DOI:
10.1039/C3BM00001J
(Paper)
Biomater. Sci., 2013,
1, 669-678
Silica as a morphogenetically active inorganic polymer
Received
1st January 2013
, Accepted 13th March 2013
First published on 2nd April 2013
Abstract
At present the scaffolds used for bioprinting of cells do not elicit morphogenetic responses in the cells. In the present study we approached a solution by studying the effect of an inorganic silica supplement added to an Na-alginate matrix. Bone- and osteoblast-like SaOS-2 cells were embedded into this organic polymeric matrix which was additionally enriched with 400 μM prehydrolyzed TEOS [tetra-ethoxy-silane], a source of ortho-silicate. In this silica-based matrix the cells synthesized hydroxyapatite crystallites after exposure to a mineralization activation cocktail composed of β-glycerophosphate, ascorbic acid and dexamethasone. The degree of hydroxyapatite synthesis, determined by staining the cells with the OsteoImage dye, strongly increased after exposure of the cells to silica. In a previous study we reported that ortho-silicate induces the expression of the gene encoding BMP-2 [bone morphogenetic protein-2]. Now we asked the question whether, in the presence of the mineralization activation cocktail, silica induces differentially the fibrillar proteins type I collagen [COLI] and type V collagen [COLV], as well as the non-collagenous proteins alkaline phosphatase [ALP], osteopontin [OPN], osteonectin [ON], osteocalcin [OC], and bone sialoprotein II [BSP]. Those expression values were correlated with the transcript levels of RUNX2 [Runt-related transcription factor 2]. The data show that the steady-state transcript level of RUNX2 remained unchanged in the presence of silica, while this inorganic polymer caused an elevated BMP-2 transcript level, and simultaneously also a significant upregulation of the COLI, COLV, OPN and ON genes. In contrast, the level of expression of OC and BSP remained unchanged in the presence of silica. It is concluded that silica causes its morphogenetic effect with respect to some bone-specific genes, COLI, COLV, OPN and ON, in a RUNX2-independent way.
1. Introduction
The key cells controlling bone formation are the osteoblasts that derive from bone marrow stromal cells, also known as mesenchymal stem cells, and the multinuclear osteoclasts that originate from haematopoietic stem cells (reviewed in ref. 1). The differentiation of the mesenchymal stem cells to osteocytes and finally to the lining cells proceeds in several stages.2 During this differentiation route, the committed stromal cells of osteoblastic lineage interact with osteoclastic progenitor cells through cell-to-cell contact. By this, the osteoclasts undergo stimulation and fuse under the formation of multinucleated, active osteoclasts.3 This process is triggered by the interaction of the receptor activator of NF-κB (RANK), which is a membrane-bound cytokine-like molecule that is expressed at the surface of the hematopoietic osteoclastic progenitor cells,4,5 with the RANK ligand (RANKL). The differentiation of these two cell lineages proceeds in close association with an extracellular fibrillar scaffold, built mainly of collagen (see ref. 6).
It is generally assumed that bone development occurs either by direct transformation of connective tissue [intramembranous ossification] or by the replacement of an initial primordial “cartilaginous model” [endochondral or intracartilaginous ossification]; these processes might occur simultaneously.7 In both processes the differentiation of osteoblast stem cells to functionally active osteoblasts is the main driving force. During intramembranous ossification, bone develops along a rod of cartilage that dictates the final morphology and position of the bone. There, within the stromal connective tissue, the mesenchymal stem cells are connected by thin cell processes and are embedded in a cast of collagenous fibrils. During differentiation to the active osteoblasts the intercellular matrix densifies, becomes more homogeneous, and provides the platform for early calcification.8 During endochondral ossification bones elongate at a preformed cartilage template within the growth plate. In the course of bone formation the inorganic deposits are synthesized upon the primary spongiosa that develops further into the secondary spongiosa. The final bone deposits are modeled in a concerted action of the osteoblasts and osteoclasts.9,10
The central anabolically acting cells of osteogenesis are the osteoblasts that derive from the osteoprogenitor cells. They are located within the deeper layer of periosteum and the bone marrow.11 RUNX2 [Runt-related transcription factor 2]/Cbfa1 [core-binding factor subunit alpha-1] has been considered as one of the master regulatory transcription factors that is associated with osteoblast differentiation and is expressed in those cells that belong to the osteoblastic lineage.12 RUNX2 is again under the control of fibroblast growth factor 2 and noggin.13 Noggin, a phylogenetically old signaling molecule,14 interacts in an antagonistic action with the bone morphogenetic proteins [BMPs] during the initial differentiation of the osteoprogenitor cells.15
Another key controlling molecule of osteogenesis is BMP-2. In contrast to RUNX2, BMP-2 is not a transcription factor but a ligand of a heterotetrameric complex of types I and II transmembrane serine/threonine kinase receptors [BMPR2].16 BMPR2 binds to BMP-7, BMP-2 and, less efficiently, BMP-4. After ligand binding, a receptor complex consisting of two type II and two type I transmembrane serine/threonine kinases is formed that – after phosphorylation – activates SMAD transcriptional regulators.17 BMP-2 has been identified to induce osteoblast differentiation through the expression of RUNX2-dependent ATF6, a basic leucine zipper-transcription factor;18Fig. 1. Osteoblasts differentiate from their stem cell precursors through a multi-step molecular pathway regulated by a series of transcription factors and signaling proteins, e.g. Indian Hedgehog, Runx2, Osterix, and also Wnt signaling pathway protein.19 More recently experimental evidence has been presented that proposes also an independent signaling pathway between Runx2 and BMP-2.20 Moreover, sclerostin, a secreted glycoprotein,21 competes with BMPs for binding to BMPRs, and by that decreases BMP signaling function and, in turn, suppresses mineralization of osteoblastic cells.22 Finally, the circulating sclerostin levels are reduced by parathyroid hormone [PTH].23
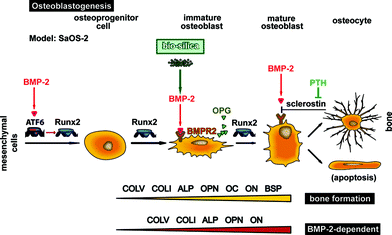 |
| Fig. 1 Complex regulation of osteoblastogenesis by at least two transcription factors, ATF6 and RUNX2. In the schematic outline it is highlighted that BMP-2 activates the transcription factor RUNX2 via ATF6. Subsequently, this cytokine binds to its receptor BMPR additionally inducing the differentiation pathway of osteoprogenitor cells (mesenchymal cells) to osteoblasts. On this differentiation pathway BMP-2 synthesis increases in response to silica/biosilica and triggers further the differentiation to mature osteoblasts. In addition, in response to silica/biosilica the osteoclastogenesis inhibitory factor OPG is induced in osteoblasts, which in turn inhibits RANKL, an essential activator of osteoclast differentiation. The interaction of BMP-2 with its receptor BMPR is inhibited by sclerostin, a glycoprotein whose synthesis is regulated by the PTH hormone. The mature osteoblast can undergo apoptosis or remains entrapped in the mature bone as an osteocyte. The expression studies suggest that genes encoding for COLI, COLV, OPN and ON are dependent on the activation of the BMP-2 signaling (RUNX2-independent) pathway. For the studies here, SaOS-2 cells have been used. | |
Testing the effect of silica-based bioactive glasses on BMP-2 gene expression it was found that those glasses induce in SaOS-2 cells not only BMP-2 expression but also an enhanced steady-state transcript level for alkaline phosphatase and collagen type I.24 In continuation, and using likewise SaOS-2, our group found that silica and biosilica, formed enzymatically from ortho-silicate and silicatein,25 induce BMP-2 expression in SaOS-2 cells.26 Furthermore, silica causes an increased transcript level for osteoprotegerin [OPG],27 a decoy receptor for RANKL, and by that inhibits the function of RANKL as a mediator for osteoclast differentiation.28 Recently our data have been confirmed.29
Osteoblasts synthesize both cytokines that regulate the growth of osteoclasts and structural proteins, required for hydroxyapatite synthesis.30,31 Among the soluble signaling molecules is osteoprotegerin that scavenges the soluble RANKL and, by that, inhibits the function of RANKL to induce the differentiation to multicellular osteoclasts.32 A further soluble bone-specific signaling molecule is osteocalcin [OC], also termed bone γ-carboxyglutamic acid-containing protein. It is a peptide hormone formed by osteoblasts and binds there to hydroxyapatite.33 The synthesis of OC is restricted to the osteoblast lineage and parallels with the differentiation of those cells.34 Experiments with osteocalcin-deficient mice suggested that OC inhibits bone formation.35
During differentiation of osteoprogenitors to osteoblasts these bone-forming cells produce the following functional and structural proteins that are required for bone formation. The enzyme alkaline phosphatase [ALP] exists with high levels in the region of highest ossification.36,37 Among the structural molecules collagen type I and type V are the quantitatively dominant proteins.6 Both collagen fibrils form the cast around the growing bone. The type V collagen [COLV] molecules are primarily cross-linked to each other and are oriented as a head-to-tail linear polymer; they become laterally linked to type I collagen [COLI] as copolymeric fibrils.38 Among the soluble, non-collagenous proteins that are synthesized by the osteoblast is osteopontin [OPN], also termed bone sialoprotein I [BSP-1]. BSP-1 is a glycoprotein that is formed by pre-osteoblasts, osteoblasts, osteocytes, as well as odontoblasts, and also other less-specialized cells, e.g. fibroblasts.39 Besides stimulating a series of interactive signaling pathways in osteoblasts this sialoprotein binds via its polyaspartic acid sites to hydroxyapatite. Finally, osteonectin [ON], also known as secreted acidic protein [rich in cysteine], is a glycoprotein in the bone that binds to sodium ions.40 It is synthesized by osteoblasts, and binds to hydroxyapatite and collagen during the synthesis of apatite crystals. In turn, ON is a bone-specific protein that acts at the inter-phase between the bone mineral and the collagen phases. Bone sialoprotein II [BSP] is a significant and integrated component of the inorganic part of the bone tissue and constitutes about 10% of the non-collagenous proteins.41 BSP strongly stimulates the migration of osteoprogenitors in concert with MMP-2 [matrix metallopeptidase 2] and integrin under the formation of a triple complex.
In the present study we embedded SaOS-2 cells in an Na-alginate-based hydrogel and supplemented this matrix with silica (400 μM prehydrolyzed TEOS [tetra-ethoxy-silane]) in order to clarify whether this inorganic silica component provides alginate with a morphogenetic potential. The alginate had been determined to be a suitable matrix for three-dimensional growth of the neuronal cells of the nucleus pulposus.42 The data show that among the genes studied here, the expression level of COLI, COLV, OPN and ON is significantly upregulated after exposure of the hydrogel-embedded cells with silica. In addition, the data show that these changes of the gene expression are independent of a concomitant change of the level of RUNX2 transcripts.
2. Materials and methods
2.1 Materials
The following materials were obtained. Sodium alginate (Cat. No. CAA W20,150-2), β-glycerophosphate, ascorbic acid, dexamethasone, trypan blue, and tetra-ethoxy-silane [TEOS] from Sigma-Aldrich (Taufkirchen, Germany); OsteoImage Mineralization Assay from Lonza Cologne (Köln, Germany); McCoy's medium from Biochrom (Berlin, Germany); fetal calf serum from GIBCO (Grand Island, NY, USA); the sources of all reagents needed for the described molecular biological assays have been listed recently.43
2.2 Incubation conditions for SaOS-2 cells
Human osteogenic sarcoma cells, SaOS-2,44 were cultivated in McCoy's medium containing 2 mM L-glutamine, gentamicin (50 μg mL−1), penicillin (100 U mL−1), and streptomycin (100 μg mL−1) in 25 cm2 flasks (Orange Scientifique, Braine-l'Alleud, Belgium), as described.27,45 The medium had been supplemented with 5% heat-inactivated FCS [fetal calf serum]. Routinely, the cells were seeded at a concentration of 105 cells mL−1. Every 3rd day the culture medium/FCS was changed. The cultures were incubated in a humidified atmosphere containing 5% CO2 at 37 °C. Where indicated, the cells in the medium/FCS were exposed to the mineralization activation cocktail composed of 5 mM β-glycerophosphate, 50 mM ascorbic acid and 10 nM dexamethasone.27,46
2.3 Preparation of alginate/silica composite hydrogel beads
An Na-alginate solution was prepared in McCoy's medium in the absence of FCS and allowed to dissolve at 65 °C for 2 h while shaking. The clear solution (4% [w/v]) was cooled down to 37 °C and the cells were added; the final SaOS-2 cell concentration was 2 × 106 cells mL−1. The final Na-alginate concentration was adjusted to 1.2% [w/v]. Those hydrogel samples were termed HG. Where indicated, the alginate hydrogel was supplemented with 400 μM prehydrolyzed TEOS, prepared as described;25 this composite hydrogel is termed Si-HG. The hydrogel beads were prepared from HG or from Si-HG by passing the cell suspension through a needle (dimension: 0.45 × 23 mm), attached to a 1 mL syringe. The cell-hydrogel suspensions were dropped into a 1.5% [w/v] CaCl2-solution. After 5 min, approximately 0.4 mm large beads were formed which were washed three times in saline and then twice in McCoy's medium. Finally, the beads (approximately 60 beads) were placed in 24-well plates (Nunc, Langenselbold, Germany) and incubated in 3 mL McCoy's medium together with FCS. If the beads contained silica also the medium in which they were incubated was supplemented with 400 μM prehydrolyzed TEOS. Culture medium/FCS was changed every 3rd day.
To release, after incubation, the cells from the hydrogel matrix, the beads in each well were transferred into 2 mL of 55 mM Na-citrate; after 30 min at 37 °C the cells could be collected by centrifugation at 900g for 5 min.47
The number of viable cells, after liberating from the hydrogel matrices, was determined with a trypan blue solution as described.48,49
2.4 Quantitative real-time RT-PCR (qRT-PCR) analysis
Applying the technique of quantitative real-time RT [reverse transcription]-PCR (qRT-PCR) the levels of transcription of the following genes were quantified in SaOS-2 cells, as described.27,45 The following primer pairs had been used: RUNX2 [Runt-related transcription factor 2; variant-3; accession number NM_004348.3] Fwd: 5′-TACAGACCCCAGGCAGGCACAG-3′ [nt1213 to nt1234] and Rev: 5′-TCGGTGATGGCAGGAAGCCCAG-3′ nt1359 to nt1338] (147 bp); BMP-2 [bone morphogenetic protein-2; NM_001200.2] Fwd: 5′-ACCCTTTGTACGTGGACTTC-3′ [nt1681 to nt1700] and Rev: 5′-GTGGAGTTCAGATGATCAGC-3′ [nt1785 to nt1804; 124 bp]; ALP [alkaline phosphatase; NM_000478.4] Fwd: 5′-TGCAGTACGAGCTGAACAGGAACA-3′ [nt1141 to nt1164] and Rev: 5′-TCCACCAAATGTGAAGACGTGGGA-3′ [nt1418 to nt1395; 278 bp]; COLI [collagen type I; NM_000088] Fwd: 5′-TATGGGACCCCAAGGACCAAAAGG-3′ [nt1122 to nt1145] and Rev: 5′-TTTTCCATCTGACCCAGGGGAACC-3′ [nt1234 to nt1257] (136 bp); COLV [collagen type V; NM_000093] Fwd: 5′-ATGCAGTTCCAGCTTAGGAAGCCAC-3′ [nt7725 to nt7749] and Rev: 5′-GCCAGACTTTTCTTTGGCAAGAGCG-3′ [nt7844 to nt7820; 120 bp]; OPN [osteopontin; NM_000582] Fwd: 5′-GGACATCACCTCACACATGGAAAGC-3′ [nt684 to nt708] and Rev: 5′-GTTTCATAACTGTCCTTCCCACGGC-3′ [nt803 to nt827; 120 bp]; ON [osteonectin; NM_003118] Fwd: 5′-GGCAAAGGGAAGTAACAGACACACG-3′ [nt1553 to nt1577] and Rev: 5′-CAGTTACAGCCTCAGGCAAACCAC-3′ [nt1675 to nt1698] (123 bp); OC [osteocalcin; NM_199173.4] Fwd: 5′-CCCTCACACTCCTCGCCCTATT-3′ [nt80 to nt101] and Rev: 5′-ATAGGCCTCCTGAAAGCCGATGT-3′ [nt351 to nt329; 272 bp]; BSP [bone sialoprotein II; NM_004967] Fwd: 5′-ACGAACAAGGCATAAACGGCACC-3′ [nt613 to nt635] and Rev: 5′-CTTCTGCATTGGCTCCAGTGACAC-3′ [nt735 to nt758] (123 bp); and for the reference gene GAPDH [glyceraldehyde 3-phosphate dehydrogenase; NM_002046.3] Fwd: 5′-CCGTCTAGAAAAACCTGCC-3′ [nt845 to nt863] and Rev: 5′-GCCAAATTCGTTGTCATACC-3′ [nt1059 to nt1078; 215 bp].
The cells were released from the hydrogel samples and then collected by centrifugation. The RNA was extracted using the TRIzol reagent50 and subjected to qRT-PCR.27 Then, 2 μL of the appropriate dilution were employed as a template in the 30 μL qRT-PCR assays.27 All reactions were run with an initial denaturation at 95 °C for 3 min, followed by 40 cycles, each with 95 °C for 20 s, 58 °C for 20 s, 72 °C for 20 s, and 80 °C for 20 s. Fluorescence data were collected at the 80 °C step. Quantitative real-time PCR experiments were performed in an iCycler (Bio-Rad). The mean Ct values and efficiencies were calculated by iCycler software (Bio-Rad, Hercules, CA, USA); the estimated PCR efficiencies were in the range of 93%–103%. Expression levels of the respective transcripts were correlated to the reference gene to determine relative expression, as described.27,51
Bright field analyses were performed with a VHX-600 Digital Microscope from KEYENCE (Neu-Isenburg, Germany), equipped with a VH-Z25 zoom lens (25× to 175× magnification).
2.6 OsteoImage mineralization assay
The hydroxyapatite crystallites formed were stained with the “OsteoImage” staining dye following the protocol supplied by the manufacturer and following the described application procedure.52 It has been proposed that the “OsteoImage” staining dye specifically binds to hydroxyapatite and is even more sensitive than Alizarin red S, which binds to calcium only.52 The stained specimens were inspected with a fluorescence microscope, using a laser with an excitation/emission wavelength of 492 nm/520 nm.
2.7 Mechanical studies
Mechanical properties of the hydrogel samples were determined with a NanoTest Vantage system (Micro Materials Ltd, Wrexham, UK), equipped with a Berkovich diamond indenter as described.53 The indenter used had a tip radius of approximately 50–100 nm. The determinations (20 replications per measuring point) had been performed with a maximum load of 20.1 mN. The first contact of the indenter had a surface approach velocity of 0.5 μm s−1; the initial contact force was kept constant at 0.1 mN. Since the hydrogels show a time-dependent plasticity, the “creep-effect”, a dwell period of 30 s had been selected. The Martens Hardness and the reduced modulus of the specimen were determined as described54 and using the software “NanoTest Platform Four V.40.08 (Micro Materials Ltd)”.
2.8 Statistical analysis
The results were statistically evaluated using the paired Student's t-test.55
3. Results
3.1 Embedding SaOS-2 cells into Na-alginate hydrogel
SaOS-2 cells at a high density of 2 × 106 cells mL−1 were encapsulated in Na-alginate hydrogel beads and incubated in McCoy's medium/FCS. The medium was further enriched with mineralization activation cocktail to induce the cells to hydroxyapatite deposition. In addition, the cells – if growing in a medium enriched with the cocktail – show an adequate degree of survival. One week after embedding into the hydrogel the cell density increased to 120 ± 24% when compared with the initial density during gel formation. If those cells (Fig. 2A-a) were stained with the “OsteoImage” staining dye a distinct green fluorescence, indicating hydroxyapatite, can be visualized by light microscopy (Fig. 2A-b). The staining patterns match the fluorescent images published earlier.52 Previously, we described that onto SaOS-2 cells hydroxyapatite/calcium and phosphorus-containing crystallites are formed.26 These analyses had been performed by EDX (energy dispersive X-ray spectroscopy).
![SaOS-2 cells embedded in Na-alginate hydrogel. (A-a and A-b) The SaOS-2 cells were incubated in the mineralization activation cocktail for one week [bright field image] (A-a). Then the cells, exposing their hydroxyapatite crystallites, were stained with the “OsteoImage” staining dye [fluorescent image; the mineralized crystallites are highlighted in green and the signals outshine the shadowy cell clump] (A-b); a distinct green fluorescence staining could be recorded. However, if the cells were embedded in the hydrogel that had been supplemented with 400 μM ortho-silicate [bright field] (A-c) and then stained with the “OsteoImage” dye an intensive green fluorescence can be monitored [fluorescent image] (A-d). (B) Influence of the 400 μM ortho-silicate supplement to the hydrogel beads and to the medium on the expression of the ALP gene in SaOS-2 cells. The cells were additionally exposed to the mineralization activation cocktail. The levels of ALP mRNA in SaOS-2 cells were determined by the absence of silica (open bars) or the presence of silica (filled bars). The cells were harvested at the beginning of the experiments (seeding time) or after incubation in the beads for 1 or 2 weeks. Subsequently, RNA was extracted and subjected to quantitative real-time RT-PCR (qRT-PCR) analysis for both ALP mRNA and GAPDH transcripts. The expression level of ALP was normalized to the expression of GAPDH. Data are expressed as mean values ± SD for five independent experiments; each experiment was carried out in duplicate. Differences between the groups were evaluated using an unpaired t-test, *p < 0.05.](/image/article/2013/BM/c3bm00001j/c3bm00001j-f2.gif) |
| Fig. 2 SaOS-2 cells embedded in Na-alginate hydrogel. (A-a and A-b) The SaOS-2 cells were incubated in the mineralization activation cocktail for one week [bright field image] (A-a). Then the cells, exposing their hydroxyapatite crystallites, were stained with the “OsteoImage” staining dye [fluorescent image; the mineralized crystallites are highlighted in green and the signals outshine the shadowy cell clump] (A-b); a distinct green fluorescence staining could be recorded. However, if the cells were embedded in the hydrogel that had been supplemented with 400 μM ortho-silicate [bright field] (A-c) and then stained with the “OsteoImage” dye an intensive green fluorescence can be monitored [fluorescent image] (A-d). (B) Influence of the 400 μM ortho-silicate supplement to the hydrogel beads and to the medium on the expression of the ALP gene in SaOS-2 cells. The cells were additionally exposed to the mineralization activation cocktail. The levels of ALP mRNA in SaOS-2 cells were determined by the absence of silica (open bars) or the presence of silica (filled bars). The cells were harvested at the beginning of the experiments (seeding time) or after incubation in the beads for 1 or 2 weeks. Subsequently, RNA was extracted and subjected to quantitative real-time RT-PCR (qRT-PCR) analysis for both ALP mRNA and GAPDH transcripts. The expression level of ALP was normalized to the expression of GAPDH. Data are expressed as mean values ± SD for five independent experiments; each experiment was carried out in duplicate. Differences between the groups were evaluated using an unpaired t-test, *p < 0.05. | |
In parallel, the cells were embedded in the Na-alginate hydrogel together with 400 μM prehydrolyzed TEOS, to prepare 400 μM ortho-silicate, and continued to be incubated in McCoy's medium/FCS additionally enriched with 400 μM ortho-silicate. Analyzing those cell populations (Fig. 2A-c) after staining with the “OsteoImage” stain (Fig. 2A-d) the individual cells show a bright brilliant green staining, indicating a strong hydroxyapatite formation by the SaOS-2 cells. In the controls, cells not treated with the mineralization activation cocktail, no fluorescence staining could be recorded (not shown).
3.2 Morphological properties and mechanical stability of the hydrogels
The surface roughness of hydrogels did not exceed 100 nm for both the silica-free Na-alginate hydrogel [HG] and the hydrogel supplemented with 400 μM prehydrolyzed TEOS [Si-HG]. Hardness measurements were performed with the sharp triangular Berkovich diamond indenter (tip radius: 50–100 nm; Fig. 3), as described under “Materials and methods”. The Martens hardness and the reduced elastic modulus were from 20 measurements; a Martens hardness of 0.53 ± 0.03 GPa [for HG] and 0.72 ± 0.04 [for Si-HG], and a reduced elastic modulus of 20.336 ± 0.812 GPa [for HG] and 22.826 ± 0.579 GPa [Si-HG] were measured.
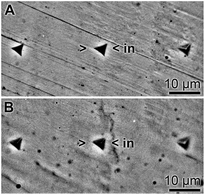 |
| Fig. 3 Surface characteristics of the alginate hydrogel in the absence of silica (HG; A), as well as in the presence of silica (Si-HG; B). The indentation marks (>in<) in these light microscopic images have been set with a Berkovich diamond and show the characteristic triangular shape. | |
3.3 Gene expression studies of SaOS-2 cells
The technique of qRT-PCR was applied to quantify the transcript level of the respective genes. The steady-state expression of the house-keeping gene GAPDH was used as a reference. For these experiments the cells were incubated with the mineralization activation cocktail.
Alkaline phosphatase.
The ALP gene expression in dependence on the duration of the incubation was determined under cultivation conditions during which the cells had been embedded in Na-alginate hydrogel. In the absence of silica the relative expression level of ALP (with respect to GAPDH) varied insignificantly during the first week around 0.02 (Fig. 2B). During the second week of incubation the relative transcript level increased slightly from 0.018 ± 0.002 (time zero) to 0.027 ± 0.004 (2 weeks of incubation). In contrast, if the alginate beads and the incubation medium contained 400 μM ortho-silicate, a strong and significant increase of the transcript level was seen already after 1 week (from 0.015 ± 0.002 to 0.067 ± 0.012), a value that leveled off after 2 weeks to 0.052 ± 0.010 (Fig. 2B).
Collagens.
Two dominant types of collagen were selected for qRT-PCR analysis: collagen type I [COLI] (Fig. 4A) and collagen type V [COLV] (Fig. 4B). The overall expression level of COLI was substantially higher (0.29 with respect to GAPDH) than the one for COLV (0.031). In the absence of silica the levels of both COLI and COLV remained almost unchanged during the two-week incubation period; only a slight but significant increase of the COLI expression from 0.29 ± 0.07 (at the time of seeding) to 0.41 ± 0.09 after a two weeks incubation was measured. If the embedded SaOS-2 cells were exposed to silica, significant increases of the COLI expression from 0.25 ± 0.06 to 0.92 ± 0.19 and for COLV from 0.032 ± 0.006 to 0.065 ± 0.011 had been determined after an incubation period of 1 week. These elevated levels remained high also for the following one week of incubation.
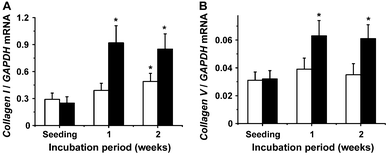 |
| Fig. 4 Altered expression of the collagen genes of type I (COLI) (A) and type V (COLV) (B) in dependence on the presence of silica. Again it was measured that in the presence of silica (filled bars) the transcript level for the respective collagen genes is significantly higher after a one or two weeks incubation period, as compared with the expression of the genes seen in cells not exposed to silica (open bars). Further details are given in Fig. 2B, and in the text. | |
Osteopontin.
The expression of OPN in the absence of silica remained unchanged in SaOS-2 cells if they were maintained in hydrogel; a basis template level of 0.0045 with respect to GAPDH is measured (Fig. 5A). Again, if 400 μM silica is added to the hydrogel and also to the medium the steady-state level of OPN increases significantly by over 2-fold (Fig. 5A).
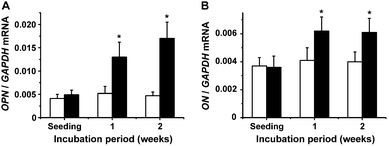 |
| Fig. 5 Effect of silica on the expression of osteopontin (OPN) (A) and osteonectin (ON) (B) in the absence (open bars) or presence of silica (filled bars). | |
Osteonectin.
Likewise, the expression of ON remained unchanged in SaOS-2 cells in the absence of a silica supplementation (Fig. 5B); a basis level of around 0.004 is determined. If the cells were incubated in the presence of silica the level of ON transcripts significantly increases to >140% already after 1 week, a level that was maintained also after 2 weeks (Fig. 5B).
Osteocalcin.
The OC expression level remained during the 2 weeks-incubation period almost unchanged; a basis level of 0.02, with respect to GAPDH, is measured irrespective of the presence or absence of silica (Fig. 6A).
 |
| Fig. 6 Gene expression of osteocalcin (OC) (A) and bone sialoprotein II (BSP) (B) in SaOS-2 cells in the absence (open bars) or presence of silica (filled bars). | |
Bone sialoprotein II.
Like for OC also for BSP no significant change of the steady-state expression of the transcripts is measured for the 2 weeks incubation (Fig. 6B). The values vary insignificantly around 0.025.
Runt-related transcription factor 2.
The gene expression of the transcription factor RUNX2 was determined during the 2 weeks incubation period in SaOS-2 cells. They were cultivated either without supplemented silica or became exposed to silica during this period. No significant change was measured by qRT-PCR (Fig. 7A).
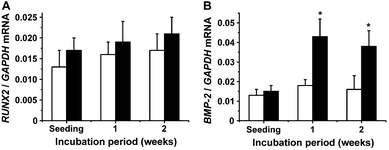 |
| Fig. 7 Gene expression of Runt-related transcription factor 2 (Runx2) (A) and bone morphogenetic protein-2 (BMP-2) (B) in SaOS-2 cells. The cultures were kept in the absence (open bars) or presence of silica (filled bars). | |
Bone morphogenetic protein-2.
Embedded in Na-alginate hydrogel the gene expression level for BMP-2 did not change during the two weeks after the start of the incubation (Fig. 7B). However, after supplementing the incubation system with 400 μM ortho-silicate the steady state expression increased from 0.015 ± 0.003 at seeding time significantly to 0.043 ± 0.09, with respect to the expression of GAPDH, after the first week of incubation. In the subsequent weeks this level remained unchanged (Fig. 7B).
4. Discussion
In the present study SaOS-2 cells were embedded into Na-alginate, supplemented with silica. Silica has been found (see “Introduction”) to display a morphogenetic activity towards SaOS-2.26,27,29,56 This biological activity of silica is retained by SaOS-2 cells that have been encapsulated into Na-alginate.53 Considering the results that the viability of the embedded SaOS-2 is enhanced in the presence of the mineralization activation cocktail,53 gene expression studies were performed in the presence of ascorbic acid, dexamethasone and the phosphate donor β-glycerophosphate. While ascorbic acid and dexamethasone alone have no mineralization-enhancing capacity, β-glycerophosphate alone is sufficient to cause mineralization in murine mammary adenocarcinoma 4T1 cells.57
The proliferation and differentiation of mesenchymal osteoprogenitor cells to mature osteoblasts are under the control of several transcription factors with RUNX2 and ATF6 being the major controlling players. It was the aim of the present study to determine the fine tuning and the mode of action of silica/biosilica on the differentiation and mineralization capacity of SaOS-2 cells.58 SaOS-2 cells can be induced to synthesize BMP-2,59 ALP, OPN, OC and ON,60 collagen I, collagen V and BSP;61Fig. 1. Expression of these genes along the differentiation pathway from the osteoprogenitor cells to the osteoblasts is required for the synthesis of hydroxyapatite in the functionally active osteoblasts.
Until now it has been unclear whether the induction of the genes encoding for the mentioned bone-specific proteins is under the control of the transcription factor RUNX2 or of BMP-2 and its down-stream signaling molecules. As inductors for both BMP-2 and RUNX2 in SaOS-2 cells silica/biosilica can be applied. Focusing on BMP-2, it has been thoroughly described that silica/biosilica induces this cytokine.26 In contrast the effect of silica/biosilica on RUNX2 remained to be studied, and performed in this report. RUNX2 in SaOS-2 has been considered to control the expression of a series of early and late genes involved in osteoblastogenesis.62,63
In the present study we could demonstrate that silica has no effect on the steady-state expression level of RUNX2. In consequence and considering the existing data that besides the transcription factor RUNX2 it is the cytokine BMP-2 that controls the expression of those genes encoding for the bone-specific protein, we postulate, at the present state of knowledge, that those genes that are upregulated in SaOS-2 cells in response to silica are the result of the BMP-2-dependent and RUNX2-independent signaling pathway,19 while the other inducible genes in bone cells are under the control of the RUNX2 pathway.18
The organic components of bone tissue can be grouped into collagenous and non-collagenous proteins.64 The genes for both collagen types studied here, collagen type I and collagen type V, become strongly upregulated by silica. These two collagens form the basic building block around which the hydroxyapatite crystals are deposited.65 With respect to cortical and trabecular bone the collagen fibrils are laid down in an alternating orientation.66 Collagen type I, with 85% the dominant component of the organic part of the bone, is considered to dictate both the location and organization of the mineral deposits in the growing bone.67 This process is accelerated by the property of this collagen to induce self-assembly under the formation of staggered molecules.68 Collagen type V exists in trace amounts in bone tissue and is involved in the regulation of the diameter of the collagen fibrils.69
Likewise, ALP is expressed very early during the biomineralization process and early during osteoblastogenesis and is continuously associated with the region of highest ossification. ALP binds to the hydroxyapatite crystals via a phosphoinositol linkage.69,70 The major non-collagenous protein in bone tissue is ON that accounts for about 2% of the total protein and is involved in the regulation of osteoblast growth and/or proliferation through controlling the diameter of the collagen fibers, as well as matrix mineralization.40ON was found to be strongly upregulated by silica in SaOS-2.
In contrast to the soluble ALP, the expression of the soluble OC that is assumed to be involved in the anabolic/catabolic bone metabolism35 is not altered during silica exposure. Also no change of gene expression is seen for BSP, an activator of migration of osteoprogenitor cells.41
These expression studies show that silica positively affects the collagenous structural scaffold formed by SaOS-2 cells, COLI and to a lower extent COLV. In addition, ALP, a serum glycosylated protein that efficiently activates calcification,71,72 very likely acts as a nucleator of apatite formation. The two additional genes induced after exposure of SaOS-2 to silica are OPN and ON. It is reasonable to assume that OPN is initiating and enhancing mineralization via its property to bind calcium and collagen.73 In addition, ON has been described to bind to collagen,40 and as a secreted protein, rich in acidic and in cysteine moieties, accelerates hydroxyapatite deposition.74
In contrast, the two bone-specific proteins, BSP and OC, show no altered steady-state-expression in SaOS-2 cells after silica exposure. It appears to be surprising that, even though BSP exhibits higher binding capacity for collagen compared to OPN,75 this sialoprotein is not under the control of silica and BMP-2. Evidence has been presented that the expression of BSP is primarily under the control of RUNX2.76 Finally, it came as no surprise that OC expression is not changed in response to silica/BMP-2 stimulus, in view of the finding that this gene is likewise under a direct control of RUNX2.77
Taken together, the data accumulated in this report extend our initial27 and the supporting data29 that silica causes morphogenetic processes via BMP-2 rather than the BMP-2-independent RUNX2 pathway.
Acknowledgements
W. E. G. M. is a holder of an ERC Advanced Investigator Grant (no. 268476 BIOSILICA) as well as of an ERC proof-of-concept grant (no. 324564; Silica-based nanobiomedical approaches for treatment of bone diseases). This work was supported by grants from the European Commission (no. 311848 “SPECIAL”, and large-scale integrating project no. FP7-KBBE-2010-4-266033 “BlueGenics”, as well as European-Chinese Research Staff Exchange Cluster MarBioTec*EU-CN* PIRSES-GA-2009-246987), the International Human Frontier Science Program, the German Bundesministerium für Bildung und Forschung – International Bureau (no. CHN 09/1AP – German-Chinese Joint Lab on Bio-Nano-Composites), the Public Welfare Project of the Ministry of Land and Resources of the People's Republic of China (grant no. 201011005-06) and the International S & T Cooperation Program of China (grant no. 2008DFA00980).
Notes and references
- T. Yin and L. Li, The stem cell niches in bone, J. Clin. Invest., 2006, 116, 1195–1201 CrossRef CAS.
- J. E. Aubin, Advances in the osteoblast lineage, Biochem. Cell Biol., 1998, 76, 899–910 CrossRef CAS.
- T. J. Martin and K. W. Ng, Mechanisms by which cells of the osteoblast lineage control osteoclast formation and activity, J. Cell. Biochem., 1994, 56, 357–366 CrossRef CAS.
- J. E. Aubin and E. Bonnelye, Osteoprotegerin and its ligand: a new paradigm for regulation of osteoclastogenesis and bone resorption, Medscape Womens Health, 2000, 5, 5 CAS.
- T. L. Burgess, Y. Qian, S. Kaufman, B. D. Ring, G. Van, C. Capparelli, M. Kelley, H. Hsu, W. J. Boyle, C. R. Dunstan, S. Hu and D. L. Lacey, The ligand for osteoprotegerin (OPGL) directly activates mature osteoclasts, J. Cell Biol., 1999, 145, 527–538 CrossRef CAS.
- A. Bernhardt, S. Thieme, H. Domaschke, A. Springer, A. Rösen-Wolff and M. Gelinsky, Crosstalk of osteoblast and osteoclast precursors on mineralized collagen – towards an in vitro model for bone remodeling, J. Biomed. Mater. Res., Part A, 2010, 95, 848–856 CrossRef CAS.
-
B. Hall, Developmental and cellular skeletal biology, Academic Press, New York, 1978 Search PubMed.
- C. T. Brighton and R. M. Hunt, Early histological and ultrastructural changes in medullary fracture callus, J. Bone Joint Surg., 1991, 73A, 832–847 Search PubMed.
- C. Comar, W. E. Lotz and G. A. Boyd, Autoradiographic studies of calcium, phosphorus and strontium distribution in the bones of the growing pig, Am. J. Anat., 1952, 90, 113–125 CrossRef CAS.
-
F. H. Netter, Musculoskeletal system: anatomy, physiology, and metabolic disorders, Ciba-Geigy Corporation, New Jersey, 1987 Search PubMed.
- G. Z. Eghbali-Fatourechi, U. I. L. Mödder, N. Charatcharoenwitthaya, A. Sanyal, A. H. Undale, J. A. Clowes, J. E. Tarara and S. Khosla, Characterization of circulating osteoblast lineage in humans, Bone, 2007, 40, 1370–1377 CrossRef CAS.
- T. Fujita, Y. Azuma, R. Fukuyama, Y. Hattori, C. Yoshida, M. Koida, K. Ogita and T. Komori, Runx2 induces osteoblast and chondrocyte differentiation and enhances their migration by coupling with PI3K-Akt signaling, J. Cell Biol., 2004, 166, 85–95 CrossRef CAS.
- I. Kalajzic, Z. Kalajzic, M. M. Hurley, A. C. Lichtler and D. W. Rowe, Stage specific inhibition of osteoblast lineage differentiation by FGF2 and noggin, J. Cell. Biochem., 2003, 88, 1168–1176 CrossRef CAS.
- H. C. Schröder, S. Perović-Ottstadt, M. Wiens, R. Batel, I. M. Müller and W. E. G. Müller, Differentiation capacity of the epithelial cells in the sponge Suberites domuncula, Cell Tissue Res., 2004, 316, 271–280 CrossRef.
- E. Gazzerro, V. Gangji and E. Canalis, Bone morphogenetic proteins induce the expression of noggin, which limits their activity in cultured rat osteoblasts, J. Clin. Invest., 1998, 102, 2106–2114 CrossRef CAS.
- B. L. Rosenzweig, T. Imamura, T. Okadome, G. N. Cox, H. Yamashita, P. ten Dijke, C. H. Heldin and K. Miyazono, Cloning and characterization of a human type II receptor for bone morphogenetic proteins, Proc. Natl. Acad. Sci. U. S. A., 1995, 92, 7632–7636 CrossRef CAS.
- J. C. Baker and R. M. Harland, From receptor to nucleus: the Smad pathway, Curr. Opin. Genet. Dev., 1997, 7, 467–473 CrossRef CAS.
- W. G. Jang, E. J. Kim, D. K. Kim, H. M. Ryoo, K. B. Lee, S. H. Kim, H. S. Choi and J. T. Koh, BMP2 protein regulates osteocalcin expression via Runx2-mediated Atf6 gene transcription, J. Biol. Chem., 2012, 287, 905–915 CrossRef CAS.
- C. Zhang, Transcriptional regulation of bone formation by the osteoblastspecific transcription factor Osx, J. Orthop. Surg. Res., 2010, 5, 37, DOI:10.1186/1749-799x-5-37.
- H. N. Kim, W. K. Min, J. H. Jeong, S. G. Kim, J. R. Kim, S. Y. Kim, J. Y. Choi and B. C. Park, Combination of Runx2 and BMP2 increases conversion of human ligamentum flavum cells into osteoblastic cells, BMB Rep., 2011, 44, 446–451 CrossRef CAS.
- D. G. Winkler, M. K. Sutherland, J. C. Geoghegan, C. Yu, T. Hayes, J. E. Skonier, D. Shpektor, M. Jonas, B. R. Kovacevich, K. Staehling-Hampton, M. Appleby, M. E. Brunkow and J. A. Latham, Osteocyte control of bone formation via sclerostin, a novel BMP antagonist, EMBO J., 2003, 22, 6267–6276 CrossRef CAS.
- E. M. Lewiecki, Sclerostin: a novel target for intervention in the treatment of osteoporosis, Discov. Med., 2011, 12, 263–273 Search PubMed.
- M. T. Drake, B. Srinivasan, U. I. Mödder, J. M. Peterson, L. K. McCready, B. L. Riggs, D. Dwyer, M. Stolina, P. Kostenuik and S. Khosla, Effects of parathyroid hormone treatment on circulating sclerostin levels in postmenopausal women, J. Clin. Endocrinol. Metab., 2010, 95, 5056–5062 CrossRef CAS.
- T. Gao, H. T. Aro, H. Ylänen and E. Vuorio, Silica-based bioactive glasses modulate expression of bone morphogenetic protein-2 mRNA in Saos-2 osteoblasts in vitro, Biomaterials, 2001, 22, 1475–1483 CrossRef CAS.
- U. Schlossmacher, M. Wiens, H. C. Schröder, X. H. Wang, K. P. Jochum and W. E. G. Müller, Silintaphin-1: interaction with silicatein during structure guiding biosilica formation, FEBS J., 2011, 278, 1145–1155 CrossRef CAS.
- M. Wiens, X. H. Wang, U. Schloßmacher, I. Lieberwirth, G. Glasser, H. Ushijima, H. C. Schröder and W. E. G. Müller, Osteogenic potential of bio-silica on human osteoblast-like (SaOS-2) cells, Calcif. Tissue Int., 2010, 87, 513–524 CrossRef CAS.
- M. Wiens, X. H. Wang, H. C. Schröder, U. Kolb, U. Schloßmacher, H. Ushijima and W. E. G. Müller, The role of biosilica in the osteoprotegerin/RANKL ratio in human osteoblast-like cells, Biomaterials, 2010, 31, 7716–7725 CrossRef CAS.
- C. A. Nelson, J. T. Warren, M. W. Wang, S. L. Teitelbaum and D. H. Fremont, RANKL Employs distinct binding modes to engage RANK and the osteoprotegerin decoy receptor, Structure, 2012, 20, 1971–1982 CrossRef CAS.
- P. Han, C. Wu and Y. Xiao, The effect of silicate ions on proliferation, osteogenic differentiation and cell signalling pathways (WNT and SHH) of bone marrow stromal cells, Biomater. Sci., 2013, 1, 379–392 RSC.
-
L. Salentijn, Biology of Mineralized Tissues: Cartilage and Bone, University College of Dental Medicine post-graduate dental lecture series, Columbia, 2007 Search PubMed.
- H. Agata, I. Asahina, Y. Yamazaki, M. Uchida, Y. Shinohara, M. J. Honda, H. Kagami and M. Ueda, Effective bone engineering with periosteum-derived cells, J. Dent. Res., 2007, 86, 79–83 CrossRef CAS.
- B. F. Boyce and L. Xing, Biology of RANK, RANKL, and osteoprotegerin, Arthritis Res. Ther., 2007, 9(Suppl 1), S1 Search PubMed.
- G. Karsenty and M. Ferron, The contribution of bone to whole-organism physiology, Nature, 2012, 481, 314–320 CrossRef CAS.
- J. B. Lian, G. S. Stein, J. L. Stein and A. J. van Wijnen, Osteocalcin gene promoter: unlocking the secrets for regulation of osteoblast growth and differentiation, J. Cell Biochem. Suppl., 1998, 30–31, 62–72 CrossRef CAS.
- P. Ducy, C. Desbois, B. Boyce, G. Pinero, B. Story, C. Dunstan, E. Smith, J. Bonadio, S. Goldstein, C. Gundberg, A. Bradley and G. Karsenty, Increased bone formation in osteocalcin-deficient mice, Nature, 1996, 382, 448–452 CrossRef CAS.
- I. J. Lorch, Alkaline phosphatase and the mechanism of ossification, J. Bone Joint Surg., 1949, 31, 94–99 Search PubMed.
- W. E. G. Müller, X. H. Wang, B. Diehl-Seifert, K. Kropf, U. Schloßmacher, I. Lieberwirth, G. Glasser, M. Wiens and H. C. Schröder, Inorganic polymeric phosphate/polyphosphate as an inducer of alkaline phosphatase and a modulator of intracellular Ca2+ level in osteoblasts (SaOS-2 cells) in vitro, Acta Biomater., 2011, 7, 2661–2671 CrossRef.
- C. Niyibizi and D. R. Eyre, Structural characteristics of cross-linking sites in type V collagen of bone. Chain specificities and heterotypic links to type I collagen, Eur. J. Biochem., 1994, 224, 943–950 CrossRef CAS.
- J. Sodek, B. Ganss and M. D. McKee, Osteopontin, Crit. Rev. Oral Biol. Med., 2000, 11, 279–303 CAS.
- J. D. Termine, H. K. Kleinman, S. W. Whitson, K. M. Conn, M. L. McGarvey and G. R. Martin, Osteonectin, a bone-specific protein linking mineral to collagen, Cell, 1981, 26, 99–105 CrossRef CAS.
- A. Karadag and L. W. Fisher, Bone sialoprotein enhances migration of bone marrow stromal cells through matrices by bridging MMP-2 to alpha(v)beta3-integrin, J. Bone Miner. Res., 2006, 21, 1627–1636 CrossRef CAS.
- J. Silva-Correia, V. Miranda-Gonçalves, A. J. Salgado, N. Sousa, J. M. Oliveira, R. M. Reis and R. L. Reis, Angiogenic potential of gellan-gum-based hydrogels for application in nucleus pulposus regeneration: in vivo study, Tissue Eng., Part A, 2012, 18, 1203–1212 CrossRef CAS.
- X. H. Wang, H. C. Schröder, B. Diehl-Seifert, K. Kropf, U. Schloßmacher, M. Wiens and W. E. G. Müller, Dual effect of inorganic polymeric phosphate/polyphosphate on osteoblasts and osteoclasts in vitro, J. Tissue Eng. Regen. Med., 2012 DOI:10.1002/term.1465.
- J. Fogh, J. M. Fogh and T. Orfeo, One hundred and twenty-seven cultured human tumor cell lines producing tumors in nude mice, J. Natl. Cancer Inst., 1977, 59, 221–226 CAS.
- H. C. Schröder, X. H. Wang, M. Wiens, B. Diehl-Seifert, K. Kropf, U. Schloßmacher and W. E. G. Müller, Silicate modulates the cross-talk between osteoblasts (SaOS-2) and osteoclasts (RAW 264.7 cells): inhibition of osteoclast growth and differentiation, J. Cell. Biochem., 2012, 113, 3197–3206 CrossRef.
- S. L. Cheng, J. W. Yang, L. Rifas, S. F. Zhang and L. V. Avioli, Differentiation of human bone marrow osteogenic stromal cells in vitro: induction of the osteoblast phenotype by dexamethasone, Endocrinology, 1994, 134, 277–286 CrossRef CAS.
- H. Yamaoka, H. Asato, T. Ogasawara, S. Nishizawa, T. Takahashi, T. Nakatsuka, I. Koshima, K. Nakamura, H. Kawaguchi, U. I. Chung, T. Takato and K. Hoshi, Cartilage tissue engineering using human auricular chondrocytes embedded in different hydrogel materials, J. Biomed. Mater. Res., Part A, 2006, 78, 1–11 CrossRef.
-
R. I. Freshney, Specialized cells, in Culture of Animal Cells: A Manual of Basic Technique, ed. R. I. Freshney, Wiley-Liss, New York, 4th edn, 2000, pp. 367–370 Search PubMed.
- V. L. Workman, S. B. Dunnett, P. Kille and D. D. Palmer, Microfluidic chip-based synthesis of alginate microspheres for encapsulation of immortalized human cells, Biomicrofluidics, 2007, 1, 014105, DOI:10.1063/1.2431860.
- W. E. G. Müller, X. H. Wang, H. C. Schröder, M. Korzhev, V. A. Grebenjuk, J. S. Markl, K. P. Jochum, D. Pisignano and M. Wiens, A cryptochrome-based photosensory system in the siliceous sponge Suberites domuncula (Demospongiae), FEBS J., 2010, 277, 1182–1201 CrossRef.
- M. W. Pfaffl, A new mathematical model for relative quantification in real-time RT-PCR, Nucleic Acids Res., 2001, 29, 2002–2007 CrossRef.
- F. Langenbach, K. Berr, C. Naujoks, A. Hassel, M. Hentschel, R. Depprich, N. R. Kubler, U. Meyer, H. P. Wiesmann, G. Kögler and J. Handschel, Generation and differentiation of microtissues from multipotent precursor cells for use in tissue engineering, Nat. Protoc., 2011, 6, 1726–1735 CrossRef CAS.
- U. Schloßmacher, H. C. Schröder, X. H. Wang, Q. Feng, B. Diehl-Seifert, S. Neumann, A. Trautwein and W. E. G. Müller, Alginate/silica composite hydrogel as a potential morphogenetically active scaffold for three-dimensional tissue engineering, RSC Adv., 2013 10.1039/c3ra23341c , in press.
-
A. Martens, Handbuch der Materialienkunde für den Maschinenbau, Springer, Berlin, 1898, p. 234 Search PubMed.
-
L. Sachs, Angewandte Statistik, Springer, Berlin, 1984, p. 242 Search PubMed.
- T. Link, X. H. Wang, U. Schloßmacher, Q. L. Feng, H. C. Schröder and W. E. G. Müller, An approach to a biomimetic bone scaffold: increased expression of BMP-2 and of osteoprotegerin in SaOS-2 cells grown onto silica-biologized 3D printed scaffolds, RSC Adv. 10.1039/C2RA22352J , in press.
- R. F. Cox, A. Jenkinson, K. Pohl, F. J. O'Brien and M. P. Morgan, Osteomimicry of mammary adenocarcinoma cells in vitro; increased expression of bone matrix proteins and proliferation within a 3D collagen environment, PLoS One, 2012, 7, e41679, DOI:10.1371/journal.pone.0041679.
- S. B. Rodan, Y. Imai, M. A. Thiede, G. Wesolowski, D. Thompson, Z. Bar-Shavit, S. Shull, K. Mann and G. A. Rodan, Characterization of a human osteosarcoma cell line (Saos-2) with osteoblastic properties, Cancer Res., 1987, 47, 4961–4966 CAS.
- H. C. Anderson, H. H. T. Hsu, P. Raval, P. R. Reynold, D. J. Gurley, M. X. Aguilera, L. S. Davis and P. E. Moylan, The bone-inducing agent in Saos-2 cell extracts and secretions, Cells Mater., 1998, 8, 89–98 Search PubMed.
- X. Li, H. Gao, M. Uo, Y. Sato, T. Akasaka, S. Abe, Q. Feng, F. Cui and F. Watari, Maturation of osteoblast-like SaoS2 induced by carbon nanotubes, Biomed. Mater., 2009, 4, 015005, DOI:10.1088/1748-6041/4/1/015005.
- C. Pautke, M. Schieker, T. Tischer, A. Kolk, P. Neth, W. Mutschler and S. Milz, Characterization of osteosarcoma cell lines MG-63, Saos-2 and U-2 OS in comparison to human osteoblasts, Anticancer Res., 2004, 24, 3743–3748 CAS.
- K. Bertaux, O. Broux, C. Chauveau, P. Hardouin, J. Jeanfils and J. C. Devedjian, Runx2 regulates the expression of GNAS on SaOs-2 cells, Bone, 2006, 38, 943–950 CrossRef CAS.
- M. Stock, H. Schafer, M. Fliegauf and F. Otto, Identification of novel genes of the bone-specific transcription factor Runx2, J. Bone Miner. Res., 2004, 19, 959–972 CrossRef CAS.
- F. Shapiro, Bone development and its relation to fracture repair. The role of mesenchymal osteoblasts and surface osteoblasts, Eur. Cell Mater., 2008, 15, 53–76 CAS.
- S. Weiner and H. D. Wagner, The material bone: structure-mechanical function relations, Ann. Rev. Mater. Sci., 1998, 28, 271–298 CrossRef CAS.
-
E. F. Eriksen, D. W. Axelrod and F. Melsen, Bone Histomorphometry, Raven Press, New York, 1994, pp. 1–12 Search PubMed.
- W. J. Landis, F. H. Silver and J. W. Freeman, Collagen as a scaffold for biomimetic mineralization of vertebrate tissues, J. Mater. Chem., 2006, 16, 1495–1503 RSC.
- R. Z. Kramer, M. G. Venugopal, J. Bella, P. Mayville, B. Brodsky and H. M. Berman, Staggered molecular arrangement of a collagen-like peptide with a single charged pair, J. Mol. Biol., 2000, 301, 1191–1205 CrossRef CAS.
- B. Clarke, Normal bone anatomy and physiology, Clin. J. Am. Soc. Nephrol., 2008, 3, S131–S139 CrossRef CAS.
- M. P. Whyte, Hypophosphatasia and the role of alkaline phosphatase in skeletal mineralization, Endocr. Rev., 1994, 15, 439–461 CAS.
-
M. P. Whyte, Hypophosphatasia, in The Metabolic and Molecular Bases of Inherited Diseases, ed. C. R. Scriver, A. L. Beaudet, W. S. Sly, D. Valle, B. Childs, K. W. Kinzler and B. Vogelstein, McGraw-Hill Inc., New York, 2001, pp. 5313–5329 Search PubMed.
- P. A. Price, D. Toroian and W. S. Chan, Tissue-nonspecific alkaline phosphatase is required for the calcification of collagen in serum: a possible mechanism for biomineralization, J. Biol. Chem., 2009, 284, 4594–4604 CrossRef CAS.
- Y. Chen, B. S. Bal and J. P. Gorski, Calcium and collagen binding properties of osteopontin, bone sialoprotein, and bone acidic glycoprotein-75 from bone, J. Biol. Chem., 1992, 267, 24871–24878 CAS.
- K. Ishizeki, T. Kagiya, N. Fujiwara, K. Otsu and H. Harada, Expression of osteogenic proteins during the intrasplenic transplantation of Meckel's chondrocytes: a histochemical and immunohistochemical study, Arch. Histol. Cytol., 2009, 72, 1–12 CrossRef CAS.
- K. M. Zurick, C. Qin and M. T. Bernards, Mineralization induction effects of osteopontin, bone sialoprotein, and dentin phosphoprotein on a biomimetic collagen substrate, J. Biomed. Mater. Res., Part A, 2012 DOI:10.1002/jbm.a.34462.
- M. Takagi, N. Kamiya, T. Takahashi, S. Ito, M. Hasegawa, N. Suzuki and K. Nakanishi, Effects of bone morphogenetic protein-2 and transforming growth factor beta1 on gene expression of transcription factors, AJ18 and Runx2 in cultured osteoblastic cells, J. Mol. Histol., 2004, 35, 81–90 CrossRef CAS.
- G. Zhou, Q. Zheng, F. Engin, E. Munivez, Y. Chen, E. Sebald, D. Krakow and B. Lee, Dominance of SOX9 function over RUNX2 during skeletogenesis, Proc. Natl. Acad. Sci. U. S. A., 2006, 103, 19004–19009 CrossRef CAS.
|
This journal is © The Royal Society of Chemistry 2013 |
Click here to see how this site uses Cookies. View our privacy policy here.