DOI:
10.1039/C3BM00161J
(Paper)
Biomater. Sci., 2013,
1, 537-544
Concentration dependent neural differentiation and neurite extension of mouse ESC on primary amine-derivatized surfaces
Received
29th October 2012
, Accepted 15th February 2013
First published on 27th February 2013
Abstract
Cell sourcing continues to be a significant limitation to regenerative medicine especially in neural lineages where population heterogeneity during in vitro culture prevents definitive phenotype assessment. For nearly 40 years, the biological community has worked with amine-derivated surfaces and hydrogels, especially alginate, with little quantitative assessment of how local amine concentration influences the extent of neural differentiation and neurite extension. In this manuscript we show that the local concentration of amines distinctly influences mouse embryonic stem cell (ESC) lineage commitment and the length of neurite extensions both of which are early indicators of differentiation. The well-defined amine gradients are a highly relevant tool for identifying these critical concentrations and thresholds. We feel these results will be of critical importance to researchers developing new ex vivo culture materials for neural applications as well as the community exploring nerve regeneration in vivo.
Introduction
Spinal cord injuries which lead to persistent neurological deficits represent a major burden for the United States (US) health care system at nearly $9.73 billion dollars a year.1 With stable incident rates2 and increasing life expectancy,3 costs will continue to increase unless novel treatments which restore neurological function are developed. Restoration of neurological function requires axonal extension to the original target and restoration of contact between the axon termini and target. Although this happens regularly in the peripheral nervous system, it rarely occurs in the central nervous system (CNS) due to differences in microenvironment.4 To overcome this lack of regeneration in the CNS, human clinical trials for spinal cord injury utilizing embryonic stem cell (ESC) injections have been conducted.5 ESC or induced pluripotency stem cells are the likely clinically relevant cell source for CNS injury repair. However, significant knowledge gaps must be addressed with regard to safety, signaling and heterogeneity within the differentiated progenitor cell populations to ensure tumorigenicity within the body is limited, lineage commitment to the desired phenotypes is maintained and facilitate survival, engraftment and maturation within the injured spinal cord regime.6–9
Initial studies have shown that soluble protein signaling factors and surface chemistry systems have a profound effect on lineage commitment and cellular function.10–13 Of these model systems, functionalized amine surfaces have been most commonly studied10,14–16 due to the long standing use of primary amine-coated surfaces, such as poly-lysine, to promote neural cell culture.17 Collectively, primary amine groups have been found to promote stem cell viability, migration and neurite extension compared to other surface chemistries.10,12 However, the influence of amine concentration has not been examined widely even though the spatial presentation of chemical functionality is largely acknowledged to be important in surface mediated integrin signaling and strongly influences the cell behavior.18–21 In this study, we examine the effects of amine concentration on mouse ESC neural differentiation, proliferation, and neurite extension.
To maximize information obtained from each study while reducing the time and resources necessary to examine media conditions and surface concentrations that are difficult to characterize individually,22–24 combinatorial concentration gradient methods were utilized. Although several methods to fabricate haptotactic molecular gradients have been published,12,18,25,26 each has shown one or more limitations regarding the substrate, dimensionality, reproducibility and functional group versatility.27 In this study, we present the development of a novel “vacuum away” channel confinement vapor deposition method (Fig. 1A), which overcomes challenges with regard to chemical tunability25,28 concentration control spatially12 and has potential for translation into complicated geometries that are present in the aforementioned gradient formation methods.
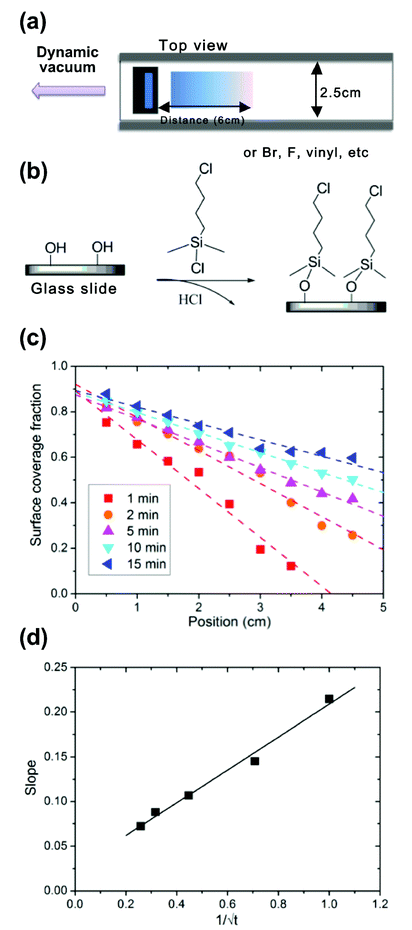 |
| Fig. 1 (a) The fabrication scheme for generating chemical concentration gradients of self assembled monolayers using a confined channel. (b) 4-chlorobutyldimethylchlorosilane was used as chemical source and may be deposited on silicon or glass. The profile data were based on measurements from three samples prepared under identical conditions. (c) The relative concentration of chlorine molecules along the substrate and profiles was varied precisely by deposition time (theoretical prediction depicted by dotted lines). (d) There is a linear correlation (solid line) between the slope of the gradient and the diffusion time, which is in perfect agreement with the theoretical description. Static water contact angles were measured for each step of the substrate fabrication. | |
Materials and methods
Materials
4-Chlorobutyldimethylchlorosilane and 7-octenyldimethylchlorosilane were purchased from Gelest. Texas Red®-Succinimidyl Ester, (Mixed Isomers) was purchased from Invitrogen. All other reagents were purchased from Sigma-Aldrich and used as received unless specifically noted in the biological sections.
Chlorine-terminated SAM gradient fabrication
All substrates were pre-treated with ultraviolet–ozone exposure (UVO, Jelight Company Inc. Model No. 42A) for 10 min to remove organic contaminants from the surface. The substrates were washed with methanol and toluene 3 times, and blown dry with nitrogen prior to use. The chlorosilane reservoir (1.5 cm × 2.5 cm × 1.1 cm) and substrate support (7.5 cm × 2.5 cm × 1.0 cm) for the glass coverslips were fabricated from Teflon. Both the reservoir and substrate support, which controls the channel height, were inserted into rectangular glass tubing (30 cm × 2.5 cm × 1.3 cm) and placed in a sealed metal chamber. The dynamic vacuum was pulled from the side nearest the reservoir and away from the substrate. Methanol was injected through a syringe port to quench the diffusion process following defined time intervals. Following the vapor deposition, the substrates were removed from the chamber, washed successively with methanol, toluene, methanol, and blown dry under N2. The samples were stored in vacuum desiccators at room temperature until used. Samples are stable for approximately 6–8 weeks.
Amine-concentration gradient fabrication
4-Chlorobutyldimethylchlorosilane (150 μL, neat in reservoir) was deposited on clean glass substrates using a 2 min diffusion time to yield a chlorine concentration gradient surface. Two subsequent chemical reactions were carried out immediately following the gradient profile formation. The first is an SN2 substitution of the Cl end group with NaN3 and further reduction of azide with PPh3/H2O. For the SN2 substitution, Cl gradient substrates were incubated into NaN3 (0.2 g, 61 mM)/DMF (HPLC grade, 50 mL) solution, and small amount of 18-crown-6 was also added as a phase-transfer catalyst. The reaction was carried out at 65 °C for 2 days. After 2 days, the slides were removed, washed with methanol, toluene, and methanol and blown dry under N2. The azide reaction was followed by immersing these slides into PPh3 (1.3 g, 5 mM)/H2O (90 μL, 5 mM)/THF (50 mL) solution, stirring at ambient temperature overnight.
Contact angle measurements
The advancing contact angles were measured using an Advanced Goniometer (Ramé-Hart Instrument Co., Model 500) at 25 °C using ultrapure water (2 μL, 18 M cm−1) as the probe fluid and analyzed by a drop shape analysis method (ImageJ, National Institute of Health, Bethesda, MD, USA, download available at http://rsb.info.nih.gov/ij/). The surface coverage fractions were calculated based on eqn (1),29 which is suitable for the heterogeneous monolayers when the size of patches approach molecular dimensions. | (1 + cos θ)2 = f1(1 + cos θ1)2 + f2(1 + cos θ2)2 (f1 + f2 = 1) | (1) |
The standard uncertainty of contact angle measurements at each point was determined by the standard deviation between three independent measurements on three samples prepared under identical conditions at each position noted along the gradient.
XPS measurements were performed on a Kratos AXIS Ultra DLD spectrometer using silicon wafer as substrates. The X-ray source was monochromated Al Kα, scanning over a binding-energy range of 0 to 1200 eV with a dwell time of 100 ms. The analyzer pass energy was 160 eV for the survey spectra and 20 eV for the high-resolution C1s, N1s, Cl2s and O1s scans. Each spectrum was collected over a 300 μm × 700 μm sample area. Peak area was analyzed and atomistic concentrations were calculated with CasaXPS software using a Levenberg–Marquardt algorithm assuming a linear background.
Fluorescence staining and microscopy
Texas Red Succinimidyl Ester (5 mg) was dissolved in 500 μL of DMSO (biotech grade) to form a stock solution and then diluted 100 times with PBS buffer immediately prior to each use. Three drops of diluted dye solution (50 μL) were dispensed onto the amine surface, covered with a clean slide and incubated at 37 °C, 100% humidity for 2 h. Gradients were then washed with water and methanol, and blown dry under N2. The fluorescence intensity was viewed with an IX81 microscope (Olympus) with mercury bulb excitation and the appropriate filters. Displayed images were taken sequentially with the same light intensity, exposure time and magnification.
D3 mouse embryonic stem cell culture and seeding
D3 mice ESC30 were cultured on 0.1% gelatin-coated tissue culture flasks in ESC media (DMEM supplemented with 10% FBS, 10−4 M β-mercaptoethanol, 0.224 μg mL−1L-glutamine, 1.33 μg mL−1 HEPES, and 1000 units per mL human recombinant LIF). 500
000 cells were seeded evenly along each gradient in neural media (80% F-12/20% Neurobasal media with N2 and B27 supplements, 10 mM sodium pyruvate and 1 μM retinoic acid). The media was changed every other day for the duration of the experiment.
Immunofluorescence and alkaline phosphatase quantification
Cells cultured on gradient substrates were fixed at designated time points with 4% paraformaldehyde/PBS, washed, and stored at 4 °C in PBS. Nonspecific antibody binding was blocked by incubating in 10% goat serum, then the gradients were exposed to TUJ1 (PRB-435p, Covance, 1
:
500) and Sox 3 (sc17324, Santa Cruz, 1
:
50) antibody, followed by appropriate secondary antibodies conjugated with Alexaflour 488 or Alexaflour 544 (Invitrogen). DAPI was used to stain the cell nuclei. Images were taken with an automated IX81 microscope (Olympus) every 5 mm along the length of the gradient. Cellular density at each position was determined by using the automated counting function of ImageJ to count nuclei in images at each position from at least 5 separate samples. Statistical averages of neurite lengths were made from measurements of 200 cells from at least 5 separate samples. Quantification of alkaline phosphatase was determined with a SensoLyte pNNP Alkaline Phosphatase assay kit (AnaSpec, Fremont, CA) according to the manufacturers protocol. For normalization, total protein was measured in samples with a Dc Protein assay (Biorad, Hercules, CA) according to the manufacturer's protocol.
Statistics
All experiments were conducted at least 3 times (n ≥ 3) with multiple substrates as noted in each section. All quantitative data are presented as the average ± standard deviation. One-way analysis of variance (ANOVA) with Tukey post hoc analysis was performed where applicable. Significance was set at a p-value of less than 0.05.
Results and discussion
Under Fickian diffusion principles,31 the vapor concentration should have a linear relationship as a function of distance and can be tuned precisely by varying the deposition time over short distances relative to the solvent reservoir. We generated a series of linear concentration gradients to demonstrate both the concentration control and chemical tunability of the “vacuum away” method (Fig. 1B). Due to the hydrophobicity of 4-chlorobutyldimethylchlorosilane, a surface energy gradient (Fig. 1C) is generated linearly with the concentration profile. The contact angle data correlated well with theoretical calculations (Fig. 1B and C). The slope of the gradient had a linear correlation with 1/√t (Fig. 1D), as predicted by Fick's diffusion laws. Designed gradient profiles can be calculated, easily achieved and well controlled by deposition time.
The concentration profile and functionality were verified using high resolution Cl2s X-ray photoelectron spectroscopy (XPS) scans (Fig. 2A). The number of atoms/test area decreased linearly with position along the substrate (Fig. 2B), matching the linear results obtained from contact angle and surface energy measurements (Fig. 2C). A two-step chemical derivatization (Fig. 3A) process was used to achieve the amine-terminated surface concentration gradient. The chlorine group was efficiently displaced via SN2 reaction by an azide salt and subsequently reduced to a primary amine. The atomistic conversions were verified by succinimidyl ester conjugated Texas Red (which reacts specifically with amines yielding a fluorescent gradient) (Fig. 3B) and XPS (Fig. 3C, D). High resolutions XPS scans show the quantitative loss of the Cl2p signal and acquisition of the N1s peaks following the azide reaction and the further transition to a single peak which corresponds to the primary amine following the reduction step. High fidelity and efficiency is critical in our fabrication processes as quantitatively probing biological hypotheses often requires the generation of >100 identical substrates to engage multiple time points and controls.
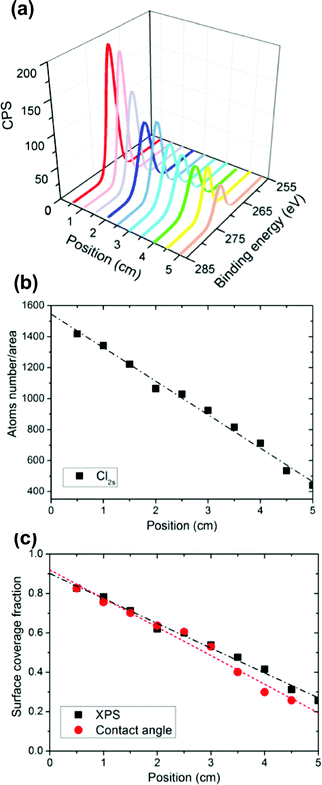 |
| Fig. 2 The chlorine SAM gradient was deposited on a UV-ozone treated silicon (100) surface (75 mm × 25 mm) using a 2 min deposition time. Molecular concentrations along the substrate were verified by high-resolution XPS Cl2s measurements. (a) Quantitative atom number concentrations were captured by peak area/relative sensitivity factor (RSF) (single measurement for each position). (b) The component fractions were calculated by comparing the peak area with the universal close-packed chlorine Cl2s signal, which were consistent with contact angles results as shown (c). | |
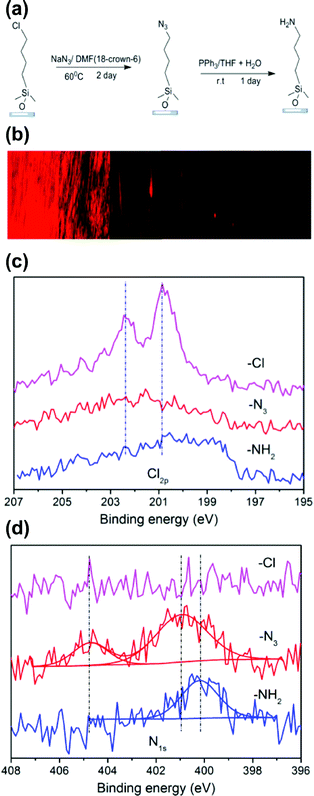 |
| Fig. 3 (a) Chlorine-terminated SAMs are converted easily to azides using an SN2 mechanism and further reduced quantitatively to an amine under mild conditions. (b) Fluorescence images for an amine concentration gradient surface that was derivatized with Texas Red afforded visualization of the amine-concentration profile on the gradient surface. (c) High resolution N1s and Cl2s spectra from XPS verified the quantitative conversion of the individual reactions. (d) High resolution N1s spectra show the stepwise transition from Cl to N3 to NH2 functional groups. | |
ESCs have two principal characteristics, pluripotency and the ability to self-renew.32 Both must be monitored in order to ensure efficacy and safety when ESC derived cells are used as a cell source for neural tissue engineering. Loss of pluripotency was evident after 1 day of neural differentiation on the amine gradients with ESC at all concentrations as measured by a significant drop in alkaline phosphatase (ALP) content relative to starting ESC population (Fig. 4A). However, ESC cultured on the 140 pmol cm−2 amine concentration region maintained higher ALP content relative to ESC on other gradient regions after 1 day of neural differentiation.
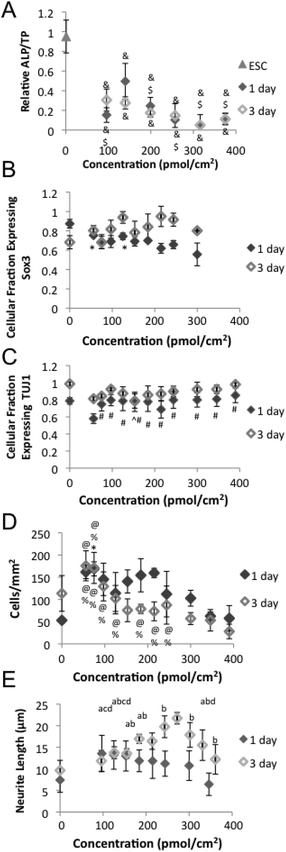 |
| Fig. 4 Mouse embryonic stem cell (ESC) response to neural differentiation on amine surface gradient. After 1 day of differentiation, (A) Alkaline phosphatase content and (B) Expression of early neural markers Sox3. (C) Expression of early neural markers TUJ1. (D) Cell number and (E) quantification of neurite length. & indicates p-value less than 0.01 relative to ESC. ¢ indicates a p-value less than 0.05 relative to the 0 p mol cm−2. $ indicates p-value less than 0.05 relative to 140 pmol cm−2. # indicates p-value less than 0.05 relative to 56 pmol cm−2. ^ indicates p-value less than 0.05 relative to 154 pmol cm−2. $ indicates p-value less than 0.05 relative to 300 pmol cm−2. ^ indicates p-value less than 0.05 relative to 154 pmol cm−2. % indicates p-value less than 0.05 relative to 346 pmol cm−2. @ indicates p-value less than 0.05 relative to 390 pmol cm−2. a indicates p-value less than 0.05 relative to 243 pmol cm−2. b indicates p-value less than 0.05 relative to 270 pmol cm−2. c indicates p-value less than 0.05 relative to 302 pmol cm−2. d indicates p-value less than 0.05 relative to 184 pmol cm−2. | |
Signaling molecule gradients play a pivotal role in the development of neural development.33–35 As such, neural differentiation is greatly affected by epigenetic factors such as media and substrate, which creates high variability in efficiency between protocols and laboratories.36–39 Expression of neurofilament protein in a polar distribution in formatting neurites has been reported in as little as 24 h.37 One ESC direct differentiation protocol has reported results as high as 48% of cells expressing neural differentiation markers at 24 h and 89% at 72 h.36 A higher fraction of ESCs cultured on lower amine concentration regions expressed Sox3, a marker of early neural precursors, compared to ESC cultured on higher amine concentrations (Fig. 4B and 5A). While a lower fraction of ESC cultured on lower amine concentration regions expressed class III beta tubulin (TUJ1), a marker for immature neurons, compared to ESC cultured on higher amine concentrations (Fig. 4C and 5B). Morphological changes accompany the differentiation and are evident after 1 day of differentiation (Fig. 5A), but up to 5 days of differentiation may be necessary to achieve overt neural morphology.39 Together this data indicates that ESC cultured on higher amine concentrations lose pluripotency and differentiate to neuronal phenotypes more quickly than ESC cultured on lower amine concentrations. Previous experiments demonstrated that negatively charged proteins preferentially absorb to amine surfaces.40 The relative surface concentration of positively charged amines is likely to create a significantly different microenvironment spatially due to variations in protein absorption, epitope presentation and potentially metabolite sequestration along the gradient which lead to the respective differences in cellular differentiation. For example, retinoic acid (RA), a key regulator of many genes involved in neural differentiation and neurite extension41 that can inhibit proliferation and viability at high concentrations,42 is a protein whose absorption my vary due to its negative charge.
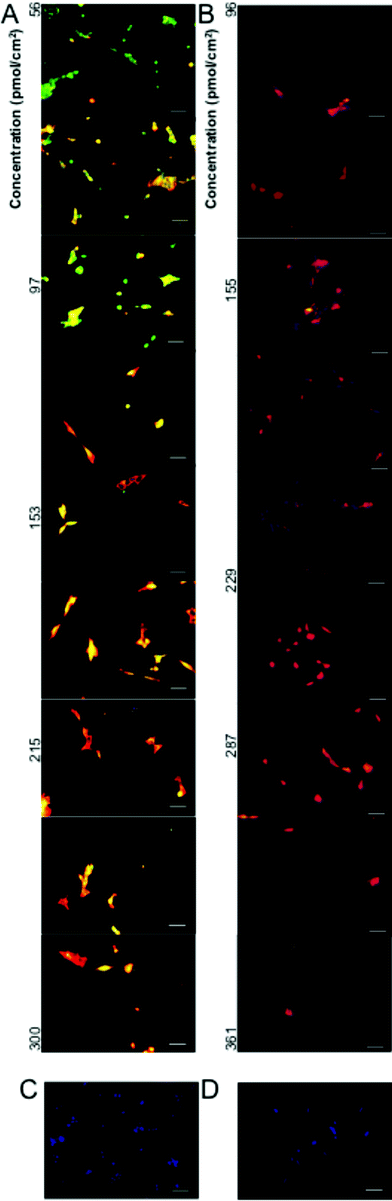 |
| Fig. 5 Immunoflourescent staining of neural markers Sox3 (Green) and TUJ1 (Red) with nuclear staining (Blue) after (A) 1 day and (B) 3 days of neural differentiation. Scale bar = 50 μm. Control staining without exposure to primary antibodies after (C) 1 day and (D) 3 days of neural differentiation. Scale bar = 50 μm. | |
Ionic interactions between the amine surface and negatively charged cell surface proteoglycans can promote cellular adhesion and spreading.43 Previous studies have indicated that higher amine content increased cellular attachment.14,26 However, higher numbers of ESC remained on lower amine concentrations regions compared to higher amine regions after 3 days of differentiation (Fig. 4D). Increased alkyl amine hydrophobicity, which correlates with increased concentration in our system (Fig. 2), can inhibit cellular proliferation.44 Previous studies altered the amine concentration by changing molecular chain length,26 and the organosilane SAM base.14 Both changes distinctly alter the resulting hydrophobicity44 and molecular structure,45,46 which has been shown to alter the effect of amines on proliferation. These changes may account for the variation in results in the studies.
Increased amine concentration and hydrophobicity were both reported to increase neurite length in previous studies.14,47 However, after 3 days of differentiation we found intermediate amine concentrations (∼270 pmol cm−2) yield the longest neurites (Fig. 4E, F) similar to a previous soluble polyamine study.48 The difference in results between studies could be due to the examined amine concentration range, the density gradient of SAMs used, cell density effects, or absorbed protein concentrations.16,47 Neural cells are able to sense surface bound protein and peptide gradients and alter neurite extension and direction in response.49–52 As neurite extension is competitive even at short lengths,53 the variable concentration gradient nature of our samples could contribute to our results, but is likely minimal due to the short duration of our cell studies, unrestricted neurite growth spatially, and a lack of observed neurite alignment at any gradient position.
Conclusions
In summary, we demonstrate a versatile, well controlled, and highly reproducible surface density gradient fabrication strategy that allows for precise control over surface functionality and slope. Our ESC differentiation results illustrate how amine concentration affects ESC function and provides new information on the optimal therapeutic range of amines on ESC neural differentiation while providing a novel platform to study the variations affecting neural differentiation that warrant further investigation. Future studies will translate these findings to clinically relevant substrates of lower modulus.
Author contributions
YM, LAS and MLB devised the experiments. LAS, YM and CSM performed the experiments. YM, LAS, CMS and MLB analyzed the data. YM, LAS and MLB wrote the paper.
Competing financial interests
The authors declare no competing financial interests.
Acknowledgements
The authors would like to thank Professor K. Sue O'Shea at the University of Michigan for her gift of the D3 cells. This work was supported through a grant from the National Science Foundation (DMR-1105329).
References
-
M. Berkowitz, et al., Spinal Cord Injury: An Analysis of Medical and Social Costs, Demos Medical Publishing Inc., New York, NY, 1998 Search PubMed.
- M. Wyndaele and J. J. Wyndaele, Incidence, prevalence and epidemiology of spinal cord injury: what learns a worldwide literature survey?, Spinal Cord, 2006, 44(9), 523–529 CrossRef CAS PubMed.
- M. A. McColl,
et al., Expectations of life and health among spinal cord injured adults, Spinal Cord, 1997, 35(12), 818–828 CAS.
- K. E. Rhodes and J. W. Fawcett, Chondroitin sulphate proteoglycans: preventing plasticity or protecting the CNS?, J. Anat., 2004, 204(1), 33–48 CrossRef CAS PubMed.
-
, World's first clinical trial of human embryonic stem cell therapy cleared, Regen. Med., 2009, 4(2), 161–161 Search PubMed.
- Q.-L. Cao,
et al., Differentiation of engrafted neuronal-restricted precursor cells is inhibited in the traumatically injured spinal cord, Exp. Neurol., 2002, 177(2), 349–359 CrossRef CAS PubMed.
- S. Karimi-Abdolrezaee,
et al., Delayed transplantation of adult neural precursor cells promotes remyelination and functional neurological recovery after spinal cord injury, J. Neurosci., 2006, 26(13), 3377–3389 CrossRef CAS PubMed.
- M. Nakamura,
et al., Transplantation of embryonic spinal cord-derived neurospheres support growth of supraspinal projections and functional recovery after spinal cord injury in the neonatal rat, J. Neurosci. Res., 2005, 81(4), 457–468 CrossRef CAS PubMed.
- D. G. Halme and D. A. Kessler, FDA regulation of stem-cell-based therapies, New Engl. J. Med., 2006, 355(16), 1730–1735 CrossRef CAS PubMed.
- Y.-J. Ren,
et al., In vitro behavior of neural stem cells in response to different chemical functional groups, Biomaterials, 2009, 30(6), 1036–1044 CrossRef CAS PubMed.
- A. K. Goetz,
et al., Temporally restricted substrate interactions direct fate and specification of neural precursors derived from embryonic stem cells, Proc. Natl. Acad. Sci. U. S. A., 2006, 103(29), 11063–11068 CrossRef CAS PubMed.
- J. N. L. Albert,
et al., Generation of monolayer gradients in surface energy and surface chemistry for block copolymer thin film studies, ACS Nano, 2009, 3(12), 3977–3986 CrossRef CAS PubMed.
- J. M. Curran, R. Chen and J. A. Hunt, The guidance of human mesenchymal stem cell differentiation in vitro by controlled modifications to the cell substrate, Biomaterials, 2006, 27(27), 4783–4793 CrossRef CAS PubMed.
- D. A. Stenger,
et al., Surface determinants of neuronal survival and growth on self-assembled monolayers in culture, Brain Res., 1993, 630(1–2), 136–147 CrossRef CAS PubMed.
- D. Kleinfeld, K. Kahler and P. Hockberger, Controlled outgrowth of dissociated neurons on patterned substrates, J. Neurosci., 1988, 8(11), 4098–4120 CAS.
- G. Lamour,
et al., Influence of surface energy distribution on neuritogenesis, Colloids Surf., B, 2009, 72(2), 208–218 CrossRef CAS PubMed.
- E. Yavin and Z. Yavin, Attachment and culture of dissociated cells from rat embryo cerebral hemispheres on polylysine-coated surface, J. Cell Biol., 1974, 62(2), 540–546 CrossRef CAS PubMed.
- N. D. Gallant,
et al., Universal gradient substrates for “click” biofunctionalization, Adv. Mater., 2007, 19(7), 965–969 CrossRef CAS.
- N. M. Moore,
et al., Synergistic enhancement of human bone marrow stromal cell proliferation and osteogenic differentiation on BMP-2-derived and RGD peptide concentration gradients, Acta Biomater., 2011, 7(5), 2091–2100 CrossRef CAS PubMed.
- A. P. Acharya,
et al., The modulation of dendritic cell integrin binding and activation by RGD-peptide density gradient substrates, Biomaterials, 2010, 31(29), 7444–7454 CrossRef CAS PubMed.
- N. M. Moore,
et al., The use of immobilized osteogenic growth peptide on gradient substrates synthesized via click chemistry to enhance MC3T3-E1 osteoblast proliferation, Biomaterials, 2010, 31(7), 1604–1611 CrossRef CAS PubMed.
- K. Rajan, Combinatorial materials sciences: experimental strategies for accelerated knowledge discovery, Annu. Rev. Mater. Res., 2008, 38(1), 299–322 CrossRef CAS.
- J. Sobek,
et al., Microarray technology as a universal tool for high-throughput analysis of biological systems, Comb. Chem. High Throughput Screen., 2006, 9(5), 365–380 CrossRef CAS PubMed.
- M. Yliperttula,
et al., High-throughput screening of cell responses to biomaterials, Eur. J. Pharm. Sci., 2008, 35(3), 151–160 CrossRef CAS PubMed.
- M. K. Chaudhury and G. M. Whitesides, How to make water run uphill, Science, 1992, 256(5063), 1539–1541 CAS.
- B. Liedberg and P. Tengvall, Molecular gradients of omega-substituted alkanethiols on gold: preparation and characterization, Langmuir, 1995, 11(10), 3821–3827 CrossRef CAS.
- J. Genzer and R. R. Bhat, Surface-bound soft matter gradients, Langmuir, 2008, 24(6), 2294–2317 CrossRef CAS PubMed.
- J. Genzer, K. Efimenko and D. A. Fischer, Formation mechanisms and properties of semifluorinated molecular gradients on silica surfaces, Langmuir, 2006, 22(20), 8532–8541 CrossRef CAS PubMed.
- J. N. Israelachvili and M. L. Gee, Contact angles on chemically heterogeneous surfaces, Langmuir, 1989, 5(1), 288–289 CrossRef CAS.
- T. Doetschman,
et al., The in vitro development of blastocyst-derived embryonic stem cell lines: formation of visceral yolk sac, blood island and myocardium, J. Embryol. Exp. Morphol., 1985, 87, 27–45 CAS.
-
J. Crank, Methods of solution when the diffusion coefficient is constant, The Mathematics of Diffusion, Oxford University Press, 1975, ch. 2, P11–P27 Search PubMed.
- J. A. Thomson,
et al., Embryonic stem cell lines derived from human blastocysts, Science, 1998, 282(5391), 1145–1147 CrossRef CAS PubMed.
- J. G. Flanagan, Neural map specification by gradients, Curr. Opin. Neurobiol., 2006, 16(1), 59–66 CrossRef CAS PubMed.
- G. Begemann and A. Meyer, Hindbrain patterning revisited: timing and effects of retinoic acid signalling, BioEssays, 2001, 23(11), 981–986 CrossRef CAS PubMed.
- P. A. Georgala, C. B. Carr and D. J. Price, The role of Pax6 in forebrain development, Dev. Neurobiol., 2011, 71(8), 690–709 CrossRef CAS PubMed.
- T. E. Gratsch and K. S. O'Shea, Noggin and chordin have distinct activities in promoting lineage commitment of mouse embryonic stem (ES) cells, Dev. Biol., 2002, 245(1), 83–94 CrossRef CAS PubMed.
-
K. S. O'Shea, Neural differentiation of embryonic stem (ES) cells, in Neural Stem Cells, ed. T. Zigova, P. Sanberg and J. Sanchez-Ramos, Totowa, NJ, Humana Press, 2002, pp. 3–14 Search PubMed.
- H. Hirose,
et al., Mouse ES cells maintained in different pluripotency-promoting conditions differ in their neural differentiation propensity, In Vitro Cell. Dev. Biol. – Animal, 2012, 48(3), 143–148 CrossRef PubMed.
- Q. L. Ying,
et al., Conversion of embryonic stem cells into neuroectodermal precursors in adherent monoculture, Nat. Biotechnol., 2003, 21(2), 183–186 CrossRef CAS PubMed.
- M. Riepl,
et al., Molecular gradients: an efficient approach for optimizing the surface properties of biomaterials and biochips, Langmuir, 2005, 21(3), 1042–1050 CrossRef CAS PubMed.
- M. Maden, Role and distribution of retinoic acid during CNS development, Int. Rev. Cytol., 2001, 209, 1–77 CAS.
- J. M. Breier,
et al., Development of a high-throughput screening assay for chemical effects on proliferation and viability of immortalized human neural progenitor cells, Toxicol. Sci., 2008, 105(1), 119–133 CrossRef CAS PubMed.
- S. P. Massia and J. A. Hubbell, Immobilized amines and basic amino acids as mimetic heparin-binding domains for cell surface proteoglycan-mediated adhesion, J. Biol. Chem., 1992, 267(14), 10133–10141 CAS.
- R. Hochreiter,
et al., Long chain diamines inhibit growth of C6 glioma cells according to their hydrophobicity. An in vitro and molecular modeling study, Naunyn-Schmiedeberg's Arch. Pharmacol., 2000, 361(3), 235–246 CrossRef CAS PubMed.
- R. J. Bergeron,
et al., Role of the methylene backbone in the antiproliferative activity of polyamine analogues on L1210 cells, Cancer Res., 1989, 49(11), 2959–2964 CAS.
- R. J. Bergeron,
et al., Antiproliferative properties of polyamine analogs – a structure–activity study, J. Med. Chem., 1994, 37(21), 3464–3476 CrossRef CAS PubMed.
- S. J. Lee,
et al., The effect of surface wettability on induction and growth of neurites from the PC-12 cell on a polymer surface, J. Colloid Interface Sci., 2003, 259(2), 228–235 CrossRef CAS PubMed.
- K. Abe,
et al., Structural requirement for axonal regeneration-promoting effect of polyamines in cultured rat hippocampal neurons, Brain Res., 1997, 766(1–2), 281–284 CrossRef CAS PubMed.
- L. J. Millet,
et al., Guiding neuron development with planar surface
gradients of substrate cues deposited using microfluidic devices, Lab Chip, 2010, 10(12), 1525–1535 RSC.
- D. N. Adams,
et al., Growth cones turn and migrate up an immobilized gradient of the laminin IKVAV peptide, J. Neurobiol., 2005, 62(1), 134–147 CrossRef CAS PubMed.
- H. Baier and F. Bonhoeffer, Axon guidance by gradients of a target-derived component, Science, 1992, 255(5043), 472–475 CAS.
- D. Bagnard,
et al., Spatial distributions of guidance molecules regulate chemorepulsion and chemoattraction of growth cones, J. Neurosci., 2000, 20(3), 1030–1035 CAS.
- Z. D. Wissner-Gross,
et al., Large-scale analysis of neurite growth dynamics on micropatterned substrates, Integr. Biol., 2011, 3(1), 65–74 RSC.
Footnote |
† Equal contributions. |
|
This journal is © The Royal Society of Chemistry 2013 |
Click here to see how this site uses Cookies. View our privacy policy here.