DOI:
10.1039/C3BM00163F
(Paper)
Biomater. Sci., 2013,
1, 494-502
Fibroblasts remodeling of type IV collagen at a biomaterials interface
Received
31st October 2012
, Accepted 23rd January 2013
First published on 13th February 2013
Abstract
This paper describes the fate of adsorbed type IV collagen (Col IV) in contact with fibroblasts on model biomaterial surfaces, varying in wettability, chemistry and charge. We found that fibroblasts not only interact but also tend to remodel differently adsorbed Col IV employing two distinct mechanisms: mechanical reorganization and proteolytic degradation. Apart from the trend of adsorption –NH2 > CH3 > COOH > OH– the cells interact better with NH2 and OH surfaces – i.e. independently of the amount of adsorbed Col IV – evident from the quantitative measurements of cell adhesion and spreading and the improved recruitment of alpha 1 and alpha 2 integrins as well as p-FAK in focal adhesions. The linearly arranged Col IV co-localize with FN fibrils formed from either secreted, or exogenously added protein, which confirms their interdependence during a reorganization process. We further found that this reorganization is better pronounced on hydrophilic OH and positively charged NH2 surfaces correlating with the improved cellular interaction. Conversely, the fibroblasts tend to round on COOH and CH3 surfaces in compliance with the altered integrin signaling and also the increased pericellular proteolysis activity quantified by the increased de-quenching of adsorbed FITC-Col IV and zymography. Taken together, these results show that remodeling of Col IV at a cell–biomaterial interface depends strongly on the surface properties of a material and affects significantly its biological performance.
Introduction
The extracellular matrix (ECM) contains various spatiotemporal cues1,2 that affect virtually all aspects of cell functioning, including cell growth, survival, migration and differentiation.1–4 The cells recognize not only the composition of the ECM but also the variations in its physical properties including elasticity, topography and organization.5 An ECM is a highly dynamic structure since cells continuously build and reshape it.6 This physiological remodeling is critical during development and tissue repair, but may also have various pathological consequences, such as fibrosis, inflammation or tumor progression.6–9 Matrix remodeling comprises of synthesis, arrangement and degradation, and the balance between these processes determines its net loss or abnormal overaccumulation.10
The ECM undergoes remodeling also in contact with biomaterials11–13 as the initial molecular events that take place during their contact with cells mimic to a great extent the natural cell–ECM interaction.14–16 In fact most materials readily adsorb matrix proteins from the surrounding medium17 which are recognized by integrins.18,19 In response integrins cluster in focal adhesion complexes where specific bidirectional signaling converges with other cellular pathways18,19 in a common tyrosine phosphorylation mechanism20 involving focal adhesion kinase (FAK), Src, Cas, paxillin, within others.21 A large and growing body of evidence shows that the cells need to accept distinct mechanical stimuli from the surrounding structures to strengthen their connections with the cytoskeleton,22 thus responding to the mechanical properties of the environment.22,23 We anticipate24–27 that tissue compatibility of materials strongly depends on this “allowance” of cells to mechanically remodel adsorbed soluble matrix proteins24,25 and to organize a provisional ECM.16 We further show that under specific conditions other, less-soluble ECM proteins like collagens may also associate with the biomaterial interface,16e.g. directly secreted by the adjacent cells or pre-adsorbed in vitro under non-physiological (acid) conditions, and thus affecting their biological properties. One such protein is type IV collagen (Col IV)26,28–30 – the main structural component of the basement membranes (BM).31–33 Col IV plays a crucial role in various physiological and pathological processes33–35 but data on its behavior at biomaterials interfaces are generally missing. It is an important issue as it encompasses various concerns in tissue engineering, such as artificial organ technologies; biomaterials induced fibrosis, endothelization of implants and neovascularization within others.35–37 It is particularly not clear how material surface properties will affect Col IV remodeling. To address this we have employed different model surfaces varying in wettability, chemistry and charge, as well as fibroblasts as a model system considering their natural involvement (as stromal cells) in the synthesis, organization and remodeling of ECM,38 including of Col IV.39 Details of this study are reported below.
Materials and methods
Model surfaces
To render the glass surface hydrophilic (OH),28 standard microscopic coverslips (22 × 22 mm) or rounded (∅12 mm) ones (Fisher Bioblock) were cleaned in an ultrasonic bath for 10 min in a 1
:
1 mixture of 2-propanol and tetrahydrofuran and then exposed to piranha solution (30% (v/v) H2O2 and 70% (v/v) H2SO4) for 30 min, followed by a copious rinsing with Milli-Q water (18.2 MΩ) and drying.
Functionalization with self-assembled monolayer's (SAMs).
The hydrophobic CH3 surfaces were prepared using an organosilanetrichloro(octadecyl)silane (ODS) purchased from Sigma (Cat. No 104817), as previously described.28 Briefly, the glass samples cleaned as above were placed in a solution containing 12.5 ml of carbon tetrachloride, 37.5 ml of heptane and 220 μl of ODS. The samples were left in this solution for 18 min at room temperature and the excess of silane was washed away with pure heptane. Samples were then heated for one hour at 80 °C.
For NH2 functionalization slides pre-cleaned as above were immersed for 18 min at room temperature in a solution containing 30 ml methanol, 10 ml 4% acetic acid glacial and 3-(2-aminoethylamino) propyltrimethoxysilane (C8H22N2O3Si, Fluka) to yield a final 1% concentration. An excess of silane was washed by immersion in an excess of solvent solution. Finally, the samples were air dried and heated at 80 °C for 1 h.29
COOH functionalization was performed in two steps, by immersing the pre-cleaned samples in a 1
:
3 mixture of CCl4 and n-C7H16 containing 0.01 M 10-(carbomethoxy) decyldimethylchlorosilane (C14H29ClO2Si, ABCR GmbH & Co) for 4 h at 4 °C, which create COOHCH3 functions. Samples were then washed in a silane-free solvent, heated as above and immersed overnight in a 12 M HCl solution to create COOH surfaces as a second step.29
The model surfaces were cleaned with distilled water in an ultrasonic bath, dried and coated with native Col IV, as follows: the samples were overlaid for 30 min at 37 °C with a solution of 50 μg ml−1 native Col IV (Abcam, Cat. No ab7536, UK) in 0.1 M sodium acetate (pH 4.5), then washed in PBS for immediate use (cellular studies). For quantitative studies the samples were washed with distilled water and dried in air.
The samples were coated with monomeric FITC-labeled Col IV (FITC-Col IV) from human placenta (Molecular Probes, Cat. No D-12052) for 30 min at 37 °C using 50 μg ml−1 solution dissolved in PBS, followed by extensive washing with PBS (for immediate use).
Quantification of adsorbed type IV collagen
The adsorption of FITC-Collagen IV (FITC-Col IV) was quantified by NaOH extraction as previously described.28 Briefly, the model surfaces were coated with FITC-Col IV as above then dried and extracted with 250 μl of 0.2 M NaOH for 2 h at room temperature. The fluorescent intensity of the extracts was measured with a fluorescent spectrometer (Horiba-Jobin y Von, USA) set to 488 nm (excitation) and 530 nm (emission) and compared to a standard curve based on known concentrations of FITC-Col IV solutions in 0.2 M NaOH.
Water contact angle measurement
The wettability of OH, CH3, NH2 and COOH surfaces was measured with water contact angles using a sessile drop technique performed on Dataphysics Contact Angle Systems OCA15. The same measurements were performed with dried samples after coating with native Col IV and drying (see above). Average values were obtained from 3 measurements of at least three different samples.
Atomic force microscopy
AFM measurements were performed on dried model surfaces coated with native Col IV as above for 10 min. We have used the AFM NanoScope III from Digital Instruments (Santa Barbara, CA) operating in the tapping mode in air utilizing a Si cantilever from Veeco (Manchester, UK) with a force constant of 2.8 N m−1 and a resonance frequency of 75 kHz. The phase signal was set to zero at the resonance frequency of the tip. The tapping frequency was 5–10% lower than the resonance one. The drive amplitude was 200 mV and the amplitude set point (Asp) was 1.4 V. The ratio between the amplitude set point and the free amplitude was kept equal to 0.7. AFM images were analysed using the WSxM software (Nanotec, Spain) to observe the initial topography of non-coated surfaces, as well as, the uniform distribution of protein on the different SAMs.
Cells
Human dermal Fribroblast purchased by PromoCell (Cat. No. C-12302) was cultured in Dulbecco's modified Eagle's medium (DMEM) supplemented with 10% fetal bovine serum (FBS), 1 mM sodium pyruvate, 2 mM L-glutamine, and penicillin–streptomycin, all of them purchased from Invitrogen. The growth medium was exchanged each 3rd day. For the experiments the cells were detached from around confluent flasks with tripsin/EDTA (Invitrogen) and the remaining trypsin activity was stopped with FBS before 2 times washing with a pure medium to remove any traces of serum proteins. Finally, the cells were resuspended in serum free DMEM.
Initial cellular interaction
105 cells per well were seeded in 6 well TC plates (Costar) containing the above functionalized samples (22 × 22 mm) for 2 h in a serum free medium. Typically, the samples were pre-coated with native Col IV or monomeric FITC-Col IV as stated above. At the end of incubation, the cells were fixed with 4% paraformaldehyde (20 min), permeabilized with 0.5% Triton X-100 for 5 min and saturated for 15 min in PBS containing 1% albumin. To study the overall cell morphology we viewed an actin cytoskeleton stained for 30 min with FITC-phalloidin (Molecular Probes, Cat. No. A12379), while for quantification of adhesion (see below) we co-stained the nuclei with Hoechst 34580 (Invitrogen, Cat. No. H21486). Both dyes were dissolved in PBS containing 1% albumin. Finally, the samples were mounted on Mowiol, viewed and photographed on a fluorescent microscope Axio Observer Z1 (Zeiss, Germany). At least 3 representative images for each magnification were acquired. For some experiments focal adhesions were viewed simultaneously using a monoclonal anti-vinculin antibody (Sigma, Cat. No. V9131) dissolved in PBS-1% albumin for 30 min followed by AlexaFluor 555 goat anti-mouse (Invitrogen) as a secondary antibody.
Quantification of cell adhesion and spreading
The number of adhering cells and the mean cell surface area were quantified using an Image J plug-ins (NIH, USA). The adhesion was measured by counting the cell nuclei in at least 3 randomly chosen squares from the images acquired for each sample (10× magnification) using the blue channel of the microscope. The average cells area was further measured (in μm2) at 20× magnification in the green channel of the microscope (to visualize the overall shape via cellular actin). Three samples were studied for each condition and the results presented are from 3 independent experiments.
Integrin signalling
105 cells per well were seeded for 2 h on Col IV coated samples. α1 and α2 integrins were viewed separately using specific monoclonal anti-human integrin antibodies (Chemicon, Cat. No. MAB1973) and (Abcam, Cat. No. Ab24697), respectively, followed by AlexaFluor 555 goat anti-mouse (Invitrogen) as a secondary antibody. In some cases FITC-phalloidin was added to the secondary antibody to visualize the actin cytoskeleton. The samples were viewed and photographed in a fluorescent microscope Axio Observer as above. At least 3 representative images were acquired (63×) for each experimental condition.
To visualize simultaneously the focal adhesions (vinculin) and phosphorylated focal adhesion kinase (p-FAK) we used the primary monoclonal antibody for vinculin as above combined with a polyclonal anti-p-FAK (Tyr925) antibody (Cell Signaling, Cat. No. 3284), both dissolved in PBS-1% albumin, and followed by AlexaFluor 488 goat anti-mouse (Invitrogen) and AlexaFluor 555 goat anti-rabbit (Invitrogen), respectively, as a secondary antibody. Preliminary studies omitting first or the secondary antibodies were performed confirming no cross-reactivity.
Type IV collagen remodelling
To study the fate of adsorbed Col IV glass cover-slips (diameter 12 mm) were coated with native Col IV or FITC-Col IV as above. After washing with PBS, 5 × 104 fibroblasts were seeded in a serum free medium and cultured for a time as indicated (5 or 24 hours). 10% serum was added to the medium at the 2nd hour considering the importance of serum proteins for the reorganization process (loosening the protein to substratum interaction).16 At the end of incubations all samples were fixed, permeabilized and stained for native Col IV (see below) or directly mounted (FITC-Col IV samples).
Native Col IV samples were processed for immunofluorescence using a monoclonal anti-collagen IV antibody (Milllipore, Cat. No. MAB1910) followed by Cy3-conjugated goat anti-mouse IgG (Jackson ImmunoResearch, Cat. No. 115-165-062) or AlexaFluor 488 (Invitrogen, Cat. No. A11001) as a secondary antibody. The samples were viewed and photographed on a fluorescent microscope as above.
Co-localization of type IV collagen with fibronectin
To study co-localization of remodelled Col IV with secreted FN the cells were cultured for different time periods (as indicated) on native Col IV or FITC-Col IV coated model surfaces. Native Col IV was viewed as explained above using AlexaFluor 488 as a secondary antibody, while secreted FN was viewed by polyclonal anti-FN (Sigma, Cat. No. F3648) followed by AlexaFluor 555 anti-rabbit (Invitrogen, Cat. No. A21428), as in the other experimental set-ups when FITC-Col IV was used. To study co-localization of Col IV with exogenously added FN, the cells were cultured for 4 hours on native Col IV and at 3rd hour 100 μg ml−1 of human plasma FN (Sigma, Cat. No. F2006) was added to the medium for 1 h prior to fixation, and finally co-stained for Col IV and FN as above.
To study co-localization of secreted FN with exogenously added FITC-Col IV the cells were cultured on serum coated samples for 24 hours and 1 hour before the end of incubation 100 μg ml−1 FITC-Col IV was added, then the FN was stained on fixed samples as above.
All samples were finally mounted on Mowiol and viewed and photographed on a fluorescent microscope Axio Observer Z1 (Zeiss, Germany) at different magnification. At least 3 representative images for each magnification were acquired.
Pericellular proteolytic activity
De-quenching of FITC-Col IV: the monomeric FITC-Col IV (Molecular Probes) is conjugated with FITC in such a way that part of its fluorescence is quenched. It results in a significant increase of the quantum yield after proteolytic degradation of the protein. As our preliminary experiments have shown that NaOH extraction by itself does not induce any dequenching of adsorbed FITC-Col IV (it was compared with trypsin-treated samples; not shown), we used this method to quantify the pericellular proteolytic activity, measuring the increase of an extracted fluorescence signal from samples with cells versus without cells. For that purpose the model surfaces were coated with FITC-Col IV and seeded with fibroblasts (105 cells per well) for 5 h (2 h in a serum-free medium, than 10% serum was added for the next 3 more hours). Then the samples were washed and extracted with 0.2 M NaOH as described above, and measured with a fluorescent spectrometer set to 488 nm (excitation) and 530 nm (emission). The ratio of the fluorescent signal from samples with cells, and the signal from identically treated samples without cells, characterized the dequenching activity (i.e. the pericellular proteolysis). All experiments were performed in triplicates.
Zymography was used to study the activity of the two major types of MMPs (MMP-2 and MMP-9) known to cleave Col IV. They were measured in the conditioned medium from fibroblasts cultured for 24 h on model materials. For that purpose, 25 μl of each supernatant was mixed with 5 μl of sample buffer (0.04 M Tris-HCl, pH 6.8, 4% SDS, 33% glycerin, 0.04% bromophenol blue) and charged on a Ready Gel Zymogram (Biorad, 15-well, 10% gelatin) followed by standard gel electrophoresis at 50 V for 4 h at 4 °C. The gels were then incubated in 2.5% Triton X-100 for 30 min before overnight incubation in renaturation buffer (1 M Tris-HCl, pH 7.5, 5 M NaCl, 1 M CaCl2, 10% Triton X-100) at 37 °C. After staining with 0.5% Coomassie blue R-250 (in 30% methanol/10% acetic acid) and destaining, gelatinolytic activity was detected as white (unstained) bands on the blue background of the sample and quantified using a molecular imager gel Doc+ (Biorad).
Statistical analysis was performed using Stat Graphics Plus software employing the ANOVA test to determine statistically significant differences between groups (p < 0.05). Each data point represents mean ± standard deviation (SD) from at least three independent experiments.
Results
The data presented in Fig. 1A (black columns) show a significant change of water contact angles (WCA°) confirming the successful and homogenous functionalization of samples: the CH3 surface shows about twice higher WCA° in comparison to NH2 and about triple values compared to OH and COOH substrata (p < 0.05). However, after coating with Col IV (white columns) the hydrophobicity decreased substantially and tends to equilibrate at values between 20° and 35°, suggesting that the cells will not “assess” difference in wettability upon contact with these surfaces. That is to say, surface chemistry influences protein adsorption and conformation, but not the physical properties.
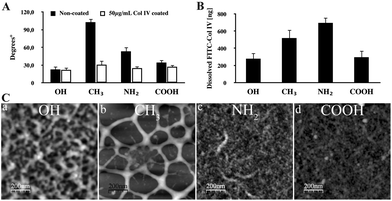 |
| Fig. 1 Adsorption of Col IV on model material surfaces. (A) Water contact angles of model OH, CH3, NH2, and COOH surfaces measured before (black bars) and after coating with 50 μg ml−1 native Col IV (white bars). (B) Amount of FITC-Col IV adsorbed from a solution of 50 μg ml−1 on different surfaces and extracted with 0.1 M NaOH (see the Methods section). (C) AFM topographic images of adsorbed native Col IV (50 μg ml−1/10 min) on different model surfaces. | |
The amount of adsorbed Col IV was measured, using extraction with 0.2 M NaOH as previously described,28,29 and compared to a standard curve with known FITC-Col IV concentrations. The adsorption concentration was set to 50 μg ml−1 not accidently, but based on our previous studies,28,29 showing saturation for the same model surfaces at concentrations between 30 and 50 μg ml−1. The same conditions were applied also for the cellular studies. Indeed, as shown in Fig. 1B, detectable values of protein were obtained for all substrata following the trend of adsorption as close as – NH2 > CH3 > COOH > OH, where CH3 and NH2 demonstrated significant (p < 0.05), about twice higher adsorption, compared to OH and COOH, while both doubles did not differ significantly from each other (p > 0.05).
To learn more about the adsorption pattern of native Col IV we used AFM as previously described.28,29 AFM shows a clear tendency for non-homogenous adsorption to the different surfaces (Fig. 1C). A prominent network consisting of the above molecular size features was found on CH3 suggesting the deposition of Col IV as molecular aggregates.28 Consistent with the later study this network was much “finer” on OH, representing single molecular features resembling dimers and tetramers. Conversely, a rather dense structure, consisting of small molecular-sized features, was observed on NH2, which were less prominent on COOH, with appearance of specific fibril-like supramolecular structures on NH2.29
Cellular interaction
The typical morphology of fibroblasts adhering for 2 h on native Col IV coated model surfaces is shown in Fig. 2A (a–h). On OH (a) and NH2 (c) surfaces fibroblasts attach better representing their regular flattened morphology. On COOH (d, h) and CH3 (b, f) adhesion was less efficient with appearance of both round and flattened cells suggesting delayed spreading (f, h). The quantitative data for cell adhesion (B-left) and spreading (B-right) showed significant (*) decrease for both parameters on CH3 substrata only (p < 0.05).
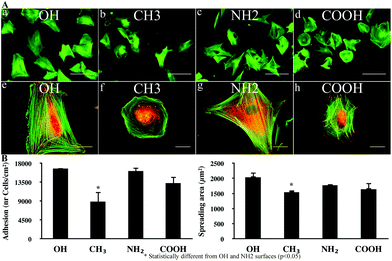 |
| Fig. 2 Initial cellular interaction: (A) overall morphology of human fibroblasts adhering to Col IV coated model materials for 2 h under serum free conditions. The cells were stained simultaneously for actin (green) and vinculin (red). On (a–d) the cells are viewed for actin at low magnification to follow the overall cell morphology (Bar – 100 μm), while the higher magnification on (e–h) depicts the development of focal adhesion contacts and actin filaments (Bar – 20 μm); (B) shows graphical illustrations of the quantities for cell adhesion (left) and spreading (right). | |
Integrin expression and signalling
To follow the behaviour of integrins we studied the organization of α1β1 and α2β1 heterodimers referred to as the main collagen receptors.19,33 As shown in Fig. 3, both α1 and α2 integrins are better expressed in fibroblasts adhering to OH and NH2 surfaces (a, e and c, g, respectively). However, α1 integrin (a and c) represents a rather linear pattern, while α2 (e and g) shows clusters resembling focal adhesion contacts. On CH3 and COOH surfaces almost no α1 clusters were found (b and d, respectively), but α2 integrins still formed clusters resembling small focal adhesions (f and h).
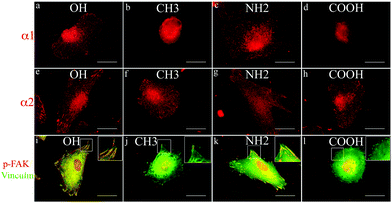 |
| Fig. 3 Integrin expression and signalling. Expression of α1 (a–d) and α2 (e–h) integrins by fibroblasts adhering to Col IV coated model surfaces for 2 h under serum free conditions. (i–l) Double staining for vinculin (green) and p-FAK (red) of fibroblasts seeded on different Col IV coated model surfaces (only merged images are presented) demonstrating the recruitment of signalling molecules in focal adhesion complexes. Bar – 20 μm. | |
To learn whether the development of focal adhesion complexes induces recruitment of phosphorylated signalling molecules we co-stained for vinculin and p-FAK. The data presented in Fig. 3 (i to l) show a higher degree of co-localization between the well-developed focal adhesion contacts (vinculin in green) and p-FAK (viewed in red) on OH and NH2 substrata (not shown), resulting in orange on merges (i and k, respectively). Conversely, on CH3 and COOH surfaces although some cells make small focal contacts (j and l, in green), less p-FAK is recruited.
Fibroblast reorganization of type IV collagen
Fig. 4A demonstrates that within 5 h of culture fibroblasts not only interact with adsorbed Col IV (on regular glass), but also tend to reorganize in a fibril-like pattern both native (a) and monomeric FITC-Col IV (b). The dark zone at cell borders represents the places from where the protein was removed to be arranged. No difference between patterns of native and monomeric Col IV was found.
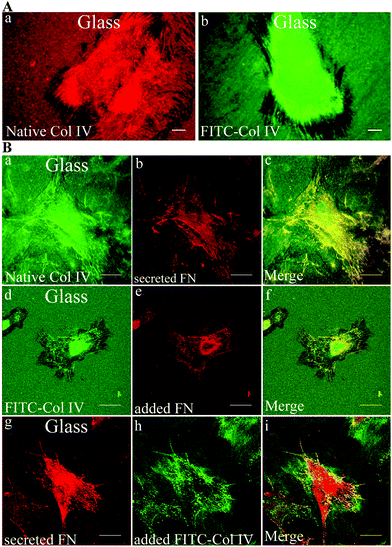 |
| Fig. 4 Remodelling of adsorbed Col IV – the role of fibronectin. (A) Fibroblast reorganization of adsorbed native (a) and FITC-labelled (b) Col IV after 5 h of culture, bar – 10 μm. (B) This panel represents the co-localization between remodelled native Col IV (a, d) and FN (b, e, g) in three different protocols. Fibroblasts were seeded for 5 h on Col IV coated glass and stained for: (b) endogenous FN fibrils; (e) FN is exogenously added and stained in the same way; (e) and (f) are the respective merged images with rearranged Col IV (a, d). On the lower panel the secreted endogenous FN (g) is viewed simultaneously with exogenously added FITC-Col IV (h) and on the right both images are merged (i). Bar – 20 μm. | |
One possible mechanism for Col IV reorganization is its tethering by FN molecules during fibrillogenesis. To prove such possibility we performed a separate experiment where FN and Col IV were “exposed” to each other in three different protocols and the results are summarized in Fig. 4 (panel B). The upper row (a–c) represents a native Col IV reorganization by fibroblasts after 5 h of incubation (a); viewed simultaneously with secreted FN (b); both images are merged on (c). The middle row shows how reorganized Col IV (d) co-localizes with FN fibrils formed from exogenously added FN to the medium (e); merged on (f). Finally, the lower row shows the result when soluble FITC-Col IV was added to the medium (h) and viewed together with secreted FN (g), then merged at (i). All three conditions showed a high degree of co-localization, no matter of native or monomeric Col IV were used.
Reorganization of type IV collagen on different model surfaces
The morphological observations on the reorganization of adsorbed native Col IV and the monomeric (FITC-labelled) Col IV were similar, therefore, we show only the data with FITC-Col IV (Fig. 5A), where dark zones of substratum removal and also fibril-like arrangements of protein (upper row) can be seen on all materials. As shown in (a–d), however, the cells arrange FITC-Col IV better on OH and NH2 (a, c), which corroborates with the pronounced FN fibrils formation (e, g). The higher degree of co-localization results in orange on merges (i and k). However, on NH2 substrata (c) the removal of Col IV is absent, while on OH it is well expressed (a). COOH and CH3 substrata also show some specificity: Col IV removal (black zones) is strongest on CH3 (b) but much lower on COOH (d), although preserving some co-localization with FN fibrils on both substrata (j and l).
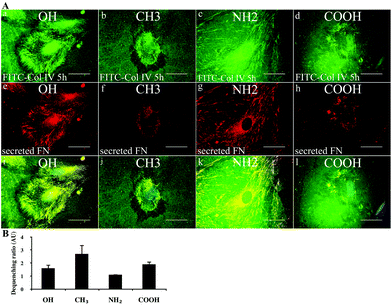 |
| Fig. 5 Remodelling of FITC-Col IV on model surfaces. (A) Comparative morphological view of FITC-Col IV remodelling by fibroblast on different model surfaces after 5 h of incubation (a–d) viewed simultaneously with endogenously secreted FN matrix fibrils (e–h). The images are merged on (i–l). Bar – 20 μm. Note the dark zones show places where FITC-Col IV is removed; it results in fibril-like arrangements that often co-localize with fibronectin fibrils (e–h) seen on all materials. (B) De-quenching of adsorbed FITC-Col IV on different model surfaces by the adhering cells after 5 h of incubation. The graph represents the ratio between extracted fluorescence signals from samples with cells versus without cells given in arbitrary units (AU) for the different materials. | |
Enzymatic remodelling of type IV collagen on model surfaces
De-quenching of FITC-Col IV.
An advantage of using FITC-Col IV is that it provides opportunity for quantification of fibroblast degradation activity leading to de-quenching of its fluorescence (see the Methods section). Fig. 5B shows the ratios between extracted fluorescent signals from samples with cells versus without cells for all surfaces after 5 hours of incubation. The signal increases (higher than 0) because of the dequenching caused by pericellular proteolysis, being normalized to the initial substratum fluorescence, which compensate the differences in adsorption. Indeed, the graph shows significantly higher Col IV degradation by fibroblasts on CH3 (p < 0.05), followed by COOH and OH surfaces, which did not differ significantly from each other (p > 0.05). The lowest degradation of Col IV (p < 0.05) was measured on the NH2 surface.
Zymography.
This was used to confirm the involvement of MMP-2 and MMP-9 in the proteolytic degradation of native Col IV. The fibroblasts were seeded for 24 h on OH, CH3, NH2, and COOH surfaces coated with native Col IV. Fig. 6 (panel A) shows that, morphologically, the remodelling of native Col IV follows the same trend as FITC-Col IV (Fig. 5), i.e. improved reorganization on NH2, then on OH, correlating with stronger deposition of an FN matrix, while both processes are inhibited on CH3 and partly on COOH. Though the black zones of protein removal are seen on both OH and COOH, the protein is obviously absent around cells on CH3.
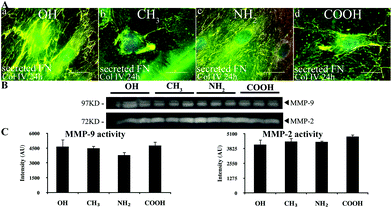 |
| Fig. 6 Remodelling of adsorbed Col IV. (A) The cells were cultured on model surfaces coated with native Col IV, then the samples were simultaneously stained for Col IV (green) and FN (red), and only merged images are shown on (a–d). Bar – 20 μm. (B) Zymography of the conditioned medium after 24 h of fibroblasts culture on model surfaces showing expression of both MMP-9 and MMP-2 activities at 97 kDa and 72 kDa, respectively. (C) Densitometry results for MMP-9 (left) and MMP-2 (right). | |
Analysis of the conditioned medium with zymography showed the involvement of both MMP-9 and MMP-2 in Col IV degradation (Fig. 6, panel B). The densitometry analysis however shows no significant differences between MMP-2 activity in different samples (C – right), while MMP-9 activity was significantly suppressed on the NH2 substrata (C – left).
Discussion
This study shows that human fibroblasts not only interact with adsorbed Col IV, but also tend to remodel it in a distinct pattern depending on the materials surface properties. Two types of cell activities might be foreseen: substratum Col IV reorganization and its degradation via enzymatic cleavage. In agreement with previous investigation26 here we confirm that fibroblasts are able to mechanically translocate adsorbed Col IV in a fibril-like pattern that frequently co-localizes with FN matrix fibrils,40,41 and further show that it differs between model surfaces. It is noteworthy that Col IV is not fibrillar protein and typically it is observed in the basement membrane (BM), where it assembles in a sheet-like structure providing the major structural support for organ-specific cells.32,33,35,37 Col IV molecules assemble by anti-parallel interactions and extensive disulfide bonding of three monomers. Native (triple-helical) Col IV molecules associate through NC1 domain forming dimers and through 7S domain forming tetramers; further lateral interaction and complex disulfide bonding create the network that forms the BM (together with laminin and other linkage proteins).33,42 The role of adjacent cells in these processes, however, is not clear. From this angle the linear fibril-like rearrangement of Col IV by fibroblasts in 2D substrata does not look physiologically relevant. But it is noteworthy that similar linear organization of Col IV has been observed during early basement membrane assembly in an in vitro 3D skin culture model,43 shown to be a cell-driven process. Notwithstanding concerns on its physiological relevance, our data suggest that the driving force for Col IV reorganization is the association with FN fibrils. In fact, such a mechanism is demonstrated for fibrillar collagen types I and III,44,45 and also for adsorbed fibrinogen.25 We were the first however showing that it works also for the substratum associated Col IV.26 Indeed, the double staining experiments, aimed to further corroborate Col IV reorganization with the material surface properties, again demonstrate a high degree of co-localization between FN and Col IV, moreover at all model substrata, confirming active involvement of FN in the reorganization process. In fact, the association between these ECM proteins is not surprising, as an FN molecule has at least two binding sites for collagens8,46 and corresponding binding sites for FN have been identified on the collagen molecule.44,47,48
FN is synthesized by many types of cells, including fibroblasts, which assemble it into a fibrillar network.49 During its assembly, FN undergoes conformational changes that expose FN-binding sites and promote intermolecular interactions important for the fibril formation.46 The earlier work of Dzamba et al.50 shows that the FN binding site in fibrillar type I collagen may regulate FN fibril formation by fibroblasts. Conversely, Sottile and Hocking47 show that FN polymerization into the ECM is required for the deposition of type I collagen. Furthermore, Chernousov et al.51 reported that Schwann cells use directly a Col IV-dependent mechanism for FN fibril assembly. Consistent with this are our comprehensive data showing that FN and Col IV frequently co-localize, either when pre-adsorbed or exogenously added in the medium (Fig. 4). Hence, in contrast to the earlier belief that collagen polymerization occurs only via self-assembly35 our data showed that the preformed FN matrix is essential for Col IV network formation. As FN matrix assembly is strongly dependent on the material surface properties24,41 it becomes the most probable explanation for the observed differences in Col IV reorganization, turning some light on its physiological relevance. In this respect it is worthy to mention the recent work of Trappmann describing tethering of substratum-associated collagen as important feedback guiding cell-fate decisions.52 Employing completely different chemistry the authors conclude that stem cells insert a mechanical force on collagen molecules that is critical for triggering the ECM related signaling events, in this case determining their switch to differentiation.52 The co-localization however is not obligatory, suggesting also an independent translocation of the protein onto the cell surface, now dependent on collagen specific integrins.47 The integrins are active and even tend to organize in a distinct linear pattern, as shown in Fig. 3 for α1 integrin on NH2 and OH surfaces. On the other hand, it looks that Col IV reorganization does not require the native configuration of protein as it is equal when partly denatured (monomeric) FITC-Col IV, or a native one has been used. But it does require the presence of serum proteins in the medium as in serum free media no reorganization was observed (not shown). It seems that serum proteins act non-specifically, loosening the protein–substratum interaction (as do with fibronectin)16,24,41 thus enabling the cells to remove and translocate Col IV over the substratum. This, together with the fact that denatured collagen possesses even higher affinity for FN,53 suggests that the transient association with FN fibrils is more important for Col IV reorganization than its specific recognition by integrins.
A novel observation in this study is that fibroblast reorganization of Col IV strongly depends on the material surface properties: it is supported on hydrophilic OH and a positively charged NH2 substratum, while it is abrogated on negatively charged COOH and a hydrophobic CH3 surface. It is of particular tissue engineering interest as it comprises an approach for controlling the provisional ECM deposition on implants, e.g. affecting the material-induced fibrosis or foreign body reaction.6,9 The pattern of Col IV reorganization is also different: on OH the dark zones of removal are clearly visible, while on an NH2 surface, zones of protein removal were not observed. It seems on NH2 fibroblasts cannot remove adsorbed Col IV presumably because the protein is too strongly bound. But they can use the protein that overlay the substratum-adjacent molecules, which also can be of consideration when one wants to guide the cellular behavior on an implant. Indeed, AFM images show appearance of fibril-like suprastructures on NH2 suggesting multilayered deposition of protein (Fig. 1) that could be the source for Col IV arrangement. Conversely, the fine network-like structure of Col IV on OH is easy to be removed from the substratum, because of the lowered strength of interaction characteristic for hydrophilic substrata.24,40 It is noteworthy, however, that such a hydrophilic environment provokes Col IV arrangement in a network consisting of dimers and tetramers,28 suggesting assemblies in a rather natural conformation.
Another important issue is that apart from the trend of Col IV adsorption –NH2 > CH3 > COOH > OH– the cells interact better with NH2 and OH surfaces, i.e. act independently of the amount of adsorbed Col IV, rather following their allowance to reorganize the adsorbed protein. This was evident from the quantities for cell adhesion and spreading, the expression of α1 and α2 integrins, and also p-FAK recruitment in focal adhesions – all characterizing the proper function of cell adhesion machinery. It is noteworthy that the same surfaces (OH an NH2) support FN reorganization, which could be a reason for facilitating Col IV translocation. Conversely, the fibroblasts tend to round on COOH and CH3 surfaces correlating with the lowered Col IV reorganization, also with the abrogated fibronectin matrix deposition (Fig. 5f, h).
Of course the role of other matrix proteins like laminins or any other ECM component, coming either from the serum or secreted by the cell during the experiment, cannot be excluded. Particularly for laminin, our recent studies showed that it tends to form a joint network with Col IV upon adsorption,30 which could significantly affect the process of remodeling, moreover laminin is secreted by fibroblasts.54 However, such studies were out of the scope of the present work, but obviously need further attention. Nevertheless, the role of FN fibrillogenesis in the matrix remodeling process is well-documented55,56 and our results confirm it. In general AFM shows non-homogenous adsorption of Col IV (Fig. 1C) with prevailing tendency for protein–protein interactions. The peculiar network found on CH3
28 suggests the deposition of Col IV in aggregates that are growing in the vertical dimension,28 and possessing lowered bioactivity. The same lowered interaction shows fibroblasts on COOH, but the pattern of Col IV organization is different – a rather dense structure, consisting of small globular features. Thus, it becomes obvious that other mechanisms are involved in the recognition of the adsorbed protein layer by the cells.
One such mechanism could be the proteolytic cleavage of adsorbed Col IV by the cells. Indeed, zymography shows activity of both MMP-2 and MMP-9 in the medium, but differences between surfaces were found for MMP-9 only, showing the significantly lower activity (p < 0.05) on NH2 substrata. This may explain the favored cellular interaction with NH2 but not with the OH surface, neither the abrogated interaction with COOH and CH3. Obviously the answer is not simple, but one can hypothesize that even when cleaved most of the protein remains on the substratum. Indeed, when de-quenching of adsorbed FITC-Col IV was measured, a significantly higher amount of cleaved protein was observed on CH3, followed by COOH and OH. It seems when cells face the aggregated forms of Col IV, particularly in a hydrophobic environment, they tend to degrade the protein while the rather physiological assemblies of Col IV on OH substrata suppress the degradation process, while favoring reorganization ones. Interestingly on positively charged NH2, although the highest amount of protein adsorbs, almost no substratum Col IV degradation was observed, which agrees with the enhanced FN matrix formation.
Conclusions
Taken together these results support our view that the ability of cells to remodel surface associated proteins strongly affects the biological performance of a biomaterial. They also show that the appropriate chemical functionalization (NH2, OH), combined with Col IV pre-adsorption, comprises a prospective surface modification that mimic the organization of basement membrane and might improve the biocompatibility of an implant.
Acknowledgements
The current work was supported by the Spanish Ministry of Science and Innovation, through projects MAT2012-38359-C03-03 to GA and a collaborative intramural project S-2011-2012 with CIBER-BBN.
Notes and references
- K. S. Kolahi and M. R. Mofrad, Wiley Interdiscip. Rev.: Syst. Biol. Med., 2010, 2, 625–639 CrossRef CAS.
- M. Aumailley and B. Gayraud, J. Mol. Med., 1998, 76, 253–265 CrossRef CAS.
- R. O. Hynes, Science, 2009, 326, 1216–1219 CrossRef CAS.
- M. F. Goody and C. A. Henry, Mol. Reprod. Dev., 2010, 77, 475–488 CrossRef CAS.
- V. Vogel and G. Baneyx, Annu. Rev. Biomed. Eng., 2003, 5, 441–463 CrossRef CAS.
- W. P. Daley, S. B. Peters and M. Larsen, J. Cell Sci., 2008, 121, 255–264 CrossRef CAS.
- R. Ala-aho and V. M. Kahari, Biochimie, 2005, 87, 273–286 CrossRef CAS.
- M. Larsen, V. V. Artym, J. A. Green and K. M. Yamada, Curr. Opin. Cell Biol., 2006, 18, 463–471 CrossRef CAS.
- T. A. Wynn, J. Pathol., 2008, 214, 199–210 CrossRef CAS.
- F. Shi, J. Harman, K. Fujiwara and J. Sottile, Am. J. Physiol.: Cell Physiol., 2010, 298, C1265–C1275 CrossRef CAS.
- E. S. Place, N. D. Evans and M. M. Stevens, Nat. Mater., 2009, 8, 457–470 CrossRef CAS.
- V. Llopis-Hernández, P. Rico, J. Ballester-Beltrán, D. Moratal and M. Salmerón-Sánchez, PLoS One, 2011, 6, e19610 Search PubMed.
- S. M. Irvine, J. Cayzer, E. M. Todd, S. Lun, E. W. Floden, L. Negron, J. N. Fisher, S. G. Dempsey, A. Alexander, M. C. Hill, A. O'Rouke, S. P. Gunningham, C. Knight, P. F. Davis, B. R. Ward and B. C. H. May, Biomaterials, 2011, 32, 6351–6361 CrossRef CAS.
- B. D. Ratner and S. J. Bryant, Annu. Rev. Biomed. Eng., 2004, 6, 41–75 CrossRef CAS.
- M. M. Stevens and J. H. George, Science, 2005, 310, 1135–1138 CrossRef CAS.
-
G. Altankov, T. Groth, E. Engel, J. Gustavsson, M. Pegueroles, C. Aparicio, F. J. Gil, M.-P. Ginebra and J. A. Planell, in Advances in Regenerative Medicine: Role of Nanotechnology, and Engineering Principles, ed. V. P. Shastri, G. Altankov and A. Lendlein, Springer, Netherlands, 2010, pp. 19–43 Search PubMed.
- F. Grinnell and M. K. Feld, J. Biol. Chem., 1982, 257, 4888–4893 CAS.
- A. J. Garcia, Biomaterials, 2005, 26, 7525–7529 CrossRef CAS.
- R. O. Hynes, Cell, 2002, 110, 673–687 CrossRef CAS.
- B. Geiger, A. Bershadsky, R. Pankov and K. M. Yamada, Nat. Rev. Mol. Cell Biol., 2001, 2, 793–805 CrossRef CAS.
- K. M. Yamada and B. Geiger, Curr. Opin. Cell Biol., 1997, 9, 76–85 CrossRef CAS.
- P. A. Janmey and C. A. McCulloch, Annu. Rev. Biomed. Eng., 2007, 9, 1–34 CrossRef CAS.
- B. Geiger, J. P. Spatz and A. D. Bershadsky, Nat. Rev. Mol. Cell Biol., 2009, 10, 21–33 CrossRef CAS.
- G. Altankov and T. Groth, J. Mater. Sci.: Mater. Med., 1994, 5, 732–737 CrossRef CAS.
- R. Tzoneva, T. Groth, G. Altankov and D. Paul, J. Mater. Sci.: Mater. Med., 2002, 13, 1235–1244 CrossRef CAS.
- L. Maneva-Radicheva, U. Ebert, N. Dimoudis and G. Altankov, Histol. Histopathol., 2008, 23, 833–842 CAS.
- J. Gustavsson, G. Altankov, A. Errachid, J. Samitier, J. A. Planell and E. Engel, J. Mater. Sci.: Mater. Med., 2008, 19, 1839–1850 CrossRef CAS.
- N. M. Coelho, C. Gonzalez-Garcia, J. A. Planell, M. Salmeron-Sanchez and G. Altankov, Eur. Cell Mater., 2010, 19, 262–272 Search PubMed.
- N. M. Coelho, C. Gonzalez-Garcia, M. Salmeron-Sanchez and G. Altankov, Biotechnol. Bioeng., 2011, 108, 3009–3018 CrossRef CAS.
- N. M. Coelho, C. Gonzalez-Garcia, M. Salmeron-Sanchez and G. Altankov, Tissue Eng. A, 2011, 17, 2245–2257 CrossRef CAS.
- R. Timpl and J. C. Brown, BioEssays, 1996, 18, 123–132 CrossRef CAS.
- K. Kühn, Matrix Biol., 1995, 14, 439–445 CrossRef.
- J. Khoshnoodi, V. Pedchenko and B. G. Hudson, Microsc. Res. Tech., 2008, 71, 357–370 CrossRef CAS.
- P. Yurchenco and J. Schittny, FASEB J., 1990, 4, 1577–1590 CAS.
- R. Kalluri, Nat. Rev. Cancer, 2003, 3, 422–433 CrossRef CAS.
- A. Charonis, V. Sideraki, V. Kaltezioti, A. Alberti, D. Vlahakos, K. Wu and E. Tsilibary, Curr. Med. Chem., 2005, 12, 1495–1502 CrossRef CAS.
- P. D. Yurchenco, Cold Spring Harbor Perspect. Biol., 2011, 3 Search PubMed.
- L. G. Griffith and M. A. Swartz, Nat. Rev. Mol. Cell Biol., 2006, 7, 211–224 CrossRef CAS.
- R. Kalluri and M. Zeisberg, Nat. Rev. Cancer, 2006, 6, 392–401 CrossRef CAS.
- F. Grinnell, J. Cell Biol., 1986, 103, 2697–2706 CrossRef CAS.
- G. Altankov and T. Groth, J. Mater. Sci.: Mater. Med., 1996, 7, 425–429 CrossRef CAS.
- R. M. Vanacore, S. Shanmugasundararaj, D. B. Friedman, O. Bondar, B. G. Hudson and M. Sundaramoorthy, J. Biol. Chem., 2004, 279, 44723–44730 CrossRef CAS.
- R. Fleischmajer, J. S. Perlish, D. E. Macdonald, A. Schechter, A. D. Murdoch, R. V. Iozzo and Y. Yamada, Ann. N. Y. Acad. Sci., 1998, 857, 212–227 CrossRef CAS.
- T. Velling, J. Risteli, K. Wennerberg, D. F. Mosher and S. Johansson, J. Biol. Chem., 2002, 277, 37377–37381 CrossRef CAS.
- K. E. Kadler, A. Hill and E. G. Canty-Laird, Curr. Opin. Cell Biol., 2008, 20, 495–501 CrossRef CAS.
- Y. Mao and J. E. Schwarzbauer, Matrix Biol., 2005, 24, 389–399 CrossRef CAS.
- J. Sottile and D. C. Hocking, Mol. Biol. Cell, 2002, 13, 3546–3559 CrossRef CAS.
- N. Zoppi, R. Gardella, A. De Paepe, S. Barlati and M. Colombi, J. Biol. Chem., 2004, 279, 18157–18168 CrossRef CAS.
- I. Wierzbicka-Patynowski and J. E. Schwarzbauer, J. Cell Sci., 2003, 116, 3269–3276 CrossRef CAS.
- B. J. Dzamba, H. Wu, R. Jaenisch and D. M. Peters, J. Cell Biol., 1993, 121, 1165–1172 CrossRef CAS.
- M. A. Chernousov, R. C. Stahl and D. J. Carey, J. Cell Sci., 1998, 111, 2763–2777 CAS.
- B. Trappmann, J. E. Gautrot, J. T. Connelly, D. G. Strange, Y. Li, M. L. Oyen, M. A. Cohen Stuart, H. Boehm, B. Li, V. Vogel, J. P. Spatz, F. M. Watt and W. T. Huck, Nat. Mater., 2012, 11, 642–649 CrossRef CAS.
- K. C. Ingham, S. A. Brew and B. S. Isaacs, J. Biol. Chem., 1988, 263, 4624–4628 CAS.
- D. T. Woodley, J. R. Stanley, M. J. Reese and E. J. O'Keefe, J. Invest. Dermatol., 1988, 90, 679–683 CAS.
- E. Cukierman, R. Pankov, D. R. Stevens and K. M. Yamada, Science, 2001, 294, 1708–1712 CrossRef CAS.
- X. Zhou, R. G. Rowe, N. Hiraoka, J. P. George, D. Wirtz, D. F. Mosher, I. Virtanen, M. A. Chernousov and S. J. Weiss, Genes Dev., 2008, 22, 1231–1243 CrossRef CAS.
|
This journal is © The Royal Society of Chemistry 2013 |
Click here to see how this site uses Cookies. View our privacy policy here.