DOI:
10.1039/C2FO30129F
(Paper)
Food Funct., 2013,
4, 121-129
In vitro lipid digestion of chitin nanocrystal stabilized o/w emulsions
Received
11th June 2012
, Accepted 25th September 2012
First published on 27th September 2012
Abstract
Chitin nanocrystals (ChN) have been shown to form stable Pickering emulsions. These oil-in-water emulsions were compared with conventional milk (whey protein isolate, WPI, and sodium caseinate, SCn) protein-stabilized emulsions in terms of their lipid digestion kinetics using an in vitro enzymatic protocol. The kinetics of fatty acid release were evaluated as well as the change in oil droplet size of the respective emulsions during lipid digestion. The interfacial pressure was measured by addition of the duodenal components using drop tensiometry and the electrical charge of the oil droplets was also assessed, in an attempt to relate the interfacial properties with the stability of the emulsions towards lipolysis. Lipid hydrolysis in the ChN-stabilized emulsion was appreciably slower and the plateau values of the total concentration of fatty acids released were much lower, compared to the WPI- and SCn-stabilized emulsions. Moreover, the ChN-stabilized emulsions were relatively stable to coalescence during lipid digestion, whereas the WPI- and SCn-stabilized emulsions exhibited a significant increase in their droplet size. On the other hand, no major differences were shown among the different emulsion samples in terms of their interfacial properties. The increased stability of the ChN-stabilized emulsions towards lipolysis could be attributed to several underlying mechanisms: (i) strong and irreversible adsorption of the chitin nanocrystals at the interface that might inhibit an extensive displacement of the solid particles by bile salts and lipase, (ii) network formation by the nanocrystals in the bulk (continuous) phase that may reduce lipid digestion kinetics, and (iii) the ability of chitin, and consequently of ChNs, to impair pancreatic lipase activity. The finding that ChNs can be used to impede lipid digestion may have important implications for the design and fabrication of structured emulsions with controlled lipid digestibility that could provide the basis for the development of novel products that may promote satiety, reduce caloric intake and combat obesity.
1 Introduction
The design of colloidal delivery systems to control the rate and extent of lipid digestion within the gastro-intestinal tract has received extensive attention lately. Inhibiting or slowing down lipid digestion is considered to be an effective means to reduce appetite and promote satiety,1,2 resulting in obesity reduction and a more balanced energy intake.1 Moreover, it can aid towards increased bioavailability of highly lipophilic bioactive components, or their effective delivery to specific sites in the gastro-intestinal (GI) tract. Therefore, these delivery systems may lead to the manufacturing of foods that could combat some of the most frequent chronic human diseases, i.e. obesity, cancer, diabetes, hypertension and heart disease.3–5
Many review articles have recently shown that emulsion structure and stability can play an important role in lipid digestion and absorption.6–8 More specifically, it has been shown that the initial properties of oil-in-water (o/w) emulsions may affect the rate and extent of lipid digestion.9–12 It has also been established that management of the lipid digestion process can be achieved by tuning interfacial characteristics, such as interfacial tension, thickness, elasticity, and composition, as well as by cross-linking of the components forming the interfacial layer.13–15 These parameters can be controlled by using techniques such as electrostatic layer-by-layer deposition,16–20 or using highly surface-active surfactants (galactolipids) that prevent other surface-active components within the GI tract (e.g., bile and lipase) from adsorbing into the lipid droplet interfaces.21 These studies suggest that proper modification of the oil–water interfaces can be used as a strategy to inhibit lipid digestion. In the same context, emulsions that remain stable under harsh environmental conditions could also exhibit enhanced resistance throughout the actual lipid digestion process. For example, systems that display improved physical stability are solid particle-stabilized emulsions or so-called Pickering emulsions.22 These emulsions are stabilized by solid particles that accumulate at the oil–water interface in the form of a densely packed layer, which prevents droplet flocculation and coalescence via steric stabilization.23,24 In most cases, the energy of desorption per particle is predicted to be in the order of several thousand kT,24,25 indicating that once the particles are at the interface, they are effectively and irreversibly adsorbed. Consequently, one of the most outstanding characteristics of the particle-stabilized emulsions is that they are extremely stable to coalescence even when the droplets are quite large.26,27 In addition to the formation of a densely packed layer around the droplets, some other mechanisms responsible for the prevention of droplet coalescence have been proposed for Pickering emulsions. One of these mechanisms is aggregation of the solid particles, where the particle-based barrier is not a simple densely packed layer, but an interconnected network of particles, forming aggregates held together by attractive inter-particle forces.27
Although there is a lot of research on particle-stabilized emulsions, few studies are related to foods,24 which has hampered the use of these emulsions in real food applications. Natural biopolymers such as polysaccharides, can indeed be an attractive source of particulate material (particle emulsifiers) for stabilizing food oil-in-water emulsions. One example is chitin, a structural biopolymer found in shellfish, insects and microorganisms, which is the second most abundant polysaccharide in nature. It is known that acid-hydrolyzed chitin preparations spontaneously disperse into rod-like crystalline particles of nanodimensions.28–31 At low pH, these chitin nanocrystals exhibit positive charges at their surfaces due to protonation of the amino groups.32 It has also been shown that such colloidal dispersions of acid-hydrolysed chitin can undergo an isotropic–nematic transition when their solid concentration is increased.28–31 This transition is manifested by the formation of a nematic gel-like structure, with its properties being affected by solid particle concentration, ionic strength, pH, temperature28 as well as by addition of whey proteins.33 The ChNs have been recently found to stabilize oil-in-water emulsions against coalescence and at certain concentrations against creaming over long periods of time.26
To the best of our knowledge, the potential of Pickering emulsions to restrain lipid digestion has not been explored. Therefore, the objective of the current study was to compare the behavior of o/w emulsions stabilized by chitin nanocrystal (ChN) particles with some “common” milk protein-stabilized emulsions using an in vitro lipid digestion protocol, and to relate their interfacial, physicochemical, and structural properties to the lipid digestion characteristics. For that reason, particle size characterization by static light scattering, z-potential measurements, microscopic observations and interfacial characterization by drop tensiometry have been carried out before and after the in vitro digestion procedure. Additionally, the effect of initial droplet size of the ChN particle-stabilized emulsions on lipid digestion was studied, since the rate of lipid digestion (fatty acids released per minute) has been shown to increase with decreasing droplet size and thereby an increase in the droplet surface area.34 In summary, this study has attempted to elaborate whether there is any relation between the enhanced physical stability of the solid particle-stabilized emulsions and the potential for higher endurance towards lipid hydrolysis. Some important implications of this work could be the design of structured emulsions with controlled lipid digestibility, suitable for the development of novel low-calorie products that promote satiety and combat obesity.
2 Materials and methods
2.1 Materials
Crude chitin from crab shells was obtained from Sigma Chemicals (St Louis, MO). Whey protein isolate (WPI BiPRO) and sodium caseinate were obtained from Davisco Foods International (Le Sueur, Minessota, USA). Hydrochloric acid (concentrated 37% v/v), sodium acetate, glacial acetic acid, potassium hydroxide, sodium chlorite and sodium hydroxide were all of reagent grade and purchased from Sigma Chemicals (St Louis, MO, USA). Calcium chloride (CaCl2·2H2O) was obtained from Fisher Scientific International Inc. Sunflower oil was obtained from a local supermarket and used without further purification, apart from the interfacial measurements where it was purified by using an active carbon-treated filter (Carbograph Extract, no. 210121, IL 60015) to remove any surface active contaminants. Bile extract (porcine, B8631) and lipase from porcine pancreas (activity 2.0 USP units mg−1, Type II, L3126) were obtained from Sigma-Aldrich (St Louis, MO, USA). The composition of bile extract (BS, batch# 058K0066, Sigma-Aldrich) has been reported by the company to consist of: total bile salt (BS) content 49 wt%; 10–15% glycodeoxycholic acid, 3–9% taurodeoxycholic acid, 0.5–7% deoxycholic acid, 1–5% hyodeoxycholic acid, and 0.5–2% cholic acid; 5 wt% phosphatidyl choline (PC); Ca2+ <0.06 wt%; CMC of bile extract at ∼0.07 ± 0.04 mM; the molar ratio of BS to PC being around 15
:
1.12 Type II lipase also contains amylase and protease, in addition to lipases; it has been reported that lipase activity is 100 to 400 units mg−1 protein when using olive oil, and 30–90 units mg−1 protein when using triacetin for an incubation period of 30 min.35 All solutions were prepared with Millipore water (Millipore Corporation, Billerica, Massachusetts, USA).
Aqueous stock dispersions of chitin nanocrystals (ChN) were prepared by acid hydrolysis (3.0 M HCl, 95 °C, 90 min) of the original raw material of crude chitin from crab shells. Detailed information on bleaching (with sodium chlorite) and acid hydrolysis are given elsewhere.28 The solid chitin content of the stock dispersion was determined gravimetrically by drying the samples at 50 °C until a constant weight was obtained; the total solids content of the stock dispersion was approximately 2.7% w/w. The final pH of the stock ChN dispersion was adjusted to 3.0 with 1.0 M HCl.
2.3 Emulsion preparation
Oil-in-water emulsions were prepared by mixing appropriate quantities of the ChN stock dispersion, sunflower oil and an aqueous solution adjusted to pH 3.0 with HCl solution, using an ultrasonic homogenizer (Branson Sonifier 250, 24 kHz, Danbury, USA) for 2 min, operated at 20 s intervals to avoid overheating of the samples. The same procedure was applied for the whey protein isolate (WPI) emulsion system. The sodium caseinate emulsions (SCn) were instead prepared at a pH of 7.0. The concentration of the sunflower oil was always adjusted to 10% w/w. The concentration of SCn and WPI in the 10% w/w oil-in-water emulsions was 0.5% w/w, whereas that of ChN varied within 0.03 and 0.5% w/w. All ingredient concentrations (oil and emulsifiers) refer to the whole emulsion and not just to the aqueous phase.
2.4
In vitro lipid digestion model
The in vitro digestion model used in this study was a modification of those described previously.11,12 The original emulsions (10% w/w sunflower oil) were diluted with their respective continuous phase (e.g. aqueous solution of pH 3.0 for ChN and WPI or pH 7.0 for SCn) as to obtain a final emulsion of 0.5% w/w oil. Portions of 30 mL of the latter diluted emulsion were incubated in a water bath at 37.0 °C for 10 min and subsequently the pH was adjusted to 7.0 using NaOH solutions. Then, 5.0 mL of bile extract solution (187.5 mg bile extract dissolved in phosphate buffer 10 mM, pH 7.0, 37 °C) and 1.0 mL of CaCl2 solution (187.5 mM CaCl2 in double distilled water, 37 °C) were added to the samples under continuous stirring and the system was adjusted back to pH 7.0 if required. A small amount of 1.5 mL freshly prepared pancreatic lipase suspension (60 mg pancreatic lipase powder dispersed in 1.5 mL phosphate buffer, pH 7.0, 37 °C) was added to the above mixture. The final composition of the reaction mixture in the vessel was 150 mg lipid, 5 mg mL−1 bile extract, 1.6 mg mL−1 pancreatic lipase, and 5 mM CaCl2. A pH-stat automatic titration unit (Metrohm, USA Inc.) was employed to automatically monitor the pH continuously during lipid digestion and to maintain the pH at 7.0 by titrating with 0.1 M NaOH solution to neutralize any free fatty acids (FFAs) released. The volume of 0.1 M NaOH added to the samples was recorded, and used to calculate the concentration of free fatty acids generated by lipolysis. The percentage of free fatty acids released was calculated from the number of moles of NaOH required to neutralize the FFA divided by the number of moles of FFA that could be produced from the triacylglycerols if they were all digested (assuming that 2 FFAs are released per triacylglycerol molecule by the lipase action): |  | (1) |
where VNaOH is the volume of sodium hydroxide required to neutralize the FFA produced (in mL), MNaOH is the molarity of the sodium hydroxide solution used (in M), wlipid is the total weight of oil initially present in the reaction vessel (0.15 g), and MWlipid is the average molecular weight of the oil (based on an average fatty acid composition of sunflower oil; an estimate of 880 g mol−1 for the molecular weight was thus obtained). The %FFA values reported herein are actually the %FFA detected and do not necessarily reflect the total amount of FFA liberated, since some of the FFAs released are not soluble enough to be detected with the titrimetric assay.
The kinetics of the FFA release was also presented by plotting log(100/100 − %FFA) vs. time (t), assuming a pseudo first-order reaction model. Linear regression analysis showed a two-stage process, typical of heterogeneous reaction mixture kinetics. The apparent first order rate constants were calculated using the equation:36
| 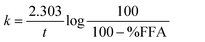 | (2) |
in which
k is the initial lipolysis rate constant.
2.5 Visual assessment and microscopy
In order to evaluate the general appearance of the emulsions and attributes like turbidity, the presence of an oil layer, sediment, etc., after the in vitro digestion procedure, the samples were placed in glass tubes and the respective images were obtained. Optical micrographs of the emulsions before and after the digestion process were captured by an Olympus BX 51 optical microscope fitted with a digital camera (Olympus, DP 50).
2.6 Emulsion droplet-size and zeta-potential measurements
Droplet-size distributions of the emulsions were determined using a Mastersizer 2000 (Malvern Instruments, Malvern, UK). Emulsion droplets were characterized under high dilution conditions by dispersing the samples in their appropriate buffer solutions. The refractive indices of water and sunflower oil were taken as 1.330 and 1.47, respectively, and the Mie theory was used for the analysis. Average droplet sizes were characterized in terms of the volume mean diameter d43 = ∑inidi4/∑inidi3, where ni is the number of droplets of diameter, di. The d43 parameter is a useful mean diameter value and it is more sensitive to the presence of large droplets. All measurements were made at ambient temperature on at least three separately prepared samples.
The zeta-potential of the emulsion droplets was measured with a Zetasizer Nano apparatus (Malvern Instruments, Ltd. Worcestershire, U.K.). Part of the 10% w/w stock emulsions or the digested samples was diluted by a factor of 100 with phosphate buffer (10 mM, pH 7.0). The zeta-potential was calculated from the particle mobility values by applying the Smoluchowski model. The average of five recordings on at least two separate freshly prepared samples is reported as the zeta-potential.
2.7 Measurement of the dynamic surface properties
The interfacial tension as a function of time was monitored using a drop tensiometer (ADT, ITCONCEPT, Longessaigne, France), where a purified sunflower oil droplet (7 μL) was allowed to form on the tip of a needle in an aqueous solution containing either ChN (0.02% w/w) or WPI (0.01% w/w). The temperature was kept constant at 37 °C. The method is described in detail elsewhere.37 When a relatively steady surface pressure was obtained (∼4000 s), the continuous phase was exchanged manually with an aqueous bile extract solution (1 mg mL−1 bile extract, pH 7.0). All results are presented as interfacial pressure, Π = γo − γ, where γo is the interfacial tension of the oil–water interface (in this case 29 mN m−1 for purified sunflower oil) and γ is the measured interfacial tension. All experiments were performed at least in duplicate, with differences between the measurements being within 5–11% of the mean reported value.
3 Results and discussion
3.1 General appearance of the emulsions
The general appearance of the emulsions, initially stabilized by all three types of emulsifiers, namely WPI and SCn, as two conventional dairy protein emulsifiers, and ChN as solid particle emulsifier, and subjected to lipid digestion, was evaluated (Fig. 1). Initially, the emulsions were only treated with the respective buffer solution (to dilute the initial emulsions at 1
:
20, v/v), without adding any bile salts and lipase (Fig. 1A), and it was noticed that all samples showed phase separation with a small creamy layer on the top. For the emulsion initially stabilized by ChN, the serum layer was almost transparent, indicating that most of the oil droplets were located in the cream layer probably due to extensive flocculation. The samples shown in Fig. 1B are the respective diluted emulsions following lipid digestion. The emulsions stabilized by WPI and SCn presented an oil layer at the top, that could be attributed to the destabilization of these systems by the digestion components, causing extensive droplet coalescence. On the other hand, the sample initially stabilized by ChN did not present any oil layer formation, indicating that it might be more stable during lipid digestion. Moreover, all the samples showed precipitates possibly due to the presence of calcium ions in the digestion medium that promote precipitation of anionic components, such as bile salts and free fatty acids. However, in the case of the ChN emulsion, a bigger precipitate is noticed, possibly due to the ChN network formation. It is known that under certain conditions of pH and salt concentration gel formation in ChN aqueous dispersions is fostered;28 the lipid digestion protocol used in this study does involve such conditions (pH = 7.0, CaCl2 addition).
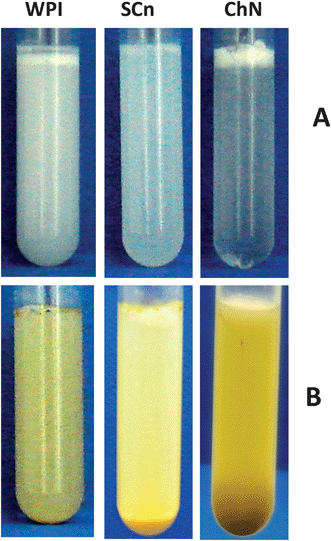 |
| Fig. 1 General appearance of diluted 1 : 20 (v/v) oil-in-water emulsions (oil 10% w/w) initially stabilized with 0.5% w/w ChN, 0.5% w/w WPI or 0.5% w/w SCn (A) after 2 h following the addition of the buffer solution (phosphate buffer 10 mM, pH 7.0, 5 mM CaCl2), and (B) after 2 h following incubation with the duodenal components: lipase (1.6 mg mL−1) and bile extract (5 mg mL−1) (at T = 37 °C, 5 mM CaCl2, pH 7.0). | |
3.2 Size of lipid droplets
The impact of lipid digestion of sunflower oil-in-water emulsions on their droplet size was also explored. The mean particle diameter of the emulsions was measured before and after they were subjected to the in vitro lipid digestion model (Fig. 2). Before digestion, the mean droplet diameter was fairly similar (d43 = 5–7 μm) for all the samples. After digestion, the ChN-stabilized emulsions presented only a slight increase in their particle size (d43 = 9 μm), pointing to the extreme stability toward coalescence of this system. It has been reported that the ChN-stabilized emulsions are particularly stable toward coalescence26 and this is also reflected in their resistance under duodenal digestion conditions. In contrast, there was a dramatic increase in the mean particle diameter of the lipid droplets of the WPI- and SCn-stabilized emulsions compared to the same samples analyzed before digestion; i.e. the d43 value increased from around 6 μm to approximately 70 μm (Fig. 2). The substantial increase in the mean particle diameter of the milk protein-stabilized emulsions indicates extensive droplet coalescence which could originate from the displacement of the proteins by the bile salts and the lipase components on the interface, promoting in this way the production of free fatty acids (FFAs) and mono-acylglycerols (MAG) at the droplet surfaces upon lipid hydrolysis by pancreatic lipase, since these lipophilic (low HLB number) surface active substances are ineffective at stabilizing oil-in-water emulsions against coalescence.38 Similar results for WPI- and SCn-stabilized emulsions under comparable digestion conditions have been reported elsewhere.12,39
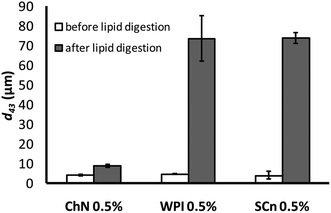 |
| Fig. 2 Mean droplet diameter (d43) of emulsions stabilized by ChN (0.5% w/w), WPI (0.5% w/w) and SCn (0.5% w/w), before and after the in vitro lipid digestion, with lipase (1.6 mg mL−1) and bile extract (5 mg mL−1, at T = 37 °C, 5 mM CaCl2, pH 7.0, hydrolysis time 2 h). | |
In addition, optical micrographs of the emulsions were obtained in an attempt to relate the microstructure with the light scattering results. Fig. 3 shows the effect of in vitro lipid digestion on the microscopic appearance of o/w emulsions. Superimposed on each micrograph is the droplet size distribution determined by light scattering. The microstructure of the ChN-stabilized oil droplets remained almost similar after the in vitro lipid digestion, compared to the sample before the digestion, apart from some aggregated droplets, which are also identified in the respective light scattering curve. In contrast, both the WPI- and SCn-stabilized emulsions showed remarkable differences in their microstructure following the in vitro lipid digestion processing. More specifically, larger particles were formed which were either flocs and/or large individual oil droplets, suggesting that both flocculation and coalescence occurred in these systems. The microstructural features are consistent with the light scattering measurements (Fig. 3) where the milk protein-WPI and SCn-stabilized systems showed droplet size distributions with higher average values, after the lipid digestion treatment.
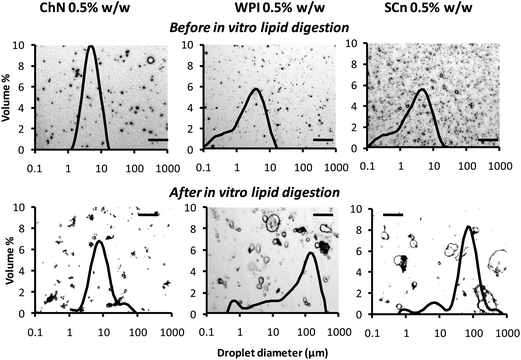 |
| Fig. 3 Typical optical micrographs combined with their respective oil droplet size distributions obtained by static light scattering experiments of ChN-, WPI- and SCn-stabilized o/w emulsions before lipid digestion (after dilution of 1 : 100) and following lipid digestion without further dilution: lipase (1.6 mg mL−1) and bile extract (5 mg mL−1) (at T = 37 °C, 5 mM CaCl2, pH 7.0, hydrolysis time 2 h); scale bar of 50 μm in all frames. | |
3.3 Interfacial pressure
Fig. 4 shows the change in interfacial pressure over time for oil–water systems containing the two different emulsifiers, ChN and WPI, at pH 3.0, before and after continuous phase exchange (approx. after 4000 s) with the bile extract solution (1 mg mL−1, pH 7.0, 37 °C). Initially, the interfacial pressure increased, which indicated that the emulsifiers adsorbed at the oil–water interface. After about 4000 s, a rather constant value was attained, implying that the interface had become saturated with the emulsifier molecules or particles, reaching a rather steady-state composition. For both systems, containing ChN and WPI, there was a steep increase in surface pressure when the continuous phase was exchanged with the bile extract solution, suggesting that bile salts adsorb at the oil–water interface of these systems. Moreover, the interfacial pressure of the oil-in-water interface containing WPI exhibited a slight tendency to increase over time after the exchange of the continuous phase; this implies a continuing rearrangement of the protein molecules at the interface and their possible displacement of by bile salts. At the same time, the respective interfacial pressure of the ChN system was fairly constant after the continuous phase exchange, indicating that a rather steady-state condition had been reached. However, none of the samples reached the value of interfacial pressure exerted by the bile extract alone, implying that the respective interfaces were not completely covered by bile extract components throughout the testing period.
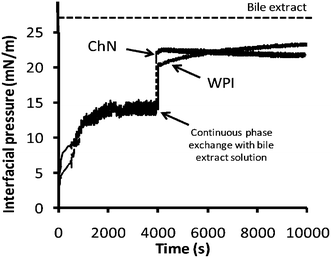 |
| Fig. 4 Development of interfacial pressure over time at oil–water interfaces stabilized by ChN (0.02% w/w) or WPI (0.02% w/w) at pH 3.0 and after continuous phase exchange at 4000 s by bile extract (solution of 1 mg mL−1 at pH 7.0). The dotted line represents the plateau interfacial pressure (∼26.5 mN m−1) reached by the bile extract solution alone at the oil–water interface. | |
3.4 Emulsion droplet surface charge
The z-potential of the lipid droplets provides indirect information about changes in interfacial composition due to in vitro duodenal digestion. The effects of duodenal components on the droplet charge of sunflower o/w emulsions stabilized by ChN and WPI were evaluated. The droplets stabilized by ChN or WPI (blanks) were −1.6 ± 1.0 and −20.1 ± 1.0 mV, respectively (Fig. 5). In both systems, the addition of duodenal components (pancreatic lipase, bile extract, etc.) resulted in an enhancement of the negative surface charge of the emulsion droplets (Fig. 5). These results suggest that at least some lipase and bile extract molecules were adsorbed onto the oil droplet surfaces stabilized by all the emulsifiers. These components could have displaced, interpenetrated or adsorbed on top of the already existing surface-active molecules or particles at the interfaces. However, in the case of ChN-stabilized emulsions, as already discussed, it is less likely that the ChNs are displaced due to their strong adsorption (high binding energy of desorption) at the interface. It is worth mentioning that the increase in negative charge of oil droplets when the bile extract and lipase components were added would have been expected to increase the electrostatic repulsion between the oil droplets, therefore reducing the tendency of droplet aggregation and coalescence. However, this process of component displacement at the interfaces is not spontaneous. Nevertheless, gradual displacement of the WPI molecules by the bile extract components in conjunction with the calcium ions may promote droplet flocculation through charge neutralization effects. In addition, the bile salts, lipid digestion products and the lipase are considerably smaller molecules and thus do not provide a significant steric barrier to the oil droplets, which may further enhance coalescence.
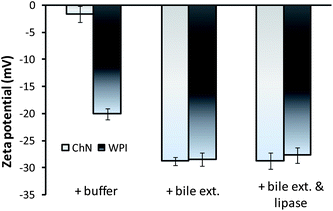 |
| Fig. 5 Electrical charge of emulsion droplets (z-potential) in ChN- and WPI-stabilized o/w emulsions after 2 h of adding buffer solution (blank), 5 mg mL−1 bile extract, or the mixture of bile extract and lipase 1.6 mg mL−1 (at T = 37 °C, 5 mM CaCl2, pH 7.0). | |
3.5
In vitro lipid digestion
The activity of pancreatic lipase in the presence of bile salts was quantified in terms of the percentage of FFAs released from the emulsions during 1 h of hydrolysis using a pH-stat method (Fig. 6a). As a general trend, the percentage of FFA liberated increased rapidly at first, but then reached a pseudo-plateau value after about 50 min, corresponding to the maximum (apparent) concentration of fatty acids released. Milk proteins have been studied extensively for their ability to stabilize o/w emulsions and it has been shown that these colloidal systems were not particularly resistant to lipid hydrolysis, with the WPI exhibiting higher resistance to lipolysis compared with SCn emulsions.12,39 It is obvious from the data of Fig. 6a, that the ChN-stabilized emulsions underwent a considerably lower degree of lipid digestion compared to the WPI and SCn-stabilized emulsions; e.g. the maximum % of FFA released, after 1 h of digestion was approximately 33% in the case of the ChN-stabilized emulsions, while the two protein-stabilized emulsions, WPI and SCn, yielded two-fold higher values, i.e. 58 and 66% FFA, respectively. In order to estimate the initial lipolysis rate, the data of the first stage of the lipolysis process were also presented by plotting log(100/100 − %FFA) vs. time (t). Linear regression analysis showed a good fit of the experimental data with a first order type of process and the apparent first order rate constants were calculated using eqn (2) (Fig. 6b). It was noticed that the ChN-stabilized emulsion exhibited a significantly lower value for the initial digestion rate compared to the two milk protein-stabilized emulsions (inset Fig. 6b). Therefore, ChN was shown ro be quite effective in retarding lipid digestion when compared with the WPI- and SCn-stabilized emulsions.
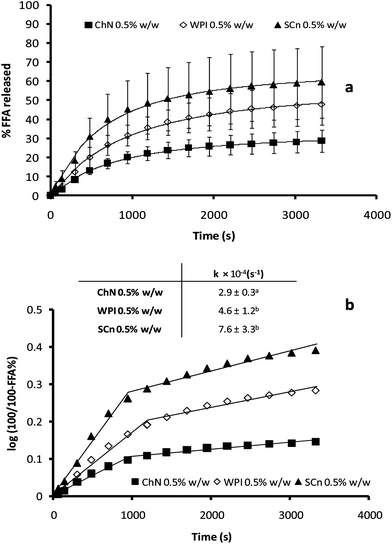 |
| Fig. 6 (a) Time dependence of FFA release (FFA%) from sunflower o/w emulsions stabilized by ChN (0.5% w/w), WPI (0.5% w/w) and SCn (0.5% w/w), after adding the mixture of lipase (1.6 mg mL−1) and bile extract (5 mg mL−1; at T = 37 °C, 5 mM CaCl2, pH 7.0); (b) FFA release data plotted as log(100/100 − %FFA) vs. time. The inset shows the respective apparent rate constants for the initial stage of the lipolysis curve as calculated from eqn (2). | |
Since droplet size has been reported as a parameter affecting lipid digestion kinetics,40 the influence of the ChN concentration on lipid hydrolysis was further explored. It has been found that emulsions stabilized by lower concentrations of ChNs have bigger droplet sizes, since there are not enough ChN particles to stabilize larger interfacial areas.26 In Fig. 7, emulsions with three different concentrations of ChN and consequently possessing different initial droplet sizes (inset Fig. 7) are compared for their FFA release kinetics. Apparently, no actual differences in the rate or the extent of digestion were noted among these preparations which reached similar plateau hydrolysis values (Fig. 7). Therefore, for these systems, the lipid digestion kinetics do not seem to be affected by the initial oil droplet size.
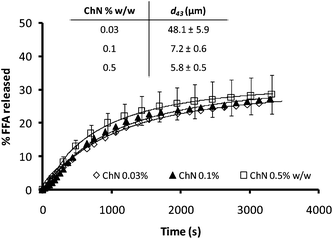 |
| Fig. 7 Time dependence of FFA release (FFA%) from sunflower o/w emulsions stabilized by different concentrations of ChN, following the addition of the mixture of lipase (1.6 mg mL−1) and bile extract (5 mg mL−1; at T = 37 °C, 5 mM CaCl2, pH 7.0). The inset shows the respective d43 values for the ChN-stabilized emulsions. | |
In general, the process of lipid digestion includes the action of pancreatic lipase on triglycerides in order to release more soluble and absorbable constituents such as fatty acids. The lipase has to adsorb on the surface of the oil droplet, increasing the contact between the enzyme and substrate. A number of constituents exist in the human digestive environment which could enhance the ability of lipase to adsorb onto lipid droplet surfaces. Bile salts and phospholipids are also known to displace other surface active components and thereby solubilize the digestion products; calcium is needed for enzyme activity and to remove long chain fatty acids from the droplet surfaces; furthermore, co-lipase assists lipase to adsorb onto the lipid droplet surfaces.41–43 Hence, in order to retard lipid digestion, bile salts should be inhibited to adsorb onto the oil–water interface,1 and either lipase or the other essential duodenal components activity must be reduced.
Generally, proteins do not form densely packed adsorbed layers on oil–water interfaces, and therefore they can easily be displaced by small molecular weight surfactants to create defects in the protein network at the interface.1 Furthermore, adsorbed proteins are susceptible to hydrolysis by proteases, both in the stomach and the small intestine; proteolysis can therefore weaken the protein network and increase displacement of the protein molecules by small molecular weight surfactants and bile salts.44,45 Instead, the ChN-stabilized emulsions did show much lower digestion levels, which may be explained by a number of physicochemical phenomena. It is well established that in the case of Pickering or particle-stabilized emulsions, the solid particles are effective and irreversibly adsorb at the interfaces, since the energy of desorption is of the range of several thousand kT.24,25 Additionally, such systems have been found to be particularly stable in time and under different environmental conditions (e.g. pH, ionic strength, etc.)26 The ChN-stabilized oil-in-water emulsions indeed exhibit extreme stability toward coalescence, even when the droplet sizes are considerably large (approx. 100 μm);26 in the present work, the ChN-stabilized emulsions were particularly resistant to coalescence even under the in vitro lipid digestion conditions employed (Fig. 2 and 3). Moreover, the ChN particles have been proposed to form a network at the oil–water interface that provides a strong mechanical barrier around the droplets. At high concentrations, the ChNs undergo an isotropic to nematic phase transition, which originates from their ability to form highly ordered and densely packed network structures in an aqueous environment. The bile salts, being small molecules, could possibly pass through this ordered network and adsorb at the interface, as revealed from the interfacial and z-potential measurements (Fig. 4 and 5), but it is likely that the extensive displacement of ChN by the bile salts does not take place, compared to adsorbed milk proteins, due to the very strong binding of the nanocrystals and their rigid structure (stiff rod-like particles unable to undergo conformational changes). The stability of this system to coalescence under the in vitro lipid digestion protocol (Fig. 2 and 3) is also an indication of the strong adherence of the ChNs at the interface and their resistance to displacement by the duodenal components. The formation of a steric bulky layer with a considerable thickness and ordering of the ChN network at the interface may impede the adsorption and penetration of the lipase–co-lipase enzyme system. Such a steric mechanism has been proposed before in the literature by Chu et al.21 for galactolipids and by Maldonado et al.40 for Pluronic surfactant.
Another reason for the reduced digestion kinetics might be the formation of a ChN network in the bulk phase which mechanically entraps the lipid droplets and thereby decreases their accessibility to bile salts or lipase. Even if the bile salts or lipase have access to the lipid droplets and promote hydrolysis, the digestion products could slowly diffuse away from the interface—slowing in this way the kinetics of the overall process. Indeed, enhancement of the ChN network structure can occur at the pH and ionic strength conditions of the adopted lipid digestion protocol (pH 7.0 and CaCl2 5 mM); this is manifested by a strengthening of the ChN gelling behavior.28,33 ChNs are positively charged particles at low pH, but with a pKa of approximately 6.5,31 they have a tendency to aggregate under the digestion conditions employed (pH 7.0, salt), due to shielding of the electrostatic interactions resulting from the reduction of the charge density and double layer thickness. This can further promote the isotropic-to-nematic phase transition and thereby the establishment of a gel network structure.28,31
Finally, it is worth pointing out that chitin and chitosan themselves display hypolipidemic and hypocholesterolemic properties. Many in vitro and clinical studies have revealed that both chitin and chitosan inhibit pancreatic lipase46 and thereby reduce the intestinal absorption of dietary fat; i.e. they exert an anti-obesity effect in high-fat diet-treated subjects. Moreover, chitosan and chitin are weak anion exchangers and thus they would be expected to bind bile salts.47,48 In the same context, ChN being positively charged, may repel the Ca2+ ions from the interface, so that they are not able to bind and precipitate long chain FFAs that accumulate at the oil–water interface due to lipolysis of the emulsified triacylglycerols. If the fatty acids are not removed from the lipid droplet surfaces, they may accumulate and limit the ability of lipase to access and hydrolyse the emulsified triacylglycerols. However, despite the abundance of research on chitin and chitosan, the underlying mechanisms of lessening the lipid digestion process are still not completely understood.49
In the case of ChN emulsions with different droplet sizes, no major differences among the various samples were observed and this may be attributed either to the possibility that the larger droplets were less covered by ChN, therefore being more accessible to bile salts, or the chitin nanocrystal particle properties play an important role in lipolysis. Further studies are required, however, to explore the underlying mechanism(s) for this observation.
Overall, the retardation of the in vitro lipolysis in ChN-stabilized emulsions could be attributed to a combination of all the aforementioned factors, as schematically illustrated in Fig. 8.
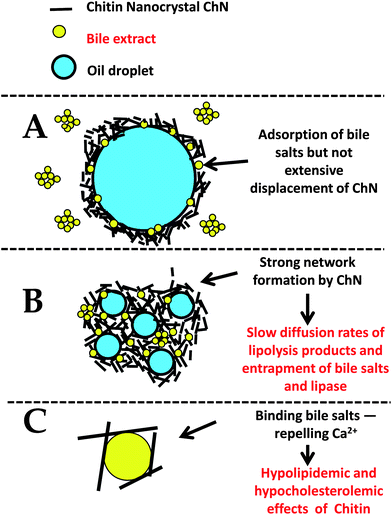 |
| Fig. 8 Schematic diagram for the proposed mechanism(s) of the reduced lipid digestion in o/w emulsions stabilized by ChN particles (not to scale). | |
4 Conclusions
Chitin nanocrystal (ChN) particle-stabilized emulsions were studied for their endurance in lipid digestion conditions and the results were compared to the respective data for WPI- and SCn-stabilized emulsions. The ChN-stabilized emulsions exhibited improved stability against lipolysis, compared to milk protein-stabilized emulsions. Moreover, the ChN containing emulsions were relatively stable towards coalescence, whereas those stabilized by the milk proteins displayed a significant increase in their droplet size, following in vitro lipid digestion. However, no major differences were observed between the nanocrystal particle- and protein-stabilized emulsions in terms of their interfacial properties. The increased stability of the ChN containing emulsions can be attributed to the irreversible adsorption of the ChN particles at the interface, resulting in the formation of a ChN network in the bulk phase that may entrap/protect the lipid droplets and decrease the diffusion rates of the lipolysis products, and to the ability of chitin and consequently of the ChNs to reduce lipase activity. The results of the present study clearly point to the potential of using ChNs for the design of food dispersions with tailored functional properties, aiming at combating chronic diseases such as obesity, diabetes, etc.
Acknowledgements
This research has been co-financed by the European Union (European Social Fund, ESF) and Greek national funds through the Operational Program "Education and Lifelong Learning" of the National Strategic Reference Framework (NSRF)—Research Funding Program: Heracleitus II,“Investing in knowledge society through the European Social Fund”. Part of the experimental work was carried out at Wageningen University (Cost Action 1001).
References
- P. J. Wilde and B. S. Chu, Adv. Colloid Interface Sci., 2011, 165, 14–22 CrossRef CAS.
- P. W. J. Maljaars, H. P. F. Peters, D. J. Mela and A. A. M. Masclee, Physiol. Behav., 2008, 95, 271–281 CrossRef CAS.
- J. S. Patton and M. C. Carey, Science, 1979, 204, 145–148 CAS.
- A. M. N. Renzaho and D. Mellor, Nutrition, 2010, 26, 1–9 CrossRef.
- R. C. Benshitrit, C. S. Levi, S. L. Tal, E. Shimoni and U. Lesmes, Food Funct., 2012, 3, 10–21 CAS.
- D. J. McClements, E. A. Decker, Y. Park and J. Weiss, Crit. Rev. Food Sci. Nutr., 2009, 49, 577–606 CrossRef CAS.
- D. J. McClements, E. A. Decker and Y. Park, Crit. Rev. Food Sci. Nutr., 2009, 49, 48–67 CrossRef.
- M. Golding and T. J. Wooster, Curr. Opin. Colloid Interface Sci., 2010, 15, 90–101 CrossRef CAS.
- M. Armand, P. Borel, P. Ythier, G. Dutot, C. Melin, M. Senft, H. Lafont and D. Lairon, J. Nutr. Biochem., 1992, 3, 333–341 CrossRef CAS.
- M. Armand, B. Pasquier, M. Andre, P. Borel, M. Senft, J. Peyrot, J. Salducci, H. Portugal, V. Jaussan and D. Lairon, Am. J. Clin. Nutr., 1999, 70, 1096–1106 CAS.
- S. Mun, E. A. Decker, Y. Park, J. Weiss and D. J. McClements, Food Biophys., 2006, 1, 21–29 CrossRef.
- S. Mun, E. A. Decker and D. J. McClements, Food Res. Int., 2007, 40, 770–781 CrossRef CAS.
- J. Maldonado-Valderrama, N. C. Woodward, A. P. Gunning, M. J. Ridout, F. A. Husband, A. R. Mackie, V. J. Morris and P. J. Wilde, Langmuir, 2008, 24, 6759–6767 CrossRef CAS.
- J. Maldonado-Valderrama, A. P. Gunning, M. J. Ridout, P. J. Wilde and V. J. Morris, Eur. Phys. J. E, 2009, 30, 165–174 CrossRef CAS.
- J. Maldonado-Valderrama, A. P. Gunning, P. J. Wilde and V. J. Morris, Soft Matter, 2010, 6, 4908–4915 RSC.
- M. S. Katsuda, D. J. McClements, L. H. S. Miglioranza and E. A. Decker, J. Agric. Food Chem., 2008, 56, 5926–5931 CrossRef CAS.
- Y. S. Gu, E. A. Decker and D. J. McClements, Food Hydrocolloids, 2007, 21, 516–526 CrossRef CAS.
- D. Guzey and D. J. McClements, Food Biophys., 2006, 1, 30–40 CrossRef.
- U. Lesmes, S. Sandra, E. A. Decker and D. J. McClements, Food Chem., 2010, 123, 99–106 CrossRef CAS.
- D. J. McClements, J. Food Sci., 2010, 75, R30–R42 CrossRef CAS.
- B. S. Chu, G. T. Rich, M. J. Ridout, R. M. Faulks, M. S. J. Wickham and P. J. Wilde, Langmuir, 2009, 25, 9352–9360 CrossRef CAS.
- S. U. Pickering, J. Chem. Soc. Trans., 1907, 91, 2001–2021 RSC.
- T. S. Horozov and B. P. Binks, Angew. Chem., Int. Ed., 2006, 45, 773–776 CrossRef CAS.
- E. Dickinson, Curr. Opin. Colloid
Interface Sci., 2010, 15, 40–49 CrossRef CAS.
- R. Aveyard, B. P. Binks and J. H. Clint, Adv. Colloid Interface Sci., 2003, 100, 503–546 CrossRef.
- M. V. Tzoumaki, T. Moschakis, V. Kiosseoglou and C. G. Biliaderis, Food Hydrocolloids, 2011, 25, 1521–1529 CrossRef CAS.
- S. Tcholakova, N. D. Denkov and A. Lips, Phys. Chem. Chem. Phys., 2008, 10, 1608–1627 RSC.
- M. V. Tzoumaki, T. Moschakis and C. G. Biliaderis, Biomacromolecules, 2010, 11, 175–181 CrossRef CAS.
- R. H. Marchessault, F. F. Morehead and N. M. Walter, Nature, 1959, 184, 632–633 CrossRef CAS.
- J. F. Revol, H. Bradford, J. Giasson, R. H. Marchessault and D. G. Gray, Int. J. Biol. Macromol., 1992, 14, 170–172 CrossRef CAS.
- J. F. Revol and R. H. Marchessault, Int. J. Biol. Macromol., 1993, 15, 329–335 CrossRef CAS.
- J. Li, J. F. Revol, E. Naranjo and R. H. Marchessault, Int. J. Biol. Macromol., 1996, 18, 177–187 CrossRef CAS.
- M. V. Tzoumaki, T. Moschakis and C. G. Biliaderis, Food Hydrocolloids, 2011, 25, 935–942 CrossRef CAS.
- D. J. McClements and Y. Li, Food Funct., 2010, 1, 32–59 CAS.
- M. Hu, Y. Li, E. A. Decker, H. Xiao and D. J. McClements, Food Biophys., 2011, 6, 37–48 CrossRef.
- C. G. Biliaderis, D. R. Grant and J. R. Vose, Cereal Chem., 1981, 58, 502–507 CAS.
- J. Benjamins, A. Cagna and E. H. LucassenReynders, Colloids Surf., A, 1996, 114, 245–254 CrossRef CAS.
- D. J. McClements, Langmuir, 2005, 21, 9777–9785 CrossRef CAS.
- S. J. Hur, E. A. Decker and D. J. McClements, Food Chem., 2009, 114, 253–262 CrossRef CAS.
- A. Torcello-Gomez, J. Maldonado-Valderrama, A. Martin-Rodriguez and D. J. McClements, Soft Matter, 2011, 7, 6167–6177 RSC.
- P. Reis, K. Holmberg, R. Miller, M. E. Leser, T. Raab and H. J. Watzke, C. R. Chim., 2009, 12, 163–170 CrossRef CAS.
- P. Reis, K. Holmberg, H. Watzke, M. E. Leser and R. Miller, Adv. Colloid Interface Sci., 2009, 147–148, 237–250 CrossRef CAS.
- E. Bauer, S. Jakob and R. Mosenthin, Asian-Australas. J. Anim. Sci., 2005, 18, 282–295 CAS.
- M. M. Nik, A. J. Wright and M. Corredig, Food Funct., 2010, 1, 141–148 Search PubMed.
- A. Sarkar, D. S. Horne and H. Singh, Food Hydrocolloids, 2010, 24, 142–151 CrossRef CAS.
- L. K. Han, Y. Kimura and H. Okuda, Int. J. Obes., 1999, 23, 174–179 CAS.
- C. M. Gallaher, J. Munion, R. Hesslink, J. Wise and D. D. Gallaher, FASEB J., 1999, 13, A883 Search PubMed.
- C. M. Gallaher, J. Munion, R. Hesslink, J. Wise and D. D. Gallaher, J. Nutr., 2000, 130, 2753–2759 CAS.
- M. S. Rodriguez and L. E. Albertengo, Biosci., Biotechnol., Biochem., 2005, 69, 2057–2062 CrossRef CAS.
|
This journal is © The Royal Society of Chemistry 2013 |
Click here to see how this site uses Cookies. View our privacy policy here.