DOI:
10.1039/C2AN36168J
(Paper)
Analyst, 2013,
138, 129-136
Integration of multiple components in polystyrene-based microfluidic devices part I: fabrication and characterization†
Received
20th August 2012
, Accepted 16th October 2012
First published on 19th October 2012
Abstract
In Part I of a two-part series, we describe a simple and inexpensive approach to fabricate polystyrene devices that is based upon melting polystyrene (from either a Petri dish or powder form) against PDMS molds or around electrode materials. The ability to incorporate microchannels in polystyrene and integrate the resulting device with standard laboratory equipment such as an optical plate reader for analyte readout and pipets for fluid propulsion is first described. A simple approach for sample and reagent delivery to the device channels using a standard, multi-channel micropipette and a PDMS-based injection block is detailed. Integration of the microfluidic device with these off-chip functions (sample delivery and readout) enables high-throughput screens and analyses. An approach to fabricate polystyrene-based devices with embedded electrodes is also demonstrated, thereby enabling the integration of microchip electrophoresis with electrochemical detection through the use of a palladium electrode (for a decoupler) and carbon-fiber bundle (for detection). The device was sealed against a PDMS-based microchannel and used for the electrophoretic separation and amperometric detection of dopamine, epinephrine, catechol, and 3,4-dihydroxyphenylacetic acid. Finally, these devices were compared against PDMS-based microchips in terms of their optical transparency and absorption of an anti-platelet drug, clopidogrel. Part I of this series lays the foundation for Part II, where these devices were utilized for various on-chip cellular analysis.
A Introduction
The ability to perform fast/high-throughput analysis,1,2 utilize small sample volumes,3 and integrate multiple processes4–7 has made microfluidic-based systems an attractive platform for studying biological systems. The incorporation of biological cells onto microfluidic-based systems has become very popular due to the ability to fabricate structures with dimensions that can easily mimic in vivo environments.8–11
Our groups have published several works involving the immobilization and investigation of biological cells with microfluidic systems, with approaches that also integrate various analysis steps on the device. Previous work from the Martin group includes the development of microchip devices that integrated multiple processes such as cell immobilization, on-chip valving technologies, microchip electrophoresis, and either fluorescence or electrochemical detection.4,12 Microchip electrophoresis was useful in these studies because it enabled the determination of multiple analytes released from cells. Electrochemical detection was also beneficial, as it enabled direct, label-free detection with no derivatization steps being required.4 Complementary to efforts of integrating multiple steps along with analysis onto a single device, the Spence group has demonstrated the ability to incorporate multiple tissues and cell types (red blood cells, platelets, and endothelial cells) in a single microfluidic device in order to mimic the circulation and quantitatively study cell-to-cell communication.5,13 In addition to these studies, Spence's group has also recently begun to combine microfluidic-based systems with standard tools often employed in more biological settings. For example, the use of a standard high-throughput detection tool (plate reader) has been used to quantitate a biologically important analyte (nitric oxide, NO) released from red blood cells (RBCs) flowing in a microfluidic device.1 Furthermore, a joint study between the two groups showed that a transendothelial electrical resistance (TEER) can be integrated into a microfluidic device for real-time monitoring of cell layer integrity prior to studying the communication between flowing RBCs and endothelial cells within the same device.14
The majority of the systems cited above were fabricated with polydimethylsiloxane (PDMS), one of the most common materials used in microfluidic-based systems. PDMS is the most widely used microfluidic device substrate because it is inexpensive, transparent, flexible, amenable to rapid prototyping, and capable of being reversibly sealed to itself or other substrate materials.15 While PDMS devices have been successfully used in a variety of biological applications,1,4,12 some challenges arise when incorporating cells on devices fabricated from PDMS. For example, PDMS is a crosslinked polymer composed of hydrophobic dimethyl-siloxane oligomers, which can cause problems in cellular environments. Beebe's group was able to show that residual uncrosslinked monomers can leach from the bulk PDMS into cell culture media and small, hydrophobic molecules can also partition into the PDMS.16 While cell adhesion factors such as collagen or fibronectin can be coated onto PDMS surfaces to facilitate cellular adhesion, it would be advantageous to follow the lead of years of successful tissue culture in polystyrene flasks.
Fabrication of devices made from materials more established for cell culture, such as polystyrene, should lead to microfluidic devices that are more compatible for on-chip cell culture, as compared to use of PDMS.9 Recent work by multiple groups has focused on the fabrication of polystyrene devices for microarrays or cell-based assays.17–22 One example of this work includes development of a polystyrene device with micromolded arrays for the separation of adherent cells.19 Another is a recent study that investigated the biocompatibility of cells on different substrates including polystyrene.17 While these previous fabrication strategies were useful in demonstrating the advantages of using a biologically compatible substrate such as polystyrene, there has been a lack of integrating an analytical function to the devices so that intra- or extra-cellular processes can be monitored. Although polystyrene devices have mainly been fabricated via hot embossing,17,18 the integration of other components such as electrodes or connections for off-chip functions (such as pipets and/or pumps) has not been demonstrated.
In this two part work, we first describe a simple, and inexpensive, approach to fabricate polystyrene devices that is based upon melting polystyrene (from either a Petri dish or in a powder form) against PDMS molds or around electrode materials. This approach enables the incorporation of microchannels and integration of devices with such standard laboratory equipment as an optical plate reader for analyte readout. Furthermore, by employing a novel PDMS-injection block, a simple approach for sample and reagent delivery using a standard, multi-channel pipet is described. The fabrication of polystyrene-based devices with embedded electrodes is also demonstrated, with the approach enabling the incorporation of multiple electrode materials. It is also shown that a palladium decoupler and a carbon detection electrode can be integrated within the polystyrene substrate for use with microchip electrophoresis and electrochemical detection. Part I of this series lays the foundation for Part II, where these devices will be utilized for on-chip cellular analysis. The manner in which the devices are fabricated in Part I are shown to be critical for integrating an analysis step with on-chip cell culture.
B Experimental
Fabrication of polystyrene device with integrated channels
The general strategy for fabricating polystyrene devices with integrated channels is shown in Fig. 1. A PDMS mold with raised features was fabricated based on previously established soft lithography techniques,15,23 except a positive mask was used to produce recessed features in the master. Masters were fabricated on clean silicon wafers with SU-8 50 photoresist (Microchem Corporation, Newton, MA, USA) being spun at 500 rpm for 15 s and then 1000 rpm for 30 s, eventually leading to features that were 100 μm tall. The coated wafer was then exposed to ultraviolet light through a transparency positive mask containing 250 μm wide channels in a serpentine pattern. After development, this resulted in a master with recessed features. A 10
:
1 ratio of elastomer
:
curing agent (Sylgard 184, Ellsworth Adhesives, Germantown, WI, USA) was then poured over the completed master, cured at 75 °C, and subsequently removed from the master and sealed to a glass slide using a thin layer of uncured PDMS. A retaining ring, made from PDMS, was then sealed to the mold to eventually hold melted polystyrene. A surface modification on the PDMS was then performed by preparing a 0.1 M solution of (3-mercaptopropyl)-trimethylsiloxane (Sigma-Aldrich, St. Louis, MO, USA) in acetonitrile and filling the mold with this solution. The acetonitrile was allowed to evaporate, and then approximately 5 g of polystyrene from Petri dishes were heated above its transition temperature in the PDMS mold via contact heating on a hotplate set to 185 °C for 9 hours. The hotplate was then turned off and the polystyrene device cooled to room temperature before it was removed from the mold. Once the polystyrene device was removed from the mold, the channels were air plasma sealed to a 10
:
1 PDMS layer with 1/8 inch inlet and outlet wells for each serpentine channel. This PDMS layer was made by pouring the elastomer and curing agent mixture onto a blank silicon wafer. In order to achieve air plasma sealing, the PDMS and polystyrene layers were cleaned with isopropyl alcohol and doubly deionized water before being dried under vacuum for 5 minutes and then exposed to air plasma for 1 min.15 Immediately following exposure to plasma, the two layers were aligned by hand and sealed. To ensure permanent sealing, the device was placed in a 75 °C oven for 30 min.
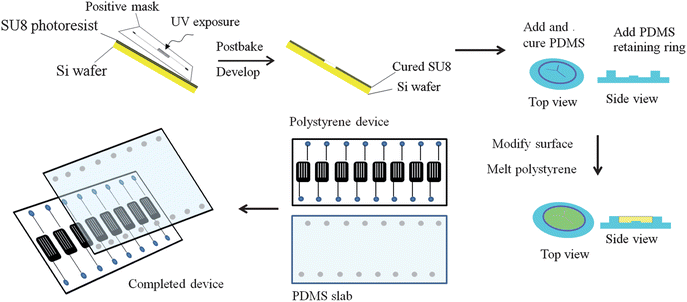 |
| Fig. 1 Fabrication of polystyrene devices with integrated channels. A silicon master is coated with SU-8 50 photoresist, and exposed to UV light through a positive photomask containing the serpentine features. The master is then developed and a PDMS replicate of the master is made. This PDMS mold is then sealed to a piece of glass, and a retaining ring of PDMS sealed along the outside. The PDMS is modified with 0.1 M (3-mercaptopropyl)-trimethylsiloxane, and polystyrene melted into the mold at 185 °C for 9 hours. The completed device is then removed and a PDMS cover slab with punched access holes is sealed to the polystyrene device through exposure to air plasma. | |
A PDMS injection block was also fabricated for sample delivery (Fig. 2). Pipet tips were suspended in a 10
:
1 ratio of elastomer
:
curing agent and cured for 30 min at 75 °C. The pipet tips were then removed and inlets were punched with a 20 gauge luer stub adapter. The PDMS injection block was then cut to the size of the polystyrene chip and air plasma sealed to the device in the same manner as described above.
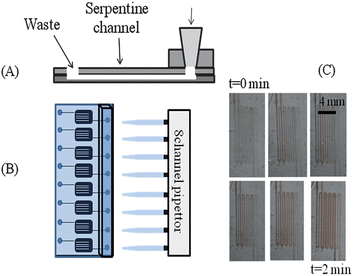 |
| Fig. 2 High-throughput pipet pumping in polystyrene based microfluidic device. (A) Side view of the device. A pipet block is molded from immobilized 200 μL pipet tips in PDMS. This is sealed to the cover slab of PDMS over an access hole. The polystyrene device containing the serpentine channels is then sealed to the PDMS components. (B) Top-view of the device, where an 8-channel pipet can individually and simultaneously address each channel. (C) Time-lapse photography of pumping RBCs through the serpentine channels using this system. | |
Red blood cell preparation for time lapse studies
Whole blood was collected from healthy, consenting donors via venipuncture into lithium heparin vacutainers. The whole blood was then centrifuged at 500 × g at 25 °C for 10 min and the plasma was collected for other experimentation. The remaining red blood cells (RBCs) were then resuspended and washed three times in a physiological salt solution (PSS) containing, in mM, 4.7 KCl, 2.0 CaCl2, 140.5 NaCl, 12 MgSO4, 21.0 tris(hydroxymethylaminomethane), 5.6 glucose and 5% bovine serum solution at a final pH of 7.4. The hematocrit of the RBCs was measured using a CritSpin® analyzer. The RBC solution used in the time lapse study was 7% RBCs in PSS.
Fabrication of polystyrene-encapsulated electrodes
A brief description of the polystyrene-encapsulated electrode fabrication procedure is provided here and demonstrated in Fig. 3. Electrode insertion holes were punched (1 mm away from one another) with a 20-gauge Luer stub adapter (Becton Dickinson, Sparks, MD, USA) into a 70 mL capacity disposable aluminum dish (6.7 cm in diameter, 1.6 cm in depth, Fisher Scientific, Springfield, NJ, USA). Electrodes (1 mm palladium, 1 mm glassy carbon, Alfa Aesar, Ward Hill, MA, USA) or P-25 carbon fiber bundle (Goodfellow, Oakdale, PA, USA) were affixed to copper extending wires via soldering or colloidal silver (Ted Pella, Redding, CA, USA). Heat shrink tubing was used to insulate the electrical connection before insertion into the aluminum dish. The electrodes were placed in a vertical orientation into the electrode insertion holes on a hot plate. Approximately 35 g of polystyrene powder (250 μm particle size, Goodfellow, Oakdale, PA, USA) was poured into the aluminum dish around the electrodes. The hot plate was then set to 250 °C and the powder was allowed to heat slowly for ∼2 hours. Polystyrene powder was replenished as needed in order to make a thicker substrate to support the electrodes. After 2 hours, the polystyrene was covered by another aluminum dish to allow for more uniform heating. Once the powder was completely melted, the substrate was allowed to cool to room temperature. The substrate was then removed from the aluminum dish and shaped by wet polishing. Wet polishing was achieved by using commercially available polishing pads (Buehler, Lake Bluff, IL, USA) and water. A range of grits (200–1200) were used, each for about two minutes. Uniformity can be achieved by rotating the electrode base while polishing or using a variable speed grinder-polisher (MetaServ 3000, Buehler, Lake Bluff, IL, USA), where the polishing pad rotates.
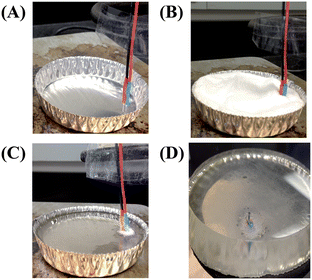 |
| Fig. 3 Fabrication of polystyrene-embedded electrodes. (A) A palladium decoupler and a detection electrode are affixed to connecting wires and are placed in a vertical position into an aluminum dish. (B) Commercially available polystyrene powder is poured around electrodes. (C) The polystyrene is heated to 250 °C for several hours. (D) Side-view of a polystyrene-embedded electrode base that has been removed from the mold and shaped by wet polishing. | |
Integration of polystyrene device with plate reader
In order to make the device amenable for measurement in a plate reader (Spectramax M4, Molecular Devices, Sunnyvale, CA, USA) a conventional, black, 96-well plate (Greiner Bio-One, Monroe, NC, USA) was modified by removing the bottom of wells with a rotary tool. This plate was then aligned over the serpentine channels in the fabricated polystyrene microfluidic device, and the assembly was inserted into the plate reader for analysis.1 Fluorescein standards were then pipetted into the device using the pipet interface block described above. Fluorescence measurements were taken using an excitation wavelength of 494 nm and an emission wavelength of 521 nm. Fluorescein standards were prepared in phosphate buffered saline at concentrations of 0, 18, 37, 75, and 150 μM. Standards were pumped through individual channels on the polystyrene device using a 100 μL pipet set to 40 μL.
Absorption of pharmaceutical compounds into PDMS and polystyrene devices
To determine the degree of absorption into each polymer, solutions of the molecule clopidogrel were prepared. Clopidogrel was initially dissolved in 0.1 M HCl in water, with subsequent dilutions made in a physiological salt solution (PSS) containing 4.7 mM KCl, 2.0 mM CaCl2 140.5 mM NaCl, 12 mM MgSO4, 21 mM tris(hydroxymethyl)aminomethane, and 11.1 mM glucose. Two layer PDMS devices and two layer PS devices were prepared for these studies. For both types of devices, the bottom layer was a featureless polymer sheet, and the top layer contained 1/8 inch holes. When sealed together, these two layers form a well. The PDMS layers were simply cured together, while the PS layers were thermally sealed together at 90 °C with slight pressure. Solutions of clopidogrel, varying in concentration between 0 and 2.0 μM, were then pipetted directly into these wells, and removed 30 minutes later. The “standard” referenced in Fig. 5 was simply clopidogrel solutions prepared in polyethylene micro centrifuge tubes. To determine the degree of absorption, these samples were separated using reverse phase LC with an Ascentis Express C18 3 cm × 2.1 mm column with 2.7 μm diameter particles (Supelco, Bellafonte, PA, USA). Subsequent analysis was then performed with multiple reaction monitoring mass spectrometry (MRM-MS) with electrospray ionization (ESI) on a Quattro Micro triple quadrupole mass spectrometer. Additional information about the MS analysis can be found in the ESI.†
Characterization of polystyrene-embedded electrodes
The polystyrene-based devices containing electrodes were characterized in terms of their optical transparency and the ability to perform electrophoretic separations. Transmittance data was conducted using a Cary Series UV-Vis Spectrophotometer (Agilent Technologies, Santa Clara, CA, USA) in single beam scanning mode. The % transmittance was measured from 200–600 nm on a polystyrene-embedded electrode base, polystyrene from cell culture flasks, and PDMS. All of the substrates had a 1 cm path length.
The PDMS-based fluidic channel used for the electrophoretic separation was fabricated based on previous methods23,24 utilizing soft lithography, silicon masters, and SU-8 10 photoresist. The channel height was measured using a profilometer (Dektak3 ST, Veeco Instruments, Woodbury, NY, USA). A 20
:
1 mixture of Sylgard 184 elastomer base (Ellsworth Adhesives, Germantown, WI, USA) was poured onto the silicon master and heated at 75 °C for 1 hour. The PDMS microchip was removed from the master and reservoirs were made with a hole punch. A LabSmith HVS448 3000 V high voltage (HV) sequencer with eight independent channels (LabSmith, Livermore, CA, USA) was used as the voltage source for the electrophoretic separation. A HV (+1000 V) was applied to the buffer reservoir, a fraction of the HV (+800 V) to the sample reservoir, with the sample waste reservoir and decoupler being grounded. A gated injection was made by floating the HV being applied to the buffer reservoir. The separation channel was 2.75 cm long, (field strength = 200 V cm−1) 24 μm tall, and 40 μm wide. The buffer used was 10 mM boric acid with 25 mM SDS (pH = 9.2). Amperometric detection was performed at +0.9 V with a CH Instruments potentiostat (Austin, TX, USA). The carbon fiber bundle was the detection electrode and a platinum wire served as the auxiliary and reference (quasi-reference) electrode.
C Results and discussion
Although PDMS has been widely used as a substrate material for microfluidic devices, limitations of its use for biological assays include poor cell adhesion, absorption of hydrophobic molecules, and swelling in organic solvents.9 In this work, an alternative microchip substrate (polystyrene) more suitable for cell culture has been utilized to address these limitations. There have been several reports of fabricating polystyrene-based microfluidic devices with hot embossing methods.17,18,22 One of the major objectives of the work reported here was to exploit the capabilities of already existing equipment typically used for soft-lithographic fabrication of PDMS, with the final devices also being amenable to integration of materials and tools often incorporated in PDMS-based microfluidic devices. Allbritton's group recently described an approach where gamma-butyrolactone (GBL) was used to dissolve polystyrene, and the resulting solution could be poured against a PDMS mold that contained raised features.25 The use of GBL did not cause swelling of the PDMS mold; the final devices were very thin (0.3 mm) and required full evaporation of the GBL solvent. Goral et al. also recently reported the use of PDMS-based molds for hot-embossing of polystyrene devices; however, there was no integration of microfluidics or analytical measurements with the final device.22 A goal of this work was to develop simple fabrication methods of microfluidic-based polystyrene devices that also enable the integration other device components such as an injection block (for interfacing with a pipet) and electrodes (for integrated detection). In these studies it was found that thicker chips (at least 3 mm for studies with microchannels/injection block and at least 1 cm for devices with embedded electrodes) were needed to integrate these other functionalities. This led to the development of a different strategy that is based upon melting of polystyrene (from either a Petri dish or in a powder form) on a conventional hotplate against a PDMS mold or around electrode materials to form thicker, robust devices that can be fabricated in a few hours with no issues with bubble formation or solvent evaporation. The strategies for integrating both on-chip functions (channels and electrodes) and off-chip interfaces (pipet for pumping and plate reader for detection) with polystyrene devices are outlined below.
Fabrication of polystyrene-based devices
Devices with integrated channels.
Fig. 1 outlines the process by which PDMS molds were created for subsequent channel formation in polystyrene devices. The process of fabricating a master is shown in Fig. 1, where a lithographically derived master with recessed features was used to create the PDMS mold with raised features. A retaining wall fabricated from PDMS was then sealed to this mold. It was determined that the mold and retaining ring should be of the same material to ensure equal thermal expansion. Thus, when pieces of a polystyrene-based Petri dish were melted onto the PDMS with retaining walls, serpentine channels were formed in the polystyrene. While upper and lower channel width limits were not determined here, in this study we routinely utilized channel widths ranging from 45 to 250 μm. In order to seal the channels on the polystyrene device, a 10
:
1 PDMS layer with an inlet and waste well for each serpentine channel was subjected to air plasma and irreversibly sealed to the polystyrene.
The last step in device construction involved the creation of an injection block that was molded out of PDMS using suspended pipet tips. The PDMS block was cut to size and aligned over the inlets that were punched in the PDMS layer sealed to the polystyrene. The block was then air plasma sealed in a similar manner to the PDMS layer and the final device is shown in Fig. 2. A unique feature of the injection block is that it is molded to support the use of pipets, thus enabling a standard pipet or fluidic injection system to serve as the device pump. Pipet tips, suitable for a 100 or 200 μL pipet, were used to create the mold and thus, deliver solutions to the serpentine channels and out the waste well (Fig. 2A). This allows for the use of pipets to induce flow rather than syringe-based pumps that involve multiple syringes and intensive rinsing procedures. A multi-channel pipet can be used to pump solution through 8 channels simultaneously (Fig. 2B). The image in Fig. 2C shows time-lapse figures of RBCs being pumped through such a device. The ability to propel blood samples through the device using an 8-channel pipet as the pump is significant on multiple platforms. First, blood is one of the most complex biological matrices; the ability to propel such a sample through the device strongly suggests that less-complex samples will be amenable to this delivery scheme. Secondly, biomarker discovery often includes screening of biological fluids such as blood or urine. The ability to propel such fluids through a microfluidic device in small volumes and in a high-throughput manner will have high impact in screening laboratories.
Devices with integrated electrodes.
The fabrication strategy shown in Fig. 3 was used to embed multiple electrode materials into a polystyrene base for use with microchip electrophoresis and electrochemical detection. The fabrication process for the embedded electrodes was optimized for minimal bubble formation on the polystyrene surface. If the polystyrene powder was overheated or not heated in a uniform manner, bubbles would form within the polystyrene base. Electrodes were soldered or connected with colloidal silver to an extending wire for the electrical connection. The electrodes were placed in a vertical fashion into a circular aluminum dish where electrode insertion holes were punched to fix the alignment of a palladium decoupler electrode and a detection electrode (Fig. 3A). A polystyrene powder was then distributed around the electrodes (Fig. 3B) and heated on a hotplate. Once the polystyrene powder was completely melted, the substrate was cooled to room temperature (Fig. 3C) and shaped by wet polishing. A range of grits (200–1200) was used to achieve a fine polish before reversible sealing of a PDMS microchip over the electrodes (Fig. 3D). After polishing, a typical device with embedded electrodes was 1.4 cm in thickness. This thickness, which was previously determined with epoxy-based encapsulation of electrodes,26,27 was needed to support the electrodes (with connecting wires), and to ensure that the devices can be re-used (and re-polished) for long periods of time. Devices have been re-used for up to a year (and counting) with this approach. It should be noted that attempts to make devices of this thickness using polystyrene dissolved in GBL25 were not successful, as complete solvent evaporation was not possible leading to a substrate that was not structurally stable enough for the incorporation of electrodes. The resulting device also had a large number of entrapped air pockets (bubbles) that made any type of optical detection difficult.
Optical characterization of polystyrene devices
One of the promises of microfluidic technologies that have not yet been completely fulfilled involves the concept of true high-throughput analyses. The lack of integrating microfluidic devices with standard high-throughput equipment is one possible reason that microfluidic devices have not been a major part of high-throughput screening or analyses. An optical plate reader is standard equipment in most high-throughput screening laboratories and recently we have show integration of PDMS-based devices with plate reader systems. A polystyrene-based device would potentially be a better fit for automation equipment due to its increased rigidity and potential to be readily moved by robotic equipment that transports microtiter plates from one location to another in a high-throughput screening laboratory. Here, we demonstrate that polystyrene-based devices are capable of integration with plate readers.
The serpentine channels on the polystyrene device were fabricated to have the same width and distance apart as wells on a standard 96-well plate so that they could be read out on a standard plate reader. Fluorescein standards were prepared in phosphate buffered saline at concentrations of 0, 18, 37, 75, and 150 μM and then used to optimize alignment of the polystyrene device in the plate reader. Standards were pumped through individual channels on the polystyrene device using a 100 μL pipet set to 40 μL (Fig. 4A). In order to make the device amenable for measurement in the plate reader, a 96-well plate was aligned on top of the device so that drilled-out wells corresponded to each channel's serpentine (Fig. 4B). Fluorescence measurements were then taken using an excitation wavelength of 494 nm and an emission wavelength of 521 nm. The alignment in the plate reader proved successful as fluorescence intensity was linear (R2 = 0.99) with concentration. The detection limit was not as good as a standard fluorescence detector (we were able to measure sub-micromolar levels of fluorescein), but these values should be able to be lowered by future strategies that increase the area of the channels under the detection window (the well itself); the serpentine channels were developed to improve the limits of detection. Detection limits can also be improved by increasing the gate time of the measurement on the plate reader itself.
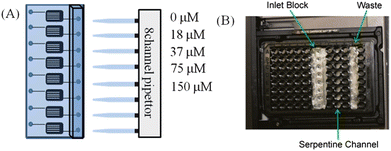 |
| Fig. 4 Pumping, alignment and fluorescence analysis from a polystyrene device. (A) Fluorescein standards are pumped with a pipet into the device. (B) The device is then placed in a plate reader and fitted with a microplate as a guide. Fluorescence analysis is performed with excitation of 494 nm and emission of 521 nm. | |
The same fabrication strategy can be utilized to produce polystyrene microchannels for electrophoretic separations. A standard T-configuration was fabricated within the polystyrene and used for electrophoresis with fluorescence detection. A PDMS slab with reservoirs punched with a hole punch was reversibly sealed over the polystyrene structure. As shown in the ESI (Fig. S4†), gated injections followed by electrophoretic separation and fluorescence detection could be performed in such a device.
To further demonstrate the capabilities of devices fabricated in polystyrene in comparison to PDMS-based devices, the absorption of an anti-platelet drug, clopidogrel, was investigated in PDMS and native polystyrene devices. A recent study reported the absorption properties of polystyrene that had been rendered hydrophilic via UV ozone treatment.17 As shown in Fig. 5, when incubated for 30 minutes in a device fabricated from PDMS alone, clopidogrel absorbs significantly into the device and cannot be recovered. However, when the same incubation is performed on a device fabricated solely from PS, the recovery of clopidogrel is statistically equivalent to that of the standards used in the MS analysis (which were stored in polyethylene centrifuge tubes), suggesting that absorption of clopidogrel does not occur in PS devices as shown in Fig. 4 (n = 3 devices, p < 0.05).
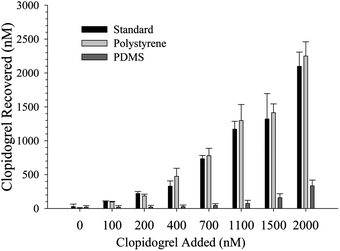 |
| Fig. 5 Clopidogrel absorption to polymer devices. At concentrations between 100 and 2000 nM, significantly less (n = 3 devices, p < 0.01) clopidogrel is recovered from PDMS devices than is from polystyrene devices, suggesting polystyrene-based microfluidic devices will be more important to studies investigating the effects of clopidogrel with in vitro platforms. Furthermore, standards incubated in polystyrene devices were shown to have a statistically equal recovery to standards prepared for MS analysis, suggesting clopidogrel does not absorb significantly to polystyrene. | |
One concern was the transparency of the thicker PS devices needed for integrating electrodes. The polystyrene-embedded electrode bases were characterized for % transmittance using a Cary Series UV-Vis Spectrophotometer in single beam scanning mode. The 1.4 cm thick polystyrene devices used for the embedded electrodes were utilized. The % transmittance was measured from 200–600 nm on three substrates (PDMS, polystyrene from cell culture flasks, and the fabricated polystyrene-embedded electrodes bases). One concern was the heating of the polystyrene powder and flasks would lead to a change in the transmittance properties of the resulting devices. The PDMS and fabricated polystyrene bases were cut so that the path length in the spectrophotometer was 1 cm. The polystyrene from the cell culture flasks was cut into thin sheets and bundled together to also form a 1 cm path length. As can be seen in Fig. 6, the % transmittance for the three substrates was similar. This shows that the devices fabricated in this study can be used for imaging studies similar to those performed in polystyrene-based cell culture flasks.
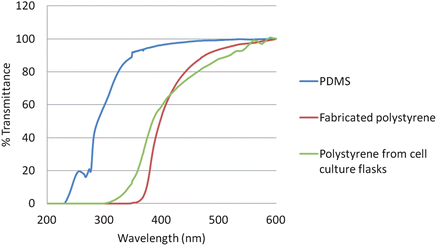 |
| Fig. 6 % Transmittance comparison of fabricated polystyrene bases, polystyrene from cell culture flasks, and PDMS. The % transmittance was measured from 200–600 nm using a spectrophotometer. All three substrates had a constant path length (b = 1 cm). | |
Microchip electrophoresis with electrochemical detection is an attractive approach for the monitoring of extracellular release from cells, as a variety of neurotransmitters can be separated and detected.28–30 Previous work from Martin's lab has focused on the integration of multiple processes such as cell culture and immobilization, on-chip valving technologies, microchip electrophoresis and electrochemical detection.4,12 Microchip electrophoresis was useful in these studies because it enables the analysis of multiple analytes, with electrochemical detection being advantageous because no derivatization step is needed and electrodes can be directly incorporated into a microchannel to minimize band broadening.24,31 A reproducible and simple way to integrate microchip electrophoresis with electrochemical detection is through the use of a palladium decoupler. Carbon electrodes are the preferred detection electrode material for detecting small molecule neurotransmitters due to minimal fouling, a lower overpotential, and a larger potential range for organic compounds.29,30 The palladium decoupler electrode serves as an electrophoretic ground and absorbs hydrogen produced from the reduction of water.24,31–34 In this work, a 1 mm palladium decoupler was embedded in polystyrene 1 mm away from either a 1 mm glassy carbon electrode (Fig. 7A) or a 100 μm carbon fiber bundle detection electrode (Fig. 7B).
The carbon fiber bundle detection was utilized for an electrophoretic separation of dopamine, epinephrine, catechol, and 3,4-dihydroxyphenylacetic acid (Fig. 7C). Carbon fiber electrodes are popular due to their small size and the ability to use them in vivo with fast scan cyclic voltammetry.26,35 To perform this analysis, a T-configuration PDMS-based microchip with a 27.5 mm separation channel was reversibly sealed over the electrodes. A gated injection was utilized to discretely inject a 140 pL plug and a 200 V cm−1 field strength was used to separate the mixture. The buffer used for this separation was 10 mM boric acid with 25 mM SDS (pH = 9.2). There was no significant difference in the EOF values for these PDMS/polystyrene devices versus an all PDMS device, most likely due to the electrophoresis channel being composed of 3 sides of PDMS.36,37 The resolution was 1.3 between dopamine and epinephrine, 3.1 between epinephrine and catechol, and 4.6 between catechol and 3,4-dihydroxyphenylacetic acid. The number of theoretical plates for catechol was 6000, which is typical for electrophoretic separations in PDMS-based microchannels.38,39 The electrode response was reproducible, with catechol having an average peak height of 2.14 ± 0.03 nA (1.4% RSD, n = 3). The results from embedding electrodes in polystyrene devices and utilizing the electrodes to integrate microchip electrophoresis with electrochemical detection holds great promise for future work on using this approach to separate and detect neurotransmitter release from cells cultured on the polystyrene devices.
D Conclusions
Microfluidic devices can be fabricated from polystyrene using standard soft-lithographic procedures and equipment. The preparation of such devices enabled us to leverage well-established procedures for electrode integration and optical detection schemes, previously developed for PDMS systems. In addition, the rigidity of polystyrene makes it more amenable to integration into equipment typically associated with high- throughput screening, such as plate readers and multi-channel fluidic delivery. Finally, and perhaps most significant, the polystyrene material is poised for enhanced compatibility for biologically related assays involving cells or for molecules containing a high degree of hydrophobicity. These advantages are demonstrated in Part II of this report.
Acknowledgements
Support for RSM came from the National Institute of General Medical Sciences (Award number R15GM084470-03). Support for DMS came from Merck. The authors would like to thank Dr Per Askeland for expertise in obtaining the SEM images. We would also like to thank the Michigan State University RTSF Mass Spectrometry Facility for instrument use.
References
- S. T. Halpin and D. M. Spence, Anal. Chem., 2010, 82, 7492–7497 CrossRef CAS.
- S. C. Jacobson, C. T. Culbertson, J. E. Daler and J. M. Ramsey, Anal. Chem., 1998, 70, 3476–3480 CrossRef CAS.
-
M. W. Li, A. L. Bowen, N. G. Batz and R. S. Martin, in Lab on a Chip Technology, Part II: Fluid Control and Manipulation, ed. K. E. Herold and A. Rasooly, Caister Academic Press, Nofolk, UK, 2009, pp. 385–403 Search PubMed.
- A. L. Bowen and R. S. Martin, Electrophoresis, 2010, 31, 2534–2540 CrossRef CAS.
- L. I. Genes, N. V. Tolan, M. K. Hulvey, R. S. Martin and D. M. Spence, Lab Chip, 2007, 7, 1256–1259 RSC.
- L. C. Mecker and R. S. Martin, Anal. Chem., 2008, 80, 9257–9264 CrossRef CAS.
- S. D. Noblitt, G. S. Lewis, Y. Liu, S. V. Hering, J. L. Collett and C. S. Henry, Anal. Chem., 2009, 81, 10029–10037 CrossRef CAS.
- J. El-Ali, P. K. Sorger and K. F. Jensen, Nature, 2006, 442, 403–411 CrossRef CAS.
- M. L. Kovarik, P. C. Gach, D. M. Ornoff, Y. Wang, J. Balowski, L. Farrag and N. L. Allbritton, Anal. Chem., 2012, 84, 516–540 CrossRef CAS.
- R. S. Martin, P. D. Root and D. M. Spence, Analyst, 2006, 131, 1197–1206 RSC.
- D. M. Spence, N. J. Torrence, M. L. Kovarik and R. S. Martin, Analyst, 2004, 129, 995–1000 RSC.
- M. W. Li and R. S. Martin, Analyst, 2008, 133, 1358–1366 RSC.
- C. Ku, T. Oblak and D. M. Spence, Anal. Chem., 2008, 80, 7543–7548 CrossRef CAS.
- P. A. Vogel, S. T. Halpin, R. S. Martin and D. M. Spence, Anal. Chem., 2011, 83, 4296–4301 CrossRef CAS.
- D. C. Duffy, J. C. McDonald, O. J. A. Schueller and G. M. Whitesides, Anal. Chem., 1998, 70, 4974–4984 CrossRef CAS.
- K. J. Regehr, M. Domenech, J. T. Koepsel, K. C. Carver, S. E. Ellison-Zelski, W. L. Murphy, L. A. Schuler, E. T. Alarid and D. J. Beebe, Lab Chip, 2009, 9, 2132–2139 RSC.
- P. M. van Midwoud, A. Janse, M. T. Merema, G. M. M. Groothuis and E. Verpoorte, Anal. Chem., 2012, 84, 3938–3944 CrossRef CAS.
- E. W. Young, E. Berthier, D. J. Guckenberger, E. Sackmann, C. Lamers, I. Eyvantsson, A. Huttenlocher and D. J. Beebe, Anal. Chem., 2011, 83, 1408–1417 CrossRef CAS.
- D. A. Detwiler, N. C. Dobes, C. E. Sims, J. N. Kornegay and N. L. Allbritton, Anal. Bioanal. Chem., 2012, 402, 1083–1091 CrossRef CAS.
- G. Mehta, J. Lee, W. Cha, Y.-C. Tung, J. J. Linderman and S. Takayama, Anal. Chem., 2009, 81, 3714–3722 CrossRef CAS.
- A. Chen, D. K. Lieu, L. Freschauf, V. Lew, H. Sharma, J. Wang, D. Nguyen, I. Karakikes, R. J. Hajjar, A. Gopinathan, E. Botvinick, C. C. Fowlkes, R. A. Li and M. Khine, Adv. Mater., 2011, 23, 5785–5791 CrossRef CAS.
- V. N. Goral, Y. C. Hsiesh, O. N. Petzold, R. A. Faris and P. K. Yuen, J. Micromech. Microeng., 2011, 21, 017002 CrossRef.
- R. S. Martin, A. J. Gawron, S. M. Lunte and C. S. Henry, Anal. Chem., 2000, 72, 3196–3202 CrossRef CAS.
- L. C. Mecker and R. S. Martin, Electrophoresis, 2006, 27, 5032–5042 CrossRef CAS.
- Y. Wang, J. Balowski, C. Phillips, R. Phillips, C. E. Sims and N. L. Allbritton, Lab Chip, 2011, 11, 3089–3097 RSC.
- A. S. Johnson, A. Selimovic and R. S. Martin, Electrophoresis, 2011, 32, 3121–3128 CrossRef CAS.
- A. Selimovic, A. S. Johnson, I. Z. Kiss and R. S. Martin, Electrophoresis, 2011, 32, 822–831 CrossRef CAS.
- J. L. Felhofer, L. Blanes and C. D. Garcia, Electrophoresis, 2010, 31, 2469–2486 CrossRef CAS.
- W. R. Vandaveer, S. A. Pasas, R. S. Martin and S. M. Lunte, Electrophoresis, 2002, 23, 3667–3677 CrossRef CAS.
- W. R. Vandaveer, S. A. Pasas-Farmer, D. J. Fischer, C. N. Frankenfeld and S. M. Lunte, Electrophoresis, 2004, 25, 3528–3549 CrossRef CAS.
- N. A. Lacher, S. M. Lunte and R. S. Martin, Anal. Chem., 2004, 76, 2482–2491 CrossRef CAS.
- T. K. Chen, G. Luo and A. G. Ewing, Anal. Chem., 1994, 66, 3031–3035 CrossRef CAS.
- M. L. Kovarik, M. W. Li and R. S. Martin, Electrophoresis, 2005, 26, 202–210 CrossRef CAS.
- J. A. Vickers and C. S. Henry, Electrophoresis, 2005, 26, 4641–4647 CrossRef CAS.
- M. L. Heien, P. E. M. Philips, G. D. Stuber, A. T. Seipel and R. M. Wightman, Analyst, 2003, 128, 1413–1419 RSC.
- D. Ross, T. Johnson and L. E. Locascio, Anal. Chem., 2001, 73, 2509–2515 CrossRef CAS.
- X. Ren, M. Bachman, C. Sims, G. P. Li and N. Allbritton, J. Chromatogr., B: Biomed. Sci. Appl., 2001, 762, 117–125 CrossRef CAS.
- J. A. Vickers, M. M. Caulum and C. S. Henry, Anal. Chem., 2006, 78, 7446–7452 CrossRef CAS.
- N. A. Lacher, N. F. de Rooij, E. Verpoorte and S. M. Lunte, J. Chromatogr., A, 2003, 1004, 225–235 CrossRef CAS.
Footnote |
† Electronic supplementary information (ESI) available: SEM and contact angle images of materials used in device preparation, additional mass spectrometry information, and information pertaining to electrophoresis experiments. See DOI: 10.1039/c2an36168j |
|
This journal is © The Royal Society of Chemistry 2013 |
Click here to see how this site uses Cookies. View our privacy policy here.