIO: superparamagnetic iron oxide; Hyal: hyaluronidase. | |
Preparation of tetraethylene glycol monocholeteryl ether (Ch–TEG, 2)
Tetraethylene glycol (20 mL) was added to a dioxane (50 mL) solution of cholesteryl tosylate (3 g, 5.6 mmol) (Scheme 1). The mixture was refluxed for 5 h under nitrogen. Then it was poured into water and extracted three times with ethyl acetate. The combined organic layers were dried over anhydrous sodium sulfate, and concentrated in vacuo. Yellow oil was obtained and purified by column chromatography (ethyl acetate/petroleum ether 2
:
8). The expected compound, Ch–TEG was obtained as a white oily solid.
Preparation of cholesterol-conjugated hyaluronic acid (Ch–HA, 3)
Lyophilized hyaluronic acid (HA, 40 mg) was dissolved in water containing EDC and NHS. The mixture was stirred for 24 h after adding a DMF solution of Ch–TEG (Scheme 1). The molar ratios of Ch–TEG to carboxyl groups of HA are 25% (Ch–HA1), 50% (Ch–HA2) and 75% (Ch–HA3), respectively. The crude product was dialyzed against water/methanol (1/3, v/v) for 1 day and water for 3 days. After lypholization, Ch–HA was obtained (Fig. S1, ESI†). In this work, Ch–HA2 was chosen for further characterizations without specification.
Preparation of superparamagnetic iron oxide (SPIO) nanoparticles (NPs)
SPIO NPs were synthesized according to the literature27 with few modifications. First, 6 nm SPIO NPs seeds were synthesized. Fe(acac)3 (2 mmol), 1,2-hexadecanediol (10 mmol), oleic acid (6 mmol), oleylamine (6 mmol), and benzyl ether (20 mL) were mixed and magnetically stirred under a flow of nitrogen. The mixture was heated at 200 °C for 2 h, and then heated at 300 °C and reflux for 1 h. The mixture was cooled to room temperature. Ethanol (40 mL) was added to the mixture and particles were precipitated. They were separated by centrifugation and then re-dissolved in hexane in the presence of oleic acid (0.05 mL) and oleylamine (0.05 mL). Centrifugation (6000 rpm, 10 min) was applied to remove any undispersed residue. Fe3O4 nanoparticles were then precipitated with ethanol, centrifuged (6000 rpm, 10 min) to remove the solvent, and redispersed into hexane.
Second, 8 nm SPIO NPs were synthesized via 6 nm SPIO NPs seeds. Fe(acac)3 (2 mmol), 1,2-hexadecanediol (10 mmol), benzyl ether (20 mL), oleic acid (2 mmol), and oleylamine (2 mmol) were mixed and magnetically stirred under a flow of N2. A 84 mg sample of 6 nm Fe3O4 nanoparticles dispersed in hexane (4 mL) was added. The mixture was first heated at 100 °C for 30 min to remove hexane, then at 200 °C for 1 h. The mixture was further heated at 300 °C and reflux for 30 min. The mixture was cooled to room temperature. Following the same procedures described in the synthesis of 6 nm particles, a hexane dispersion of 8 nm Fe3O4 nanoparticles was produced.
Preparation and characterization of doxorubicin (DOX) and SPIO-loaded Ch–HA micelles (DSCH micelles)
DOX/SPIO-loaded Ch–HA (DSCH) micelles were prepared by the dialysis method (Scheme 1). Ch–HA were dissolved in PBS buffer (pH 7.4), and DOX was dissolved in DMF. SPIO NPs hexane solution was dried by nitrogen flow and re-dispersed in THF. The DMF solution of DOX and THF solution of SPIO NPs were added into Ch–HA aqueous solution drop by drop under sonication. The resulting mixture was dialyzed against water for three days to remove unloaded DOX, SPIO NPs, DMF and THF. The micelles were biodegraded by hyaluronidase and then centrifuged to remove precipitant SPIO aggregates. The loading contents for DOX were determined using UV-vis spectrometer at 485 nm.
Characterization of Ch–HA micelles and DSCH micelles
The critical micelle concentration (CMC) of Ch–HA2 was evaluated by fluorescence spectroscopy of pyrene probe. Briefly, 1 mL of 6 × 10−6 M pyrene solution in acetone was added into a series of 10 mL volumetric flasks and then acetone was evaporated by nitrogen flow. 10 mL of different concentrations of Ch–HA2 solutions were added and stirred over night. Pyrene fluorescence spectra were obtained by a fluorescence spectrophotometer. The CMC was estimated as the cross point when extrapolating the intensity ratio I338/I333.
Ch–HA micelles and DSCH micelles were observed by FEI T12 TEM. Ch–HA samples were negatively stained by uranyl acetate.
Magnetic properties
Magnetic properties of SPIO NPs in hexane and in DSCH micelles were examined by commercial Magnetic Property Measurement System (MPMS® SQUID VSM), utilizing Superconducting Quantum Interference Device (SQUID) technology.
Cell viabilities of HeLa, HepG2, MCF7 and WI38 treated with DSCH micelles
The cytotoxicity of DSCH micelles were evaluated using the MTT assay. Four different cells (HeLa, HepG2, MCF7, WI38) were seeded at a density of 5 × 103 cells per well in 96-well flat bottom plates and incubated for 12 h. Cells were washed by DPBS buffer and incubated in the culture media with DSCH micelles for 24 h at 37 °C. Cell viability was evaluated by the MTT colorimetric procedure.
Enzyme-triggered DOX release from DSCH micelles
The concentration of released DOX from DSCH micelles were calculated by UV-vis spectrometer. Briefly, 5 mL (c = 1 mg mL−1) DSCH micelles were loaded with 0 unit or 1500 units hyaluronidase in dialysis bags (cut-off MW = 2000). Then the dialysis bags were immersed in 10 mL PBS buffer. The absorbance of PBS buffer was tested at same time intervals.
In vitro DOX release
HeLa, HepG2 and WI38 cells were cultured in EMEM medium containing 10% FBS and penicillin-streptomycin at 37 °C in a humidified 5% CO2 atmosphere. MCF7 cells were cultured in EMEM medium containing 10% FBS, 0.5% insulin and 0.1% penicillin-streptomycin at 37 °C in a humidified 5% CO2 atmosphere. After cell attachment, the medium was replaced by medium containing DSCH micelles, followed by incubation for 24 h. The cells were then washed twice with DPBS and fixed with a 4% paraformaldehyde solution. For nuclear staining, the cells were incubated with DAPI for 10 min at room temperature, following 3 washes in PBS. Then the cells were observed under confocal laser scanning microscopy (CLSM, Zeiss) and intracellular release and trafficking of DOX were examined.
Localization and cellular uptake of DSCH micelles
HeLa, HepG2 and WI38 cells were cultured in EMEM medium containing 10% FBS and 0.1% penicillin-streptomycin at 37 °C in a humidified 5% CO2 atmosphere. MCF7 cells were cultured in EMEM medium containing 10% FBS, 0.5% insulin and 0.1% penicillin-streptomycin at 37 °C in a humidified 5% CO2 atmosphere. After cell attachment, the medium was replaced by medium containing DSCH micelles, followed by incubation for 24 h.
The cells cultured on cover slides were washed twice with DPBS and fixed with a 2.5% glutaraldehyde for 1 h and washed 3 times with DPBS. Samples were then dehydrated using a graded ethanol series, and then dried by critical CO2. The cover slides were coated by Au prior to SEM analysis. The cells were then washed twice with DPBS and fixed with a 2.5% glutaraldehyde for 1 h and washed 3 times with DPBS. Samples were then dehydrated using a graded ethanol series, then replaced by acetone followed by resin series. The cells were polymerized at 60 °C over night, cut into ultrathin sections, loaded onto copper TEM grids, and stained with uranyl acetate and lead citrate prior to TEM analysis.
Results and discussion
Synthesis and characterization of the DSCH micelles
The fluorescence emission spectra of pyrene were used to determine the micellization behaviors of the Ch–HA in aqueous solution. It is well known that the intensity ratio of the first to the third vibration bands (I1/I3) in the emission spectra of pyrene is rather sensitive to the polarity of the external medium. If the micelles are formed in an aqueous solution, the pyrene molecules will transfer from a hydrophilic microenvironment to a hydrophobic microenvironment, which causes the significant decrease of I1/I3.
Fig. 1a shows the fluorescence spectra of pyrene in Ch–HA solutions of different concentrations. Fig. 1b shows the change of I1/I3 as a function of the concentration at 25 °C in the aqueous solution of Ch–HA. The Ch–HA chains are flexible and stretch at low concentrations and the value of I1/I3 stays higher than 1.7. With increasing concentration, Ch–HA self-assembled into micelles and I1/I3 decreases rapidly. The critical micellar concentration (CMC) can be calculated as 2.2 × 10−3 mg mL−1. The relatively low CMC means that the structure of the micelles can stay stable after injection of micelles into a human body; even though they are diluted by blood. Therefore, low CMC of Ch–HA suggests that it can form stable micelles in water, which is important to the encapsulation of drugs.28
 |
| Fig. 1 (a) Fluorescence of pyrene at different concentration of Ch–HA micelles. (b) Critical micellar concentration (CMC) of Ch–HA. | |
TEM was used to characterize the morphology of Ch–HA micelles (Fig. 2a), SPIO NPs (Fig. 2b) and DSCH micelles (Fig. 2c and 2d). As shown in Fig. 2, Ch–HA can form sphere-like nanoparticles with diameters from dozens to hundreds nanometers, which is ascribed to the polydisperse molecular weight of HA. DOX and SPIO NPs were encapsulated into Ch–HA micelles by dialysis method as described before. The encapsulated DOX concentrations are 0.17 mg mL−1 (1 mg mL−1 Ch–HA1), 0.15 mg mL−1 (1 mg mL−1 Ch–HA2), and 0.13 mg mL−1 (1 mg mL−1 Ch–HA3). Comparing Fig. 2b with 2c and 2d, SPIO NPs can be found in DSCH micelles.
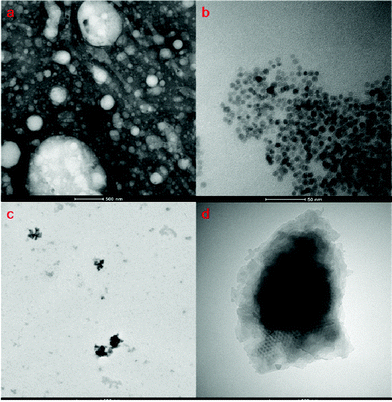 |
| Fig. 2 TEM images of (a) bare Ch–HA micelles (negative stained), (b) SPIO nanoparticles, and (c) DSCH micelles. (d) Amplified TEM image of DSCH micelle. | |
The magnetic properties were studied by a commercial Quantum Design superconducting quantum interference device (SQUID) magnetometer. The field-dependent magnetization for the SPIO NPs in hexane at 5 K and 150 K is shown in Fig. 3a. The field-dependent magnetization for the SPIO NPs encapsulated in lyophilized Ch–HA micelles at 5 K and 300 K is shown in Fig. 3c. When the field is increased, both magnetizations increase linearly and get saturated around 1 T, which indicates a very typical ferromagnetic behavior.
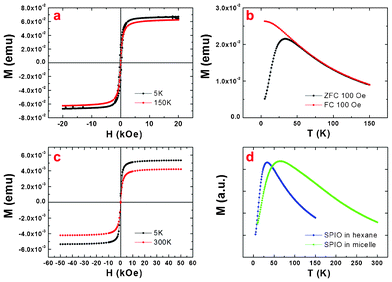 |
| Fig. 3 Magnetic properties: (a) field-dependent magnetization for the SPIO nanoparticles in hexane at 5 K and 150 K, (b) the temperature-dependent magnetizations for the SPIO in hexane under zero-field-cooled (ZFC) and field-cooled (FC) processes, (c) field-dependent magnetization for DOX/SPIO-loaded Ch–HA micelles at 5 K and 300 K, (d) ZFC measurements for the SPIO nanoparticles and DOX/SPIO-loaded Ch–HA micelles. | |
As shown in Fig. 3b, the temperature dependent magnetizations for the SPIO nanoparticles in hexane have been recorded under zero-field-cooled (ZFC) and field-cooled (FC) processes. The peak temperature of the ZFC curve is called blocking temperature TB. It can be estimated by an equation
where
K is the anisotropy constant,
V is the volume of
nanoparticles,
KB is the Boltzmann constant. As a result, a narrow size distribution of
nanoparticles would result in a narrow peak in the ZFC curve. The narrow peak in the ZFC curve in
Fig. 3b shows us a typical magnetic behavior of magnetic
nanoparticles.
The ZFC measurements for the SPIO in hexane and in lyophilized micelles respectively are shown in Fig. 3d. Here we can find that the ZFC peak of the SPIO NPs in hexane is much narrower than that of SPIO NPs in lyophilized micelles, which indicates a broadened energy barrier distribution in the latter. A broadened energy barrier (KV) distribution will give a broader distribution of the blocking temperature; consequently, the peak of the ZFC will become broader. This broadened energy barrier distribution may be induced by the agglomerations of the nanoparticles. Detailed magnetic properties of all Ch–HA micelles are shown in Fig. S2, ESI†.
Enzyme-triggered release of DOX from DSCH micelles
To investigate the enzyme-triggered release of DOX from DSCH micelles, they were loaded with 0 units or 1500 units of hyaluronidase (Hyal) in dialysis bags immersed in PBS buffer (Fig. 4). The absorbance of PBS buffer was tested at the same time intervals. Most anticancer drugs, e.g. DOX, are difficult to be released because of their insolubility in water. Therefore, DSCH micelles are stable for a long time without any Hyal. However, after adding 1500 units of Hyal, DOX can be released constantly. This indicates that a certain degree of biodegradation of HA can produce some defects in DSCH micelles which allows the constant release of DOX from DSCH micelles. Meanwhile, some of the SPIO NPs can be delivered as well.
 |
| Fig. 4 Enzyme-trigged release of DOX from DSCH micelles (in the presence and absence of Hyal). | |
In vitro cytotoxicity of DSCH micelles to cancer cells (HeLa, HepG2, and MCF7) and normal fibroblast cells (WI38) were evaluated using MTT assay (Fig. 5). The cytotoxicity of DSCH micelles to cancer cells is higher than that of free DOX. However, the cytotoxicity of DSCH micelles to normal cell shows an opposite result. This difference can be explained by enhanced uptake of DSCH micelles into cancer cells via CD44-mediated endocytosis. Moreover, a higher level of Hyal in cancer cells can accelerate the biodegradation of HA and release of DOX, leading to higher cytotoxicity. Detailed cytotoxicity of different DSCH micelles is shown in Fig. S3, ESI†.
 |
| Fig. 5 Cell viabilities of HeLa, HepG2, MCF7 and WI38 after incubation with DSCH micelles after 24 h. | |
The release behaviour of DOX from DSCH micelles was observed using confocal laser scanning microscopy (CLSM). After DSCH micelles were incubated with HeLa, HepG2, MCF7 and WI38 for 5, 15, 30, 60 min, time-dependent red fluorescence can be found in the perinuclear area of all the cells. This is ascribed to the internalization of the DSCH micelles into the cytoplasm of all the cells in a short time (Fig. S4, ESI†). However, after the incubation of DSCH micelles with cells for 24 h, strong red fluorescent signals of DOX in the nucleolus of HeLa, HepG2 and MCF7 can be observed. This means, in cancer cells, DOX can be released faster because of active endocytosis or higher level of Hyal. As shown in Fig. 6, the nucleoli of cancer cells were stained by DAPI, which is blue (the first column). The red fluorescent signal of DOX in cells can be observed in the second column. Cytotoxicity and CLSM results indicate that encapsulated DOX of DSCH micelles can be released rapidly when exposed to Hyal. Therefore, DSCH micelles can achieve intracellular release of DOX, enhance the therapeutic efficiency and provide selectivity against cancer cells.
 |
| Fig. 6 Confocal laser scan microscopy images of (a) HeLa, (b) HepG2, (c) MCF7, (d) WI38. From left to right, figures are DAPI-stained nucleus, DOX, bright field of cells, merged images. | |
Cellular internalization of DSCH micelles
A detailed localization of the DSCH micelles was obtained by SEM and TEM measurements. Fig. 7 shows the morphologies of HeLa, HepG2, MCF7, and WI38 incubated with DSCH micelles after 24 h. Due to the high viscosity and gelation of hyaluronic acid in water, DSCH micelles can maintain a sphere-like structure after critical CO2 treatment. The adherence of DSCH micelles onto cells can be observed clearly. A higher amount of adherence of DSCH micelles on HeLa, HepG2 and MCF7 than that on WI38 can be found, which is explained by the overexpression of CD44 leading to stronger interaction between them and DSCH micelles. This kind of adherence makes the following endocytosis possible.
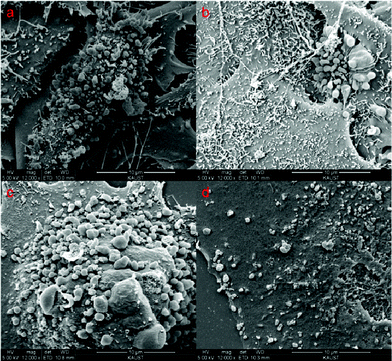 |
| Fig. 7 SEM images of (a) HeLa, (b) HepG2, (c) MCF7 and (d) WI38 cells after treatment of DSCH micelles. | |
Comparing the TEM images of different cells, DSCH micelles can be clearly located (Fig. 8). But in cancer cells, i.e. HeLa, HepG2 and MCF7, the resembled endosomes consisting of DSCH micelles can be found, in which some hyaluronic acid in endosomes is biodegraded to various degrees. These results provide strong evidence that DSCH micelles can be internalized into cancer cells. In contrast, the micelles in WI38 cells show low uptake ability as there were few micelles in WI38 without clear endosome structure. This shows that the cellular uptake of DSCH micelles in cancer cells, i.e., HeLa, HepG2 and MCF7, is mainly through endocytosis because of the formation of endosomes. However, in WI38 the internalization of DSCH micelles can be ascribed to passive penetration or membrane fusion.28
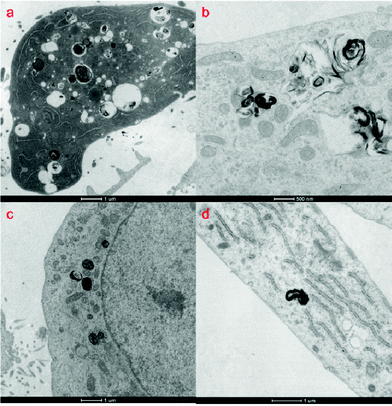 |
| Fig. 8 TEM images of (a) HeLa (b) HepG2 (c) MCF7 and (d) WI38 after treatment of DSCH micelles. | |
Conclusion
A multifunctional delivery system based on cholesterol-conjugated hyaluronic acid micelles was prepared successfully in this work. Doxorubicin and superparamagnetic nanoparticles can be delivered in the presence of hyaluronidase. Higher cytotoxicity and uptake can be observed in cancer cells because of the targetability and selectivity to the overexpressed CD44 receptors and Hyals enzyme. Moreover due to its stability and interesting magnetic properties, applications of this system in hyperthermia therapy and cell separation are now in progress.
References
- K. Kataoka, A. Harada and Y. Nagasaki, Adv. Drug Delivery Rev., 2001, 47, 113–131 CrossRef CAS.
- M. Sokolsky-Papkov, K. Agashi, A. Olaye, K. Shakesheff and A. J. Domb, Adv. Drug Delivery Rev., 2007, 59, 187–206 CrossRef CAS.
- V. P. Torchilin, Adv. Drug Delivery Rev., 2006, 58, 1532–1555 CrossRef CAS.
- Y. Ikada and H. Tsuji, Macromol. Rapid Commun., 2000, 21, 117–132 CrossRef CAS.
- X. Shuai, H. Ai, N. Nasongkla, S. Kim and J. Gao, J. Controlled Release, 2004, 98, 415–426 CrossRef CAS.
- J. Fang, H. Nakamura and H. Maeda, Adv. Drug Delivery Rev., 2011, 63, 136–151 CrossRef CAS.
- L. Lapcik, L. Lapcik, S. De Smedt, J. Demeester and P. Chabrecek, Chem. Rev., 1998, 98, 2663–2684 CrossRef CAS.
- B. P. Toole, Nat. Rev. Cancer, 2004, 4, 528–539 CrossRef CAS.
- M. Morra, Biomacromolecules, 2005, 6, 1205–1223 CrossRef CAS.
- M. Gotte and G. W. Yip, Cancer Res., 2006, 66, 10233–10237 CrossRef.
- S. Jaracz, J. Chen, L. V. Kuznetsova and L. Ojima, Bioorg. Med. Chem., 2005, 13, 5043–5054 CrossRef CAS.
- K. Y. Choi, K. H. Min, J. H. Na, K. Choi, K. Kim, J. H. Park, I. C. Kwon and S. Y. Jeong, J. Mater. Chem., 2009, 19, 4102–4107 RSC.
- K. Y. Choi, H. Chung, K. H. Min, H. Y. Yoon, K. Kim, J. H. Park, I. C. Kwon and S. Y. Jeong, Biomaterials, 2010, 31, 106–114 CrossRef CAS.
- K. Y. Choi, K. H. Min, H. Y. Yoon, K. Kim, J. H. Park, I. C. Kwon, K. Choi and S. Y. Jeong, Biomaterials, 2011, 32, 1880–1889 CrossRef CAS.
- R. Stern and M. J. Jedrzejas, Chem. Rev., 2006, 106, 818–839 CrossRef CAS.
- R. Stern, Semin. Cancer Biol., 2008, 18, 275–280 CrossRef CAS.
- E. J. Oh, K. Park, K. S. Kim, J. Kim, J.-A. Yang, J.-H. Kong, M. Y. Lee, A. S. Hoffman and S. K. Hahn, J. Controlled Release, 2010, 141, 2–12 CrossRef CAS.
- X. Xu, A. K. Jha, D. A. Harrington, M. C. Farach-Carson and X. Jia, Soft Matter, 2012, 8, 3280–3294 RSC.
- K. Y. Choi, H. Y. Yoon, J.-H. Kim, S. M. Bae, R.-W. Park, Y. M. Kang, I.-S. Kim, I. C. Kwon, K. Choi, S. Y. Jeong, K. Kim and J. H. Park, ACS Nano, 2011, 5, 8591–8599 CrossRef CAS.
- J. Li, M. Huo, J. Wang, J. Zhou, J. M. Mohammad, Y. Zhang, Q. Zhu, A. Y. Waddad and Q. Zhang, Biomaterials, 2012, 33, 2310–2320 CrossRef CAS.
- X. Yang, S. Kootala, J. Hilborn and D. A. Ossipov, Soft Matter, 2011, 7, 7517–7525 RSC.
- U. I. Tromsdorf, O. T. Bruns, S. C. Salmen, U. Beisiegel and H. Weller, Nano Lett., 2009, 9, 4434–4440 CrossRef CAS.
- J. F. Lutz, S. Stiller, A. Hoth, L. Kaufner, U. Pison and R. Cartier, Biomacromolecules, 2006, 7, 3132–3138 CrossRef CAS.
- O. T. Bruns, H. Ittrich, K. Peldschus, M. G. Kaul, U. I. Tromsdorf, J. Lauterwasser, M. S. Nikolic, B. Mollwitz, M. Merkell, N. C. Bigall, S. Sapra, R. Reimer, H. Hohenberg, H. Weller, A. Eychmuller, G. Adam, U. Beisiegel and J. Heeren, Nat. Nanotechnol., 2009, 4, 193–201 CrossRef CAS.
- B. Basly, D. Felder-Flesch, P. Perriat, C. Billotey, J. Taleb, G. Pourroy and S. Begin-Colin, Chem. Commun., 2010, 46, 985–987 RSC.
- L. Xiao, J. Li, D. F. Brougham, E. K. Fox, N. Feliu, A. Bushmelev, A. Schmidt, N. Mertens, F. Kiessling, M. Valldor, B. Fadeel and S. Mathur, ACS Nano, 2011, 5, 6315–6324 CrossRef CAS.
- S. H. Sun, H. Zeng, D. B. Robinson, S. Raoux, P. M. Rice, S. X. Wang and G. X. Li, J. Am. Chem. Soc., 2004, 126, 273–279 CrossRef CAS.
- S. Tu, Y.-W. Chen, Y.-B. Qiu, K. Zhu and X.-L. Luo, Macromol. Biosci., 2011, 11, 1416–1425 CrossRef CAS.
Footnote |
† Electronic supplementary information (ESI) available: Details of NMR results, magnetic properties, cytotoxity, and time series CLSM results. See DOI: 10.1039/c2ra21888g |
|
This journal is © The Royal Society of Chemistry 2012 |
Click here to see how this site uses Cookies. View our privacy policy here.