DOI:
10.1039/C3BM60056D
(Paper)
Biomater. Sci., 2013,
1, 745-752
Combining BMP-6, TGF-β3 and hydrostatic pressure stimulation enhances the functional development of cartilage tissues engineered using human infrapatellar fat pad derived stem cells†
Received
1st March 2013
, Accepted 12th March 2013
First published on 26th April 2013
Abstract
The objective of this study was to identify a combination of growth factors that could be used with hydrostatic pressure (HP) stimulation to enhance the functional development of cartilaginous grafts engineered using human infrapatellar fat pad derived stem cells (FPSCs) isolated from osteoarthritic patients. Agarose hydrogels were first seeded with FPSCs at different seeding densities and maintained in a chondrogenic media supplemented with TGF-β3. It was found that chondrogenesis of human FPSCs in hydrogel culture is dependent on the cell seeding density (10 versus 30 million cells per ml), with greater sulphated glycosaminoglycan (sGAG) and collagen synthesis (normalised to DNA content) observed at higher seeding densities. Additional supplementation with BMP-6 was found to augment cartilage-specific matrix synthesis, also in a cell seeding density dependent manner, increasing both cell proliferation and sGAG synthesis in constructs seeded at higher densities, but having no significant effect at lower cell seeding densities. The application of cyclic HP to FPSC seeded constructs cultured in the presence of both TGF-β3 and BMP-6 had no significant effect on DNA content or sGAG accumulation, however it did improve the dynamic modulus of the engineered tissue. These tissues stained strongly for both alcian blue and type II collagen and negatively for type X collagen. The results of this study point to the benefits of combining both biochemical and biophysical stimulation to engineer functional cartilage grafts using diseased human FPSCs.
1. Introduction
Mesenchymal stem cells (MSCs) are increasingly used for engineering functional cartilaginous grafts with the ultimate aim of regenerating damaged and diseased articular cartilage.1 Stem cells isolated from tissues within the joint space, such as synovium2–5 and infrapatellar fat pad,6–12 are particularly attractive for such purposes as they are accessible during arthroscopy and have been shown to possess a strong chondrogenic capacity. While synovium derived stem cells (SDSCs) and infrapatellar fat pad derived stem cells (FPSCs) from skeletally immature animals can be used to engineer functional cartilaginous grafts through hydrogel encapsulation,13–16 we have recently demonstrated that FPSCs from osteoarthritic patients display a diminished chondrogenic capacity upon encapsulation into such hydrogels compared to traditional pellet culture.17 While serum stimulation partially recovered this diminished potential, the mechanical functionality of the grafts was still significantly lower than normal articular cartilage.17 Therefore identifying more optimal combinations of growth factors and biophysical cues is a central challenge in engineering functional cartilage tissues using human joint tissue derived stem cells.
Different cocktails of soluble growth factors and steroids have been explored with the aim of enhancing the development of engineered cartilaginous grafts.18 The combination of either transforming growth factor-β1 or -β3 (TGF-β1 or TGF-β3) and dexamethasone (DEX) has been shown to induce robust chondrogenesis of bone marrow derived MSCs,19–21 with similar findings reported for adipose derived stem cells (ADSCs).22 However not all studies support the use of DEX for stem cell based cartilage tissue engineering. This synthetic glucocorticoid has been shown to suppress chondrogenic differentiation of human SDSCs embedded in alginate hydrogels.23 Similarly it has been shown that DEX reduced glycosaminoglycan accumulation in ADSCs transfected with a bone morphogenetic protein 6 (BMP-6) construct, despite early gene expression data indicating a pro-chondrogenic response to this combined stimulus.24 DEX has also been reported as a non-necessary medium component for the chondrogenesis of human mesenchymal stem cells in polyethylene-glycol hydrogels.25 These results demonstrate that the potential benefits and/or limitations of DEX supplementation must be determined for each combination of cell type and culture condition.
The use of other growth factors, either alone or in combination with TGF-β1 or TGF-β3, has also been explored for cartilage tissue engineering applications. The addition of soluble BMP-6 has been shown to enhance chondrogenesis of both bone marrow derived MSCs and ADSCs,26–29 although it appears particularly important for inducing robust differentiation of the latter. Fibroblast growth factor 2 (FGF-2) is commonly used to enhance the proliferative capacity of MSCs and to help maintain their plasticity during monolayer expansion,30,31 but it has also been shown that the supplementation of chondrogenic media with FGF-2 can either enhance chondrogenesis of ADSCs in a dose dependant manner32 or inhibit chondrogenesis of human bone marrow MSCs.33 Lower concentrations of FGF-2 (10 ng ml−1) enhanced sulphated glycosaminoglycan (sGAG) and collagen II accumulation, although higher concentrations (50 ng ml−1) were not beneficial for chondrogenesis.32 In contrast, supplementation with 10 ng ml−1 FGF-2 has also been shown to suppress COL2A1 and COL10A1 expression.33 The temporal addition of growth factors may also have an important role in enhancing the development of cartilaginous grafts engineered using MSCs. Supplementation with TGF-β1 for the first 7 days of culture is necessary to induce robust chondrogenesis of bone marrow derived MSCs embedded into polyethylene-glycol hydrogels, with subsequent removal of this growth factor enhancing long-term sGAG accumulation.25 Transient exposure to TGF-β3 has been shown to enhance the functional development of cartilage tissues engineered using bone marrow derived MSCs embedded into agarose hydrogels at high cell seeding densities.34
The overall aim of this study was to identify a combination of growth factors that could be used synergistically with hydrostatic pressure stimulation to enhance the functional development of cartilaginous grafts engineered using human FPSCs. The first objective was to systematically investigate how the cell seeding density, as well as the addition of different growth factors, specifically BMP-6, to a serum free chondrogenic media containing TGF-β3 would influence the development of cartilaginous tissues engineered using this cell source in free swelling conditions. Further experiments, either additionally supplementing the media with FGF-2, temporally stimulating constructs with TGF-β3 or completely withdrawing dexamethasone, were also undertaken. Having identified the most beneficial growth factor supplementation regime for engineering cartilaginous grafts using human FPSCs, we further explored if additional stimulation with cyclic hydrostatic pressure would further enhance the functional development of the engineered tissue. This biophysical cue has previously been shown to enhance chondrogenesis of MSCs and the functional development of engineered cartilaginous grafts.17,35–44
2. Materials and methods
2.1 Cell isolation and expansion
Ethical approval for the study was obtained from the institutional review board of the Mater Misericordiae University Hospital with infrapatellar fat pad tissue being obtained from 5 patients with knee osteoarthritis (OA) at joint arthroplasty (1 Male, 4 Female, 50–79 Years old). Fat pad was maintained in sterile phosphate buffered saline (PBS) and transferred immediately to the Trinity Centre for Bioengineering for further processing. Fibrous tissue was carefully removed from the fat pad. Remaining tissue was weighed, washed thoroughly in PBS and diced followed by incubation under constant rotation at 37 °C with high-glucose Dulbecco's Modified Eagle Medium (hgDMEM, GlutaMAX™) (GIBCO, Biosciences, Ireland) containing 1% penicillin (100 U ml−1)–streptomycin (100 μg ml−1) and collagenase type II (4 ml solution g−1 tissue, 750 U ml−1, Worthington Biochemical, LanganBach Services, Ireland) for 4 hours. Cells were filtered through serial cell sieves (Falcon, Sarstedt, Ireland) with pore sizes from 100 μm, 70 μm to 40 μm. The isolated FPSCs were cultured in expansion medium [hgDMEM GlutaMax supplemented with 10% v/v fetal bovine serum (FBS), penicillin (100 U ml−1)–streptomycin (100 μg ml−1) (all from Gibco, Biosciences, Ireland)] supplemented with 5 ng ml−1 fibroblast growth factor-2 (FGF-2) (Prospect-Tany TechnoGene Ltd, Israel). Cells were expanded to passage two (P2).
2.2 Chondrogenesis in agarose hydrogel
FPSCs (P2) were suspended in 2% agarose (type VII; Sigma-Aldrich) at a density of either 10 × 106 or 30 × 106 cells per ml. The agarose cell suspension was cast in a stainless steel mould and cored using a biopsy punch to produce construct cylinders (Ø5 mm × 1.5 mm). Constructs were maintained in chondrogenic medium (CM) consisting of hgDMEM GlutaMax supplemented with penicillin (100 U ml−1)–streptomycin (100 μg ml−1) (Invitrogen, Paisley, UK), 100 μg ml−1 sodium pyruvate, 40 μg ml−1L-proline, 50 μg ml−1L-ascorbic acid-2-phosphate, 4.7 μg ml−1 linoleic acid, 1.5 mg ml−1 bovine serum albumin, 1× insulin–transferrin–selenium, 100 mM dexamethasone (all from Sigma-Aldrich) and 10 ng ml−1 of recombinant human transforming growth factor-β3 (TGF-β3; Prospect-Tany TechnoGene Ltd, Israel). Constructs were maintained in this chondrogenic media with or without the addition of 10 ng ml−1 recombinant human bone morphogenic protein-6 (BMP-6; R&D systems) for 42 days. In a series of parallel experiments, the following additional supplementation conditions to the standard chondrogenic media were also explored: (i) The removal of dexamethasone from the culture media; (ii) the temporal withdrawal of TGF-β3 from the media after 2 weeks of culture; (iii) the additional supplementation with 10 ng ml−1 FGF-2. Each construct was cultured in 2.5 ml of media at 5% O2 for a period of 42 days with media exchanges performed twice weekly. Constructs were assessed at day 0 and 42 in terms of biomechanical functionality, biochemical constituents and histological analysis.
2.3 Chondrogenesis in pellet culture
Pellets were formed from culture-expanded cells by centrifuging 250
000 cells (P2) in 1.5 ml conical microtubes at 650 g for 5 min. Pellets were maintained in chondrogenic medium (described above) with or without the additional supplementation of 10 ng ml−1 BMP-6. Each pellet was cultured in 1 ml of media which was changed twice weekly. Pellets were maintained at 5% O2 and assessed at days 0 and 21.
2.4 Hydrostatic pressure loading
Hydrogels seeded with FPSCs (30 × 106 cells per ml) and maintained in a chondrogenic medium supplemented with 10 ng ml−1 TGF-β3, 10 ng ml−1 BMP-6 and dexamethasone (100 nM) were subjected to hydrostatic pressure at a magnitude of 10 MPa and a frequency of 1 Hz for 4 hours per day, 5 days per week from day 8 to 35 of culture in a custom hydrostatic pressure (HP) bioreactor.35
Cell-seeded constructs (n = 6 for HP loading and n = 6 for free swelling (FS) control) were transferred to sterile, heat-sealed bags containing 15 ml of supplemented media. The bags for HP loading were placed into a water-filled pressure vessel while bags containing free swelling (FS) constructs were placed into an open water bath as a control, both maintained at 37 °C. During the entire loading experiment (day 8–35) constructs were maintained in the sealed bags at 5% O2 with media changed twice weekly. After 5 weeks, all constructs were maintained in free swelling culture for a further 7 days to allow for elaboration of matrix.
2.5 Mechanical analysis
Constructs were mechanically assessed using a protocol described previously.45 Briefly, constructs from each group were tested in unconfined compression between impermeable platens using standard Zwick testing machine with a 5 N load cell (Zwick Z005, Roell, Germany). A ramp and hold cycle with a ramp displacement of 1 μm s−1 until 10% strain was applied and maintained until equilibrium was reached (30 min). At this point, dynamic tests were performed with a cyclic strain amplitude of 1% at 1 Hz. Dynamic moduli were calculated as the ratio of the determined stress amplitude to the applied strain amplitude.
2.6 Biochemical analysis
Gel samples and pellets were digested in papain (125 μg ml−1) in 0.1 M sodium acetate, 5 mM cysteine HCl, 0.05 M EDTA, pH 6.0 (all from Sigma-Aldrich, Ireland) at 60 °C under constant rotation for 18 hours. The DNA content of gels was quantified using the Hoechst Bisbenzimide 33258 dye assay (Sigma-Aldrich, Ireland). Proteoglycan content was estimated by quantifying the amount of sulfated glycosaminoglycan (sGAG) in constructs using the dimethylmethylene blue dye-binding assay (Blyscan, Biocolor Ltd, Northern Ireland), with a chondroitin sulfate standard. Total collagen content was determined by measuring the hydroxyproline content. Samples were hydrolysed at 110 °C for 18 h in concentrated HCL (38%) and assayed using a chloramine-T assay45,46 with a hydroxyproline-to-collagen ratio of 1
:
7.69.47
2.7 Histology and immunochemistry staining
Gels and pellets were fixed in 4% PFA, embedded in paraffin and sectioned (5 micron). Sections were stained with 1% alcian blue 8GX (Sigma-Aldrich) in 0.1 M HCl for sGAG, and picrosirius red for collagen. The deposition of collagen type II and collagen type X were identified by immuno-histochemistry.17 Briefly, sections were quenched of peroxidase activity for 20 min (PBS was used to rinse sections between steps) and treated with 0.25 U ml−1 chondroitinase ABC (Sigma) in a humidified environment at 37 °C for 1 h to enhance permeability of the extracellular matrix by removal of chondroitin sulphate. After incubation with 10% goat serum to block non-specific sites, the primary antibody of mouse monoclonal anti-collagen type II diluted 1
:
100 or mouse monoclonal anti-collagen type X diluted 1
:
500 (Abcam, Cambridge, UK) was applied for 1 h at room temperature. Then the secondary antibody (Anti-Mouse IgG biotin conjugate, Sigma-Aldrich) was added for another hour. Colour was developed using the Vectastain ABC reagent (Vectastain ABC kit, Vector Laboratories, UK) for 45 min and exposure to peroxidase DAB substrate kit (Vector laboratories, UK) for 5 min. Slides were dehydrated through ethanol and xylene and mounted with Vectamount medium (Vector Laboratories, UK). Human ligament and cartilage were included as controls for immuno-histochemistry.
2.8 Statistics
Numerical and graphical results are presented as mean ± standard deviation (3–4 samples). Statistics were performed using R (The R Foundation for Statistical Computing, Vienna, Austria). Groups were analyzed for significant differences using a linear model for analysis of variance with single factor or multiple factors and interactions between these factors also examined. Tukey HSD's tests were used for multiple comparisons. Significance was accepted at a level of p < 0.05.
3. Results
3.1 Chondrogenesis of human FPSCs in agarose hydrogels is dependent on the cell seeding density
To explore the role of the cell seeding density on chondrogenesis of human FPSCs in 3D constructs, agarose hydrogels were seeded with either 10 million or 30 million cells per ml and maintained in a chondrogenic media. Measured sGAG/DNA and collagen/DNA, representative of matrix synthesis on a per cell basis, were higher in constructs seeded at 30 million cells per ml (Fig. 1A). Histological analysis also revealed more intense staining for alcian blue and picrosirius red in constructs seeded at the higher density (Fig. 1B).
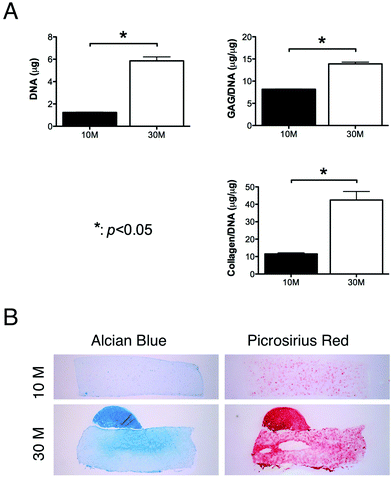 |
| Fig. 1 Effect of cell seeding density on chondrogenesis of FPSCs embedded in agarose hydrogels. FPSCs were seeded at either 10 million cells per ml or 30 million cells per ml. (A) DNA, GAG/DNA and collagen/DNA. (B) Alcian blue staining and picrosirius red staining. | |
3.2 A combination of BMP-6 and TGF-β3 enhances chondrogenesis of human FPSCs
We next explored the effect of BMP-6 in combination with TGF-β3 on chondrogenesis of FPSCs in both pellet culture and following encapsulation in agarose hydrogels. In pellet culture, supplementation with BMP-6 lead to increased proliferation of FPSCs, as evident by a significantly higher DNA content (p < 0.05) after 3 weeks of culture (Fig. 2A). While there was a trend towards higher sGAG (p = 0.06) and collagen (p = 0.06) content in pellets supplemented with BMP-6, and these differences were not significant when matrix accumulation was normalized to DNA content (Fig. 2A). Histological analysis confirmed this finding (Fig. 2B).
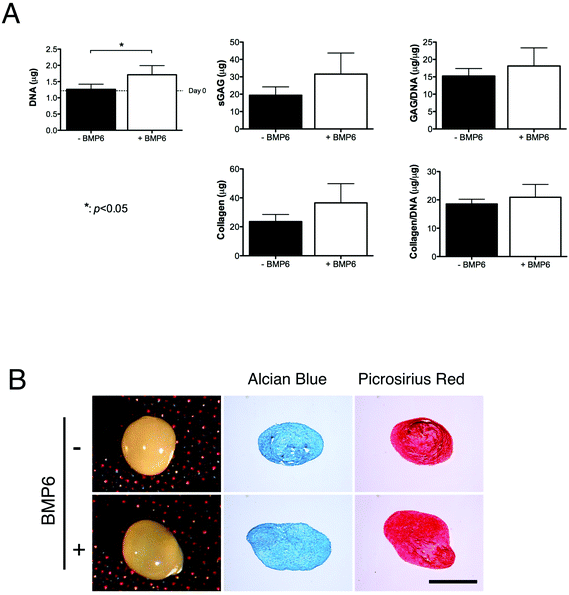 |
| Fig. 2 FPSCs in pellet culture supplemented with TGF-β3 with or without BMP-6. (A) DNA content, sulfated glycosaminoglycan (GAG) content, collagen content, GAG/DNA and collagen/DNA. (B) Pellet morphology, alcian blue staining and picrosirius red staining. “*” indicates a significant difference between FPSCs cultured with or without BMP-6. | |
BMP-6 was found to augment cartilage-specific matrix synthesis in FPSC seeded hydrogels in a cell seeding density dependent manner (Fig. 3A). Supplementation with BMP-6 was observed to increase both DNA (p < 0.01) content and sGAG (p < 0.01) synthesis (sGAG content normalised to DNA content) in constructs seeded at the higher density of 30 × 106 cells per ml, but had no statistical effect on collagen synthesis (Fig. 3). No effect of BMP-6 supplementation was observed at the lower seeding density of 10 × 106 cells per ml. More intense staining for alcian blue was observed in the high cell seeding density constructs supplemented with BMP-6, although staining for type II collagen appeared to be unaffected by the addition of this growth factor. All constructs generally stained weakly for collagen type X.
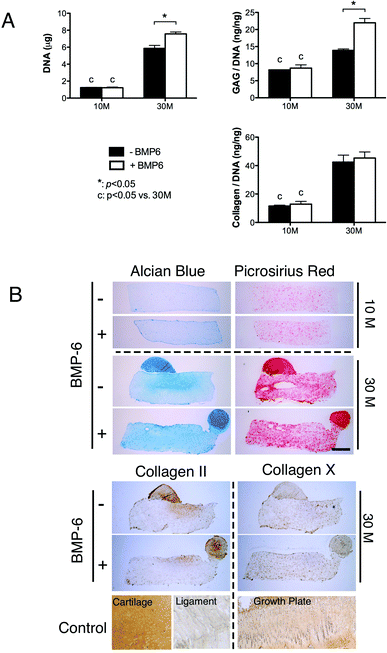 |
| Fig. 3 Effects of BMP-6 and cell seeding density on chondrogenesis of FPSCs embedded in agarose hydrogels and maintained in a chemically defined media supplemented with TGF-β3 and dexamethasone. Constructs were seeded at 10 million cells per ml or 30 million cells per ml. (A) DNA content, GAG/DNA and collagen/DNA. (B) Alcian blue staining, picrosirius red staining, and immuno-staining of collagen II and collagen X. “*” indicates a significant difference between FPSCs cultured with or without BMP-6. “C” indicates a significant difference between FPSCs seeded at 10 million per ml or 30 million per ml. | |
A number of additional supplementation conditions were also explored as part of this study. The removal of dexamethasone, the temporal exposure to TGF-β3 (i.e. withdrawing TGF-β3 from the media after 2 weeks of culture) or the additional supplementation with FGF-2 had either a negative or no positive effect on chondrogenesis (see ESI Figure†), and hence were not further considered as part of this study.
3.3 Hydrostatic pressure enhances the functional development of cartilaginous constructs engineered in the presence of TGF-β3 and BMP-6
The application of cyclic hydrostatic pressure (HP) to FPSC seeded constructs cultured in the presence of TGF-β3 and BMP-6 had no significant effect on DNA content or sGAG accumulation, however it resulted in significantly greater collagen accumulation (p < 0.05) (Fig. 4A). When normalized to DNA content, this difference was no longer significant. Hydrostatic pressure appeared to alter the spatial distribution of matrix within the engineered tissue, with more spatially uniform staining observed in the constructs subjected to HP (Fig. 4C). Both free-swelling (FS) controls and constructs subjected to HP stained positive for type II collagen and weakly for type X collagen. Dynamic mechanical testing revealed that the application of HP leads to a small but significant increase in the dynamic modulus (p < 0.05) of the engineered tissues (Fig. 4B).
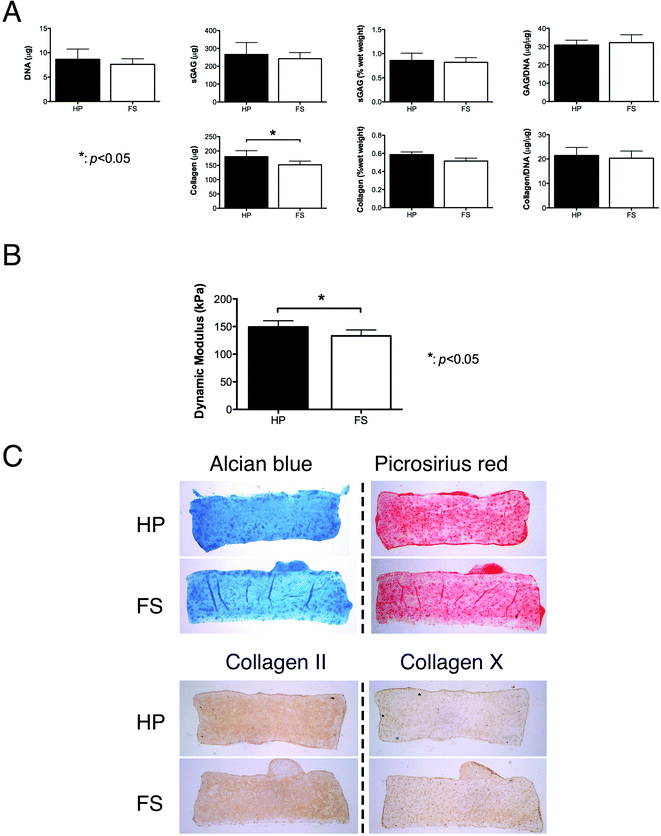 |
| Fig. 4 Effects of hydrostatic pressure on chondrogenesis of FPSCs maintained in agarose hydrogels. Hydrostatic pressure was applied from day 8–35 of a 6-week culture. (A) DNA content, sulfated glycosaminoglycan (sGAG) content, sGAG content per wet weight, GAG/DNA, collagen content, collagen content per wet weight and collagen/DNA. (B) Dynamic modulus. (C) Alcian blue staining, picrosirius red staining, and immuno-staining of collagen II and collagen X. “*” indicates a significant difference between constructs subjected to hydrostatic pressure loading or maintained in free swelling conditions. | |
4. Discussion
Chondrogenic priming of MSCs prior to implantation to generate a more cartilage-like tissue has been shown to improve outcomes in animal model studies of articular cartilage regeneration,48,49 with computational models also pointing to the importance of engineering more functional tissues prior to implantation.50 Translating such findings to treat damaged or diseased articular cartilage in man will most likely require engineering functional tissue grafts using autologous stem cells. The results of this study demonstrate that cartilage-specific matrix synthesis by FPSCs embedded into agarose hydrogels and stimulated with TGF-β3 increases with increasing cell seeding densities. Supplementation with BMP-6 further improved chondrogenesis in this hydrogel system, but in a cell seeding density dependent manner. Finally we demonstrate that this potent combination of growth factors can be combined with cyclic hydrostatic pressure to enhance the functional development of cartilaginous grafts engineered using diseased human FPSCs.
The highest levels of chondrogenesis were observed in constructs continuously stimulated with dexamethasone, TGF-β3 and BMP-6. Dexamethasone is a synthetic glucocorticosteroid that has been shown to promote both the osteogenic and chondrogenic differentiation of stem cells,21,51–53 and to maintain the functional properties of cartilage explants.54 The exact mechanism through which dexamethasone promotes chondrogenesis is unknown, but it appears to act indirectly through the major active form of the GC receptor, GCa, to induce the expression of cartilage matrix genes.55 In this study we found the addition of dexamethasone also facilitated chondrogenesis of human FPSCs embedded in agarose hydrogels, which is in contrast to other studies using alternative cell sources and hydrogel systems.23,25,56,57 It was previously shown that the exclusion of dexamethasone increases early SOX9 gene expression and reduces collagen I deposition for bone marrow derived MSCs encapsulated in a hydrogel, leading to the suggestion that a more hyaline-cartilage is generated without dexamethasone stimulation.25 These contradictory results suggest that the effect of dexamethasone on chondrogenesis of stem cells is specific to the culture system under investigation and/or the specific tissue source of stem cells.
Both GAG and collagen synthesis within the agarose hydrogel was higher at the higher FPSC seeding density. The importance of high cell densities and associated cell–cell interactions has long been appreciated for promoting chondrogenesis of MSCs,19 and it would appear that this would also be the case when engineering functional grafts using diseased human stem cells embedded into hydrogels. A secondary impact of increasing the cell seeding density is that it will lower oxygen availability with the engineered tissue due to increased levels of oxygen consumption. We have previously shown that low oxygen conditions enhances chondrogenesis of MSCs isolated from different sources,13,58,59 and although we maintained all constructs at 5% oxygen, it may be that lower levels of oxygen are optimal for inducing robust differentiation. This could potentially contribute to the enhanced levels of matrix synthesis at higher cell seeding densities, but it is also possible that lower oxygen conditions prime MSCs for stimulation by growth factors like BMP-6.
Similar to a number of studies using subcutaneous fat derived stem cells,29,60 we found that the addition of BMP-6 to a chondrogenic media containing TGF-β3 enhanced chondrogenesis of FPSCs. It has been shown that BMP-6 increases the expression TGF-β-receptor-I and hence primes stem cells for TGF-β mediated chondrogenesis.29 An interesting finding of this study was that BMP-6 only enhanced matrix accumulation at high cell seeding densities, suggesting that cell–cell communication may be also important for BMP-6 mediated improvements in FPSC chondrogenesis. BMP-6 is also known to be a strong inhibitor of hypertrophy/endochondral ossification for ADSCs,28 however we observed little type X collagen production independent of BMP-6 stimulation. This may be a result of our use of low oxygen culture conditions, which has been shown to suppress markers of hypertrophy and endochondral ossification.59
The final phase of this study demonstrated that combining hydrostatic pressure stimulation with TGF-β3 and BMP-6 improves the functional development of cartilaginous tissue engineered using FPSCs. Numerous studies have demonstrated the beneficial effect of cyclic hydrostatic pressure for promoting chondrogenesis of MSCs17,35–44 and in suppressing hypertrophy of the developing tissue.36 Here we observed that the application of HP enhanced the accumulation of collagen within the hydrogel and encouraged the formation of a more homogenous engineered tissue, which was mechanically stiffer than free swelling controls. This is in agreement with previous studies demonstrating that the application of HP can result in the formation of more homogenous and compact tissues which are important for improving mechanical functionality.35 Higher swelling pressures within these more compact tissues may explain these improvements in mechanical properties.61 Taken together, the results of this study point to the need for combinations of biochemical and biophysical stimulation to engineer functional cartilage grafts using diseased human FPSCs.
Acknowledgements
Funding for this study was provided by Irish Research Council for Science, Engineering & Technology under enterprise partner scheme with Sports Surgery Clinic Dublin (IRCSET-SSC-2010-01), a European Research Council Starter Grant (StemRepair – Project number: 258463) and a President of Ireland Young Researcher Award (08/Y15B1336). The authors would like to thank Richard Downey for help with the collection of biological tissue.
References
- M. Stoddart, S. Grad, D. Eglin and M. Alini, Regen. Med., 2009, 4, 81–98 CrossRef CAS.
- C. De Bari, F. Dell'Accio, P. Tylzanowski and F. P. Luyten, Arthritis. Rheum., 2001, 44, 1928–1942 CrossRef CAS.
- Y. Sakaguchi, I. Sekiya, K. Yagishita and T. Muneta, Arthritis. Rheum., 2005, 52, 2521–2529 CrossRef.
- M. Pei, F. He and G. Vunjak-Novakovic, Differentiation, 2008, 76, 1044–1056 CrossRef CAS.
- K. Nishimura, L. A. Solchaga, A. I. Caplan, J. U. Yoo, V. M. Goldberg and B. Johnstone, Arthritis. Rheum., 1999, 42, 2631–2637 CrossRef CAS.
- M. Q. Wickham, G. R. Erickson, J. M. Gimble, T. P. Vail and F. Guilak, Clin. Orthop. Relat. Res., 2003, 196–212 CrossRef.
- J. L. Dragoo, B. Samimi, M. Zhu, S. L. Hame, B. J. Thomas, J. R. Lieberman, M. H. Hedrick and P. Benhaim, J. Bone Joint Surg. Br., 2003, 85, 740–747 CAS.
- A. English, E. A. Jones, D. Corscadden, K. Henshaw, T. Chapman, P. Emery and D. McGonagle, Rheumatology, 2007, 46, 1676–1683 CrossRef CAS.
- W. S. Khan, A. B. Adesida and T. E. Hardingham, Arthritis. Res. Ther., 2007, 9, R55 Search PubMed.
- W. S. Khan, A. B. Adesida, S. R. Tew, J. G. Andrew and T. E. Hardingham, Injury, 2009, 40, 150–157 CrossRef CAS.
- S. O'hEireamhoin, C. T. Buckley, E. Jones, D. McGonagle, K. J. Mulhall and D. J. Kelly, Tissue Eng. Part C, Methods, 2013, 19, 117–127 CrossRef CAS.
- M. Ahearne, C. T. Buckley and D. J. Kelly, Biotechnol. Appl. Biochem., 2011, 58, 345–352 CrossRef CAS.
- C. T. Buckley, T. Vinardell and D. J. Kelly, Osteoarthr. Cartil., 2010, 18, 1345–1354 CrossRef CAS.
- C. T. Buckley, T. Vinardell, S. D. Thorpe, M. G. Haugh, E. Jones, D. McGonagle and D. J. Kelly, J. Biomech., 2010, 43, 920–926 CrossRef.
- T. Vinardell, C. T. Buckley, S. D. Thorpe and D. J. Kelly, J. Tissue Eng. Regen. Med., 2011, 5, 673–683 CrossRef CAS.
- T. Vinardell, E. J. Sheehy, C. T. Buckley and D. J. Kelly, Tissue Eng. Part A, 2012, 18, 1161–1170 CrossRef CAS.
- Y. Liu, C. T. Buckley, R. Downey, K. J. Mulhall and D. J. Kelly, Tissue Eng. Part A, 2012, 18, 1531–1541 CrossRef CAS.
- J. L. Puetzer, J. N. Petitte and E. G. Loboa, Tissue Eng. Part B-Re, 2010, 16, 435–444 CAS.
- J. U. Yoo, T. S. Barthel, K. Nishimura, L. Solchaga, A. I. Caplan, V. M. Goldberg and B. Johnstone, J. Bone Joint Surg. – Series A, 1998, 80, 1745–1757 CAS.
- A. M. Mackay, S. C. Beck, J. M. Murphy, F. P. Barry, C. O. Chichester and M. F. Pittenger, Tissue Eng., 1998, 4, 415–428 CrossRef CAS.
- B. Johnstone, T. M. Hering, A. I. Caplan, V. M. Goldberg and J. U. Yoo, Exp. Cell Res., 1998, 238, 265–272 CrossRef CAS.
- C. Y. Huang, K. L. Hagar, L. E. Frost, Y. Sun and H. S. Cheung, Stem Cells, 2004, 22, 313–323 CrossRef CAS.
- T. Kurth, E. Hedbom, N. Shintani, M. Sugimoto, F. H. Chen, M. Haspl, S. Martinovic and E. B. Hunziker, Osteoarthr. Cartil., 2007, 15, 1178–1189 CrossRef CAS.
- B. O. Diekman, C. R. Rowland, D. P. Lennon, A. I. Caplan and F. Guilak, Tissue Eng. Part A, 2010, 16, 523–533 CrossRef CAS.
- A. N. Buxton, C. S. Bahney, J. U. Yoo and B. Johnstone, Tissue Eng. Part A, 2011, 17, 371–380 CrossRef CAS.
- I. Sekiya, D. C. Colter and D. J. Prockop, Biochem. Biophys. Res. Commun., 2001, 284, 411–418 CrossRef CAS.
- N. Indrawattana, G. Chen, M. Tadokoro, L. H. Shann, H. Ohgushi, T. Tateishi, J. Tanaka and A. Bunyaratvej, Biochem. Biophys. Res. Commun., 2004, 320, 914–919 CrossRef CAS.
- B. T. Estes, A. W. Wu and F. Guilak, Arthritis. Rheum., 2006, 54, 1222–1232 CrossRef CAS.
- T. Hennig, H. Lorenz, A. Thiel, K. Goetzke, A. Dickhut, F. Geiger and W. Richter, J. Cell. Physiol., 2007, 211, 682–691 CrossRef CAS.
- L. A. Solchaga, K. Penick, J. D. Porter, V. M. Goldberg, A. I. Caplan and J. F. Welter, J. Cell. Physiol., 2005, 203, 398–409 CrossRef CAS.
- C. T. Buckley and D. J. Kelly, J. Mech. Behav. Biomed. Mater., 2011, 11, 102–111 CrossRef.
- M. Chiou, Y. Xu and M. Longaker, Biochem. Biophys. Res. Commun., 2006, 343, 644–652 CrossRef CAS.
- S. Weiss, T. Hennig, R. Bock, E. Steck and W. Richter, J. Cell. Physiol., 2010, 223, 84–93 CAS.
- A. H. Huang, A. Stein, R. S. Tuan and R. L. Mauck, Tissue Eng. Part A, 2009, 15, 3461–3472 CrossRef CAS.
- E. G. Meyer, C. T. Buckley, A. J. Steward and D. J. Kelly, J. Mech. Behav. Biomed. Mater., 2011, 4, 1257–1265 CrossRef CAS.
- T. Vinardell, R. A. Rolfe, C. T. Buckley, E. G. Meyer, M. Ahearne, P. Murphy and D. J. Kelly, Eur. Cell. Mater., 2012, 23, 121–134 CAS.
- P. Angele, J. U. Yoo, C. Smith, J. Mansour, K. J. Jepsen, M. Nerlich and B. Johnstone, J. Orthop. Res., 2003, 21, 451–457 CrossRef CAS.
- K. Miyanishi, M. C. Trindade, D. P. Lindsey, G. S. Beaupre, D. R. Carter, S. B. Goodman, D. J. Schurman and R. L. Smith, Tissue Eng., 2006, 12, 2253–2262 CrossRef CAS.
- K. Miyanishi, M. C. Trindade, D. P. Lindsey, G. S. Beaupre, D. R. Carter, S. B. Goodman, D. J. Schurman and R. L. Smith, Tissue Eng., 2006, 12, 1419–1428 CrossRef CAS.
- Z. J. Luo and B. B. Seedhom, Proc. Inst. Mech. Eng [H], 2007, 221, 499–507 CAS.
- D. R. Wagner, D. P. Lindsey, K. W. Li, P. Tummala, S. E. Chandran, R. L. Smith, M. T. Longaker, D. R. Carter and G. S. Beaupre, Ann. Biomed. Eng., 2008, 36, 813–820 CrossRef.
- R. Ogawa, S. Mizuno, G. F. Murphy and D. P. Orgill, Tissue Eng. Part A, 2009, 15, 2937–2945 CrossRef CAS.
- A. J. Steward, S. D. Thorpe, T. Vinardell, C. T. Buckley, D. R. Wagner and D. J. Kelly, Acta Biomater., 2012, 8, 2153–2159 CrossRef CAS.
- A. J. Steward, D. R. Wagner and D. J. Kelly, Eur. Cell. Mater., 2013, 25, 167–178 CAS.
- C. T. Buckley, S. D. Thorpe, F. J. O'Brien, A. J. Robinson and D. J. Kelly, J. Mech. Behav. Biomed. Mater., 2009, 2, 512–521 CrossRef.
- W. Kafienah and T. J. Sims, Methods Mol. Biol., 2004, 238, 217–230 CAS.
- N. Y. Ignat'eva, N. A. Danilov, S. V. Averkiev, M. V. Obrezkova, V. V. Lunin and E. N. Sobol, J. Anal. Chem., 2007, 62, 51–57 CrossRef CAS.
- B. Marquass, R. Schulz, P. Hepp, M. Zscharnack, T. Aigner, S. Schmidt, F. Stein, R. Richter, G. Osterhoff, G. Aust, C. Josten and A. Bader, Am. J. Sports Med., 2011, 39, 1401–1412 CrossRef.
- M. Zscharnack, P. Hepp, R. Richter, T. Aigner, R. Schulz, J. Somerson, C. Josten, A. Bader and B. Marquass, Am. J. Sports Med., 2010, 38, 1857–1869 CrossRef.
- T. Nagel and D. J. Kelly, Tissue Eng. Part A, 2013, 19, 824–833 CrossRef CAS.
- S. L. Cheng, J. W. Yang, L. Rifas, S. F. Zhang and L. V. Avioli, Endocrinology, 1994, 134, 277–286 CrossRef CAS.
- A. Poliard, A. Nifuji, D. Lamblin, E. Plee, C. Forest and O. Kellermann, J. Cell Biol., 1995, 130, 1461–1472 CrossRef CAS.
- B. Zimmermann and R. Cristea, Anat. Embryol. (Berl), 1993, 187, 67–73 CrossRef CAS.
- L. Bian, A. M. Stoker, K. M. Marberry, G. A. Ateshian, J. L. Cook and C. T. Hung, Am. J. Sports Med., 2010, 38, 78–85 CrossRef.
- A. Derfoul, G. L. Perkins, D. J. Hall and R. S. Tuan, Stem Cells, 2006, 24, 1487–1495 CrossRef CAS.
- Y. Miyazaki, T. Tsukazaki, Y. Hirota, A. Yonekura, M. Osaki, H. Shindo and S. Yamashita, Osteoarthr. Cartil., 2000, 8, 378–385 CrossRef CAS.
- H. A. Awad, Y. D. Halvorsen, J. M. Gimble and F. Guilak, Tissue Eng., 2003, 9, 1301–1312 CrossRef CAS.
- E. G. Meyer, C. T. Buckley, S. D. Thorpe and D. J. Kelly, J. Biomech., 2010, 43, 2516–2523 CrossRef.
- E. J. Sheehy, C. T. Buckley and D. J. Kelly, Biochem. Biophys. Res. Commun., 2012, 417, 305–310 CrossRef CAS.
- F. Hildner, A. Peterbauer, S. Wolbank, S. Nurnberger, S. Marlovits, H. Redl, M. van Griensven and C. Gabriel, J. Biomed. Mater. Res. A, 2010, 94, 978–987 Search PubMed.
- T. Nagel and D. J. Kelly, J. R. Soc. Interface, 2012, 9, 777–789 CrossRef.
Footnote |
† Electronic supplementary information (ESI) available. See DOI: 10.1039/c3bm60056d |
|
This journal is © The Royal Society of Chemistry 2013 |
Click here to see how this site uses Cookies. View our privacy policy here.