DOI:
10.1039/C3BM60264H
(Paper)
Biomater. Sci., 2014,
2, 710-722
Integrative and comparative analysis of coiled-coil based marine snail egg cases – a model for biomimetic elastomers†
Received
1st November 2013
, Accepted 9th December 2013
First published on 15th January 2014
Abstract
Integrative and comparative analyses of biomaterials systems offer the potential to reveal conserved elements that are essential for mechanical function. The approach also affords the opportunity to identify variation in designs at multiple length scales, enabling the delineation of a range of parameters for creating precisely tuned biomimetic materials. We investigated the molecular design and structural hierarchy of elastomeric egg capsules from the marine snail Pugilina cochlidium (family Melongenidae) and compared these data with all available published studies in order to infer the structure–property relationships of the egg case from the molecular to the macroscopic scale. While mechanical similarities had previously been observed for two other marine melongenid snails, Busycotypus canaliculatus and Busycon carica, B. canaliculatus was the only species for which detailed molecular and nanostructural data were available. Egg capsules from P. cochlidium were found to exhibit mechanical properties and shock absorbing potential that was similar to B. canaliculatus. The two species also displayed similarity in hierarchical fibril bundling and a sub-micron staggering of 100–105 nm within filaments, as shown by atomic force microscopy and small angle X-ray diffraction. In situ Raman micro spectroscopy indicated that P. cochlidium egg cases undergo a stress-induced coiled-coil to extended β-strand structural transformation that is very similar to that of B. canaliculatus. These observations supported the view that these structural and hierarchical elements are essential for egg case function. Comparative analysis of the primary amino acid sequences and structural predictions for all known egg case proteins suggested that while the proteins all contain sequences prone to adopt α-helical structures, the predicted location of coiled-coil domains and stutter perturbations varied within and between species. Despite these differences, mixtures of denatured native egg case proteins readily re-folded in citrate–phosphate assembly buffer into α-helix rich, coiled-coil based oligomers, as determined by attenuated total reflection Fourier transform infrared spectroscopy, circular dichroism and MALDI-TOF. It is concluded that both conserved and divergent designs in marine snail egg cases offer inspiration for the engineering of biomimetic elastomeric materials with a unique capability for mechanical energy absorption.
1. Introduction
The coiled-coil is a widespread structural motif found in all classes of proteins, from globular and membrane proteins to fibrous proteins.1 Coiled-coils play key roles in directing protein–protein interactions, in muscular structure and function, and in providing mechanical integrity to the cytoskeleton, among many other functions.2 Because of this versatility and the relative simplicity of primary sequence motifs responsible for coiled-coil assembly, they have generated significant interest for use as building blocks in de novo protein engineering studies, with applications in drug-delivery,3–5 proto-cell engineering,6 biosensing,7–10 and as materials that direct self-assembly of metallic nanoparticles.11–13
Among their diverse functions, coiled-coils play essential roles as load-bearing elements in various fibrous proteins and protective membranes. This includes a mechanical role in myosin,14–16 intermediate filaments (IFs) of the cell cytoskeleton,17,18 and fibrin from blood clots.19–21 In such instances, an emerging theme is the ability of coiled-coils to exhibit a high degree of elasticity through the unraveling of the superhelices and the formation of extended chain β-conformations under external stresses.22 Hence, there is strong interest in understanding the mechanical role of coiled-coil based materials at multiple levels of structural hierarchy. Recently, it has been established that egg cases secreted by marine snails are predominantly assembled from coiled-coil proteins.23,24 The egg cases are multifunctional, providing mechanical protection to developing embryos while allowing gas and nutrient exchange and a timed breakdown that enables the release of larvae. These materials represent an excellent model system to study coiled-coil mechanics and assembly across multiple length scales for several reasons. First, the secreted egg cases are relatively large. In Busycotypus canaliculatus (Fig. 1), which has attracted the most attention from the biomaterials community, the females lay a string of up to 100 egg capsules, each about 3–4 cm in diameter, enabling extensive macroscopic mechanical studies to be carried out23,25,26 in conjunction with in situ X-ray diffraction and other solid-state spectroscopy techniques.23,24 Second, large amounts of the soluble precursor proteins can be extracted and purified from the nidamental glands of these animals enabling investigations into the biochemistry of the egg case proteins that self-assemble and sclerotize into the final protective protein-based membrane. Hence, the system is in many respects ideal for investigating and integrating the relationships between coiled-coil protein sequence design and biochemistry with biophysical and biomechanical properties.
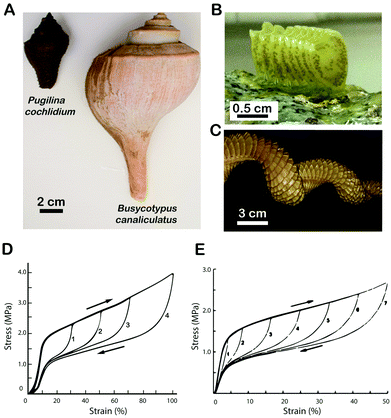 |
| Fig. 1 (A) Comparison of Pugilina cochlidium and Busycotypus canaliculatus gross morphology. (B) String of egg capsules from P. cochlidium. (C) String of egg capsules from B. canaliculatus. (D) Load cycle stress–strain curves of the P. cochlidium egg case. (E) Load cycle stress–strain curves of the B. canaliculatus egg case25 – reprinted (adapted) with permission from H. S. Rapoport and R. E. Shadwick, Biomacromolecules, 2002, 3, 42–50. Copyright 2002 American Chemical Society. | |
The mechanical properties of P. cochlidium and B. canaliculatus egg case membranes have recently been linked to the molecular design of their protein building blocks and nano-structural features.27,28 The membrane is assembled from bundles of coiled-coil α-helical proteins, which upon applied load first unravel and then transform into stiffer extended β-conformations. The process is fully elastic up to ∼150% macroscopic strain and, upon release of the load, the coiled-coils reform. Remarkably, the process is almost instantaneous, and is accompanied by mechanical hysteresis during the full loading/unloading cycle, imparting a shock-absorbing capability to the protein-based membrane, a desired property in many tissue engineering applications. To further expand our understanding of the egg case system, and by extension coiled-coil based systems in general, we adopted a comparative approach which has the potential (i) to allow us to clearly define the conserved structural and functional elements at multiple levels of biological organization, (ii) to reveal novel biological design strategies, and (iii) to provide insights into the evolutionary bases for adaptation. Information gleaned from integrative and comparative biomaterials research can also be rapidly leveraged into protein engineering design principles for creating biomimetic materials in a bottom-up fashion.
Here we present an investigation of the mechanical, structural, biochemical and molecular designs of the egg case from the marine snail Pugilina cochlidium. Where possible we compare our data sets with data available in the literature, which for the most part is limited to the marine snail B. canaliculatus. While molecular systematic studies indicate that B. canaliculatus and P. cochlidium are closely related,29P. cochlidium inhabits the murky waters of marine effluents of the Indo-Pacific region, whereas B. canaliculatus is found in the colder Northern Atlantic wave swept coastlines, suggesting that their egg cases are subject to distinct selective pressures. We aimed to identify both common and divergent design principles at multiple scales of hierarchy, with the goal of establishing their key molecular and structural characteristics for future biomimetic efforts to engineer these materials.
2. Materials and methods
2.1 Animal collection, egg case collection and nidamental gland dissection
Live P. cochlidium female snails (Fig. 1A) were collected at low tide from a large tidal monsoon drain at East Coast Park Singapore. Animals were maintained in aquaria at 27 °C in artificial sea water and fed with barnacles.30 The snails deposited fresh egg case strings on an intermittent basis and these materials were immediately harvested from the substrate and frozen at −80 °C. Nidamental (capsule) glands were obtained by euthanizing individual snails by submersion in a 7.5% solution of MgCl2 prior to dissection. The shell was cracked with vise grips at the spire and sterile scalpel blades and forceps were used to isolate and dissect the white oblong nidamental gland. Glands were stored immediately at −80 °C. B. canaliculatus egg cases and nidamental gland materials were purchased from the Marine Biological Laboratories (Woods Hole, Massachusetts, USA).
2.2 Mechanical testing of the P. cochlidium egg case
P. cochlidium egg case walls were cut to dimensions of 3 mm × 8 mm using a scalpel. Each end was glued between 2 pieces of cardboard using epoxy, resulting in a test region measuring 3 mm × 5 mm. The thickness of the sample was measured using a micrometer. Mechanical testing was done at 25 °C on hydrated samples at a 2.5 mm min−1 strain rate using a 50 N load cell on a universal tensile testing device (Model 5848 MicroTester, Instron Corporation, MA, USA). Regular cyclic loading was also performed. A total of 10 test samples were used.
2.3 Characterization of the fibrillar morphology of the P. cochlidium egg case
Scanning electron microscope (SEM) images of P. cochlidium egg cases were obtained using JEOL 5410LV and JEOL 6340F SEMs. Dry egg capsules were attached to a piece of carbon tape and mounted on an SEM stub. The samples were gold or platinum sputter coated prior to analysis. Atomic force microscopy images were generated by mounting egg capsule slices on microscope slides using epoxy. Images were obtained using the tapping mode with a MFP-3D-BIO atomic force microscope (AFM) (Asylum Research, Santa Barbara, California, USA). Imaging was performed on hydrated samples in Milli-Q water by driving a BL-RC150VB cantilever with a scan rate of 0.5 Hz.
2.4 Small angle X-ray scattering (SAXS) characterization of the P. cochlidium egg case
SAXS measurements were obtained at beamline I22 (Non-Crystalline Diffraction), Diamond Light Source (DLS), Harwell Science and Innovation Campus, Harwell, UK. A wavelength of λ = 1.21 Å and a beam cross-section of 30 μm × 20 μm were used. SAXS patterns were collected using a 2D Pilatus2M area detector. The sample to detector distance was 780 mm ± 1 mm as measured with a calibration standard of a dry rat tail tendon (with a collagen D stagger of 67 nm). Each SAXS frame had a resolution of 2048 × 2048 pixels and a pixel size of 80 μm × 80 μm. Egg capsules were strained in situ using a custom-built micromechanical tester31 equipped with a 22 N load cell (model 31 tension/compression load cell; SLC31/00005; RDP Electronics, West Midlands, UK) and strain-detection by video extensometry. Samples were tested in a fluid chamber immersed in phosphate buffered saline (PBS) to simulate the physiological hydration state. Measurements were taken at 0%, 12%, 24%, 40% and 80% material strain. Two-dimensional X-ray scattering patterns were analyzed using the FIT2D software package.32 The SAXS scattering intensity was integrated using the CAKE command in FIT2D. The angular centre of the CAKE sector was taken as the position of maximum SAXS diffraction peak intensity in the unstrained state, and the CAKE width was taken to be ∼25° to include the majority of the SAXS peak intensity. Inter-sample variation existed, but the majority of angular centers of the peak position were within ±30° of the (vertical) loading direction. The results were plotted as intensity against the scattering vector.
2.5 Analysis of secondary structural transition by in situ Raman spectroscopy
Raman spectra of the P. cochlidium egg case were obtained during mechanical deformation as previously described for B. canaliculatus egg cases.24 Briefly, a diode-pumped laser (λ = 785 nm; Toptica Photonics AG, Graefelfing, Germany) was focused on the sample through a water immersed 60× microscope objective (Nikon, N.A. = 1.0) using a confocal Raman microscope (CRM200, WITec, Ulm, Germany) equipped with a piezo-scanner (P-500, Physik Instrumente, Karlsruhe, Germany). The incident light was passed through a half-wave plate to rotate the polarization, and a polarizer (the analyzer) was placed in front of the confocal microscope pinhole. Spectra were acquired using a CCD (PI-MAX, Princeton Instruments Inc., Trenton, NJ, USA) behind a grating (300 g mm−1) spectrograph (Acton, Princeton Instruments Inc., Trenton, NJ, USA) with a spectral resolution of approximately 6 cm−1. A strip of the P. cochlidium egg case wall was dissected from the intact egg case, fixed in a plastic frame on both ends, clamped in the grips of the mechanical tester and then stretched at a constant rate of 20 mm s−1 to 60% strain in water. The material was allowed to relax for approximately 5 min before measuring. At both strain values (0% and 60%), a depth scan was performed from the surface to a depth of 40 μm, after which signal intensity degraded. Afterwards, four spectra were acquired at the depth of highest intensity of the amide I band with the polarizer and analyzer parallel, perpendicular and cross-polarized with respect to the direction of loading. Each spectrum consists of 3 accumulations with a 2 s integration time, and this was repeated 10 times and averaged. From these data, isotropic spectra were calculated as described previously.33 Spectra were background subtracted, smoothed with a 7 point Savitzky–Golay filter, and normalized to the intensity of the CH-bending peak (1425–1490 cm−1).
2.6 Amino acid composition analysis of the P. cochlidium egg case and egg case proteins (ECPs)
Whole P. cochlidium egg cases and nidamental gland extracts were hydrolyzed in vacuo in 6 M HCl/5% phenol overnight at 110 °C, washed twice with methanol and twice with water on a vacuum evaporator (SpeedVac) and analyzed on a post-column derivatization, ninhydrin-based S433 Sykam amino acid analyzer (Sykam GmbH, Eresing, Germany).
2.7 Analysis of protein composition of the P. cochlidium egg case
Female snails were euthanized and nidamental glands dissected as described above. The outer nidamental gland tissue was removed with a scalpel and egg case proteins retrieved with a small spatula. The proteins were dissolved with frequent vortexing and sonication at ∼2 mg ml−1 in 6 M urea with 5% acetic acid and 10 mM TCEP. This mixture of native ECPs, which we call the crude extract, was then subjected to SDS–PAGE with a 10% polyacrylamide gel. Crude extracts were also centrifuged at 14
000g, filtered with a 0.2 μm cutoff filter (Millipore) and analyzed by Matrix-Assisted Laser Desorption Ionization Time-of-Flight (MALDI-TOF) mass spectrometry. 2 μl of the sample was spotted onto the MALDI sample plate and allowed to air dry before the addition of 2 μl of 10 mg ml−1 Sinapinic acid matrix in 50% acetonitrile with 0.1% TFA, which was spotted on top of the ECP sample and also left to dry. MALDI data were obtained using a Kratos Axima Performance (Kratos-Shimadzu Biotech) instrument equipped with an N2 laser (337 nm, 4 ns pulse). An accelerating voltage of 20 kV was used, and spectra were recorded in linear mode by averaging 100 laser shots at a power of 100 system units. Edman sequencing was carried out by transferring the ECPs from SDS–PAGE gels onto Bio-Rad polyvinylidene difluoride (PVDF) membranes, excising the bands and submitting them to the Protein Facility of Iowa State University (Ames, Iowa, USA).
2.8 Primary amino acid sequence analysis
The primary amino acid sequences of all known ECPs were aligned with ClustalW34 and visualized using Jalview.35 Secondary structure predictions were generated with JPRED-3.36 Predictions of propensity to adopt coiled-coil structure were generated using SCORER 2.0 using a 50% threshold setting.37
2.9 Self-assembly and structural characterization of P. cochlidium and B. canaliculatus ECPs
The major protein constituents of the P. cochlidium and B. canaliculatus nidamental gland secretions were purified using an Agilent 1260 Infinity HPLC with a reverse phase Zorbax 300-SB-C8 column. Two milliliters of the ∼2 mg ml−1 nidamental gland crude extract (solubilized in 6 M urea, 5% acetic acid and 10 mM TCEP) were injected for each run. The mobile phase for gradient elution was acetonitrile (ACN) with 0.1% trifluoroacetic acid (TFA) and 10% isopropanol (IPA). The gradient was run from 5% to 100% ACN over 60 minutes. 1 ml fractions were collected over this time course and freeze dried. The similarity in primary amino acid sequence and biophysical properties of the ECPs resulted in an extensive set of fractions that contained all of the major proteins identified by SDS–PAGE to the exclusion of other trace proteins. These mixtures of proteins were used in subsequent self-assembly and solution state conformational characterizations. Individual self-assembly experiments were conducted by dissolving 1 mg of the denatured ECP mixtures in a 1 ml solution containing 5 mM citrate and 10 mM phosphate at pH 5 (ECPs have limited solubility at more neutral pH). The samples were then centrifuged at 14
000g for 10 minutes and the supernatant used for subsequent structural measurements.
The structure of egg cases and ECPs in solution was evaluated by Attenuated Total Reflection Fourier Transform Infrared Spectroscopy (ATR-FTIR). Infrared absorption measurements were obtained with a VERTEX 70 or 70v FTIR spectrometer (Bruker, Karlsruhe, Baden-Wurttemberg, Germany), respectively equipped with PIKE MIRacle and Harrick Horizon attenuated total reflection (ATR) measuring units, each containing a ZnSe ATR crystal (angle of incidence θ = 45°). The sample chamber was continuously purged with dry N2 gas, and the total reflected IR beam intensity was measured using a liquid nitrogen-cooled photovoltaic mercury cadmium telluride (MCT) detector. Spectra were recorded with a spectral resolution of 4 cm−1 in double-sided acquisition mode, and the mirror velocity was set to 80 kHz. At least 100 scans were taken for each spectrum, which was calculated using a 3-term Blackman–Harris apodization function. Spectra of egg cases and proteins were obtained by subtracting IR absorption due to the citrate-phosphate buffer. Secondary structure analysis was conducted with the OPUS 6.5 software (Bruker, Karlsruhe, Baden-Wurttemberg, Germany).
The conformation of self-assembled ECPs was also investigated by circular dichroism (CD) where spectra were obtained at 1 nm resolution using a Chirascan CD spectrometer (Applied Photophysics, England, United Kingdom) with a quartz cell of path length 0.1 mm. For each sample, 10 scans were acquired at room temperature and averaged. In all cases the buffer spectra were subtracted from the resulting spectra. Secondary structure analyses were done using the K2D3 method,38 with spectra scaled linearly to obtain the best fits. MALDI experiments on self-assembled proteins were conducted as described above.
3. Results and discussion
3.1 Comparative biomechanics of egg case proteins
The mechanical properties of P. cochlidium egg cases were evaluated by tensile testing and load cycling according to previously published methods used for B. canaliculatus egg case characterization.23,25,26 Representative stress–strain curves are shown in Fig. 1D. In general both tensile tests to failure (not shown) and load cycled samples exhibited very similar profiles to those documented for B. canaliculatus (Fig. 1E).23,25,26 The membranes exhibit reversible extension when extended as far as 150–180% and four mechanically distinct regions are observed during uniaxial loading/unloading cycles. First, an initial nearly-linear elastic domain with Young's modulus in the range 50–70 MPa is observed, which is followed by yielding and plateau regions during which large extension occurs at very small force increments. A strain hardening regime is then observed where the stress rapidly increases with strain. Finally, upon unloading to zero stress, the material recovers its initial length where the unloading plateau region occurs at lower stress values than during loading, resulting in significant hysteresis and energy absorption. Table 1 compares the mechanical properties of egg cases from both species. For most of the engineering properties evaluated, no significant differences were observed, with the exception of resilience, where our measured values were slightly greater than those for B. canaliculatus.
Table 1 Comparative summary of the mechanical properties of P. cochlidium and B. canaliculatus28 egg cases
|
B. canaliculatus egg case |
P. cochlidium egg case |
Initial stiffness (MPa) |
60 |
47.1 ± 9.2 |
Yield stress (MPa) |
2.5 |
1.9 ± 0.2 |
Yield strain (%) |
5 |
4.1 ± 1.3 |
Breaking stress (MPa) |
10 |
8.1 ± 0.2 |
Breaking strain (%) |
169 |
170.7 ± 14.7 |
Strain energy at failure (MJ m−3) |
8.5 |
8.3 ± 0.7 |
Resilience (%) |
49 |
60.1 ± 2.9 |
3.2 Comparative morphology of the egg case
We used SEM and AFM to investigate the large- and small-scale fibrillar organization of the P. cochlidium egg case. Fig. 2A shows a low magnification SEM image of this egg case. At this scale, the material appeared to be assembled from distinct layers containing relatively large fibrils >2 μm, with a preferred fibril orientation within the layers. In comparison to the B. canaliculatus egg capsule whose preferred fibril orientation between adjacent layers was found to have an orthotropic organization, the fibril orientation in P. cochlidium egg cases appears more isotropic, namely a slight misorientation was noticed from layer to layer. Under high-resolution SEM, the filaments exhibit a crimped morphology on their surface (Fig. 2B). Examination of the crimped structures by AFM revealed a distinct banding pattern (Fig. 2C and D) with a regular inter-banding spacing of ∼100–105 nm. Notably, the B. canaliculatus egg case exhibits a very similar design in fibril dimensions and banding pattern.22,23 The banding pattern was then examined in greater detail. A line scan of a high-resolution image of the banded region (Fig. 2D) revealed a distinct repetitive feature that had not previously been observed (Fig. 2E). Here, an additional crimp which appears as a peak doublet (labeled D) was found to often alternate with a single peak (singlet labeled S). The precise morphology and function of this feature warrant further investigation. As a first step in this process we generated a putative 3D model using AutoCad™ that incorporated this feature (Fig. 2F). The model suggests a possible structure for the intercalation of singlets and doublets that could facilitate the hierarchical packing of ECP fibrils. Overall, a key feature of these observations is the regular interbanding spacing of 103 nm observed for all fibrils at high magnification.
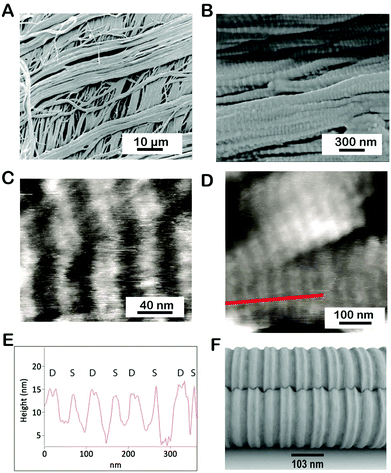 |
| Fig. 2 Fibrillar morphology of the P. cochlidium egg case. (A) Low magnification SEM image of the egg case showing orthogonally arranged fibrils. (B) Higher magnification image of fibril organization showing crimp. (C) AFM image of the banded stagger region of the egg case. (D) High resolution AFM image of the egg case. (E) Height profile of region delineated by the red line in (D). The data suggest the presence of crimp singlets (labeled S) and doublets (labeled D). (F) Model of crimp intercalation. | |
3.3 Structural analysis of the egg case by SAXS
We conducted small angle X-ray diffraction (SAXS) on a single P. cochlidium egg case at 0%, 12%, 24%, 40% and 80% strain which resulted in two-dimensional scattering patterns that were overall similar to those previously documented for B. canaliculatus (Fig. 3). In the unstrained state, a set of nearly circular reflections was observed, which were slightly more intense at 90° from each other. Upon straining, the intensity of reflections parallel to the loading direction decreased from 0% strain to 40% strain. At 80% strain the reflections at all angles completely vanished, denoting loss of long-range order. Upon unloading to 0% strain, full recovery of the SAXS pattern was observed (not shown). Analysis of the scattering patterns by integration along the loading direction (Fig. 3, lower right panel) revealed several regularly spaced peaks, with an average ΔQ value of 0.060 nm−1 and visible reflections up to the 7th order. This set of reflections corresponds to a D-spacing of ∼105 nm, which essentially matches the interbanding spacing detected by AFM imaging. This regular pattern (which is observed in both species) is reminiscent of the classical 67 nm staggering pattern observed in collagen fibrils, and suggests a highly conserved sub-micron fibrillar structure of egg cases. In contrast to B. canaliculatus,24 a new set of reflections was not observed at high strains. There are two possibilities that can explain this lack of reflections. First, this could be related to the thinner section of P. cochlidium samples in comparison to B. canaliculatus, such that when they are stretched the electronic contrast decreases. It is also possible that stretching induces temporary disorder, with sharp staggered interfaces becoming fuzzier upon straining.
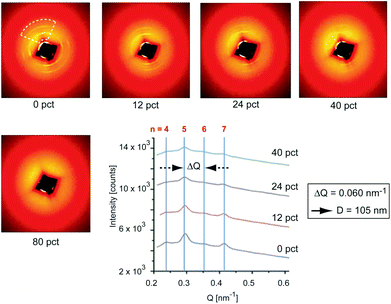 |
| Fig. 3 SAXS patterns of the P. cochlidium egg case. 2D SAXS patterns of the egg case at progressively increasing material strain levels. The dashed line represents the angular sector over which intensity is azimuthally averaged to give line scans shown in the lower right panel. The azimuthally averaged line profile of SAXS intensity is vertically translated between measurements at different material strain levels for visual clarity. Peaks corresponding to the D spacing of ∼105 nm are visible, with the 5th and 7th orders being especially clear. Reductions of intensity with increased material strain indicate loss of axial paracrystalline order. | |
3.4 Observation of the α-helix to β* transition by Raman spectroscopy
Our previous wide angle X-ray diffraction experiments clearly indicated the presence of coil–coil structure in the unstrained P. cochlidium egg case.27 However, while the α-helix to β* conformational transition has been documented in B. canaliculatus egg cases,24 it had not been observed for P. cochlidium. We therefore obtained isotropic Raman spectra on this material at 0% and 60% strain (Fig. 4). The results indicate clear changes in peak position and intensity for the P. cochlidium egg case that are consistent with the transition of a coiled-coil α-helical conformation to an extended β* conformation, as previously reported for B. canaliculatus.24 Specifically, we observe a shift of the amide I band to higher wavenumbers, the appearance of a peak at ∼1400 cm−1, and most strikingly, the appearance of an amide III band in the region of 1190–1260 cm−1. These conformational changes were found to be completely reversible immediately upon relaxation back to 0% strain.
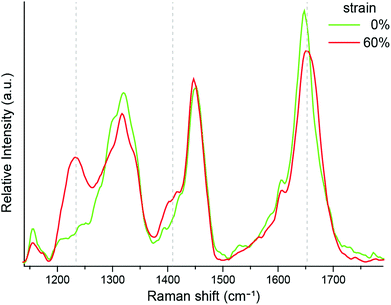 |
| Fig. 4 Isotropic Raman spectra of the P. cochlidium egg case during in situ mechanical testing. Stretching the P. cochlidium egg case material resulted in changes in the Raman spectra indicated by dashed lines that are all consistent with the transformation of an α-helical conformation to an extended β* conformation. | |
3.5 Egg case protein composition
By screening a P. cochlidium RNA-seq transcriptome library with the published B. canaliculatus egg case protein sequences (BcECPs), we previously identified two P. cochlidium protein sequences (PcECPs) with predicted molecular weights of 46.1 and 53.4 kDa.27Fig. 5A shows the SDS–PAGE results for the nidamental gland crude extracts retrieved from both species. Interestingly, the gel revealed at least 5 major bands for P. cochlidium that ranged in molecular weight between ∼40 kDa and 55 kDa. The results also suggested that the protein composition is not identical between species. To further investigate the PcECPs we conducted Matrix Assisted Laser Desorption Ionization Time-of Light mass spectrometry (MALDI-TOF) on these samples and observed 5 distinct peaks at 42.0, 47.5, 50.1, 51.5 and 54.2 kDa (Fig. 5B). The molecular weights of B. canaliculatus ECPs were 48.0 (BcECP-1a and b), 51.4 (BcECP-2) and 52.5 (BcECP-3) kDa (Fig. 5C). Based on this information, we screened our P. cochlidium RNA-seq database extensively using homology based search parameters in an attempt to identify additional PcECPs that would correspond to the additional bands observed by SDS–PAGE. However, this comprehensive screening did not yield any additional proteins. We then conducted Edman sequencing on each of the 5 P. cochlidium protein bands from our SDS–PAGE gels and Table S1† summarizes these results. The Edman sequences from bands 1 and 2 were a perfect match to PcECP-1 and band 3 yielded a reasonable match (7/10 residues) to PcECP-1. On the other hand, band 4 provided a close match (8/10 residues) to PcECP-2 and band 5 provided a perfect match to PcECP-2. There are only a few possible explanations for these results. First, we consider that of the four proteins identified for B. canaliculatus, BcECP-1a and 1b were very similar in primary amino acid sequence whereas BcECP-2 and -3 also differ only in 4 additional residues at the C-terminal domain of BcECP-3. Therefore, it is likely that the additional bands we observed on the SDS–PAGE gel for P. cochlidium represent closely related isoforms and/or splicing isoforms of the previously identified PcECPs that are not readily distinguished by the RNA-seq assembly. Post-translational modification must also be considered as a possible reason for the observed variation in molecular weights, although there is to date no evidence for post-translation modification within the nidamental gland.
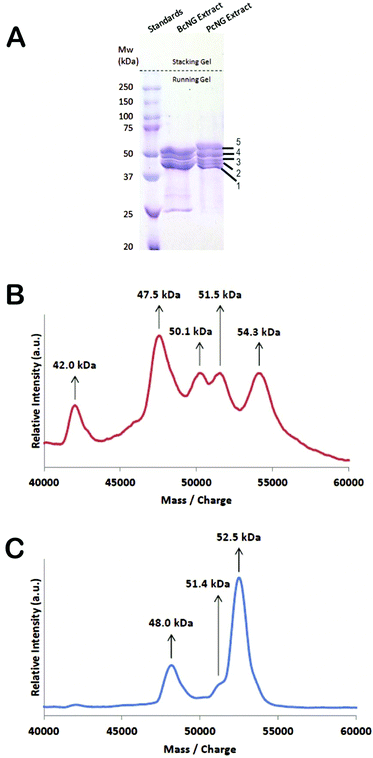 |
| Fig. 5 Protein composition of the nidamental gland. (A) SDS–PAGE of P. cochlidium and B. canaliculatus nidamental gland extracts. (B) MALDI-TOF spectra of the P. cochlidium nidamental gland extract. (C) MALDI-TOF spectra of the B. canaliculatus nidamental gland extract. | |
3.6 Comparative analysis of the primary sequences of egg case protein
Table S2† provides a comparative view of the measured amino acid compositions of egg cases, nidamental gland extracts and predicted amino acid compositions of known proteins from P. cochlidium and B. canaliculatus. In general the proteins are rich in Glutamate/Glutamine, Aspartate/Asparagine, Glycine, Alanine, Leucine and Lysine. In addition, Lysine residues, present at ∼11–9%, may play an important role in sclerotization of the protein during its final processing stages.27 In general all ECPs for which primary sequence is available have predicted molecular weights ranging from 42 kDa to 55 kDa and predicted isoelectric points of 7.5 to 9.5. We used ClustalW based homology sequence alignments to compare and contrast the ECPs (Fig. 6). The data suggest that BcECP-1a and b share close identity with one another (95%) and moderate identity with PcECP-1 (61%), while BcECP-2 and -3 were found to maintain greater identity with one another (>99%) and with PcECP-2 (60%). Notably, BcECP-1b and PcECP-2 share only 18% identity. In general the sequence alignments indicate that many regions of the ECPs are highly conserved, a likely reflection of the requirement of coiled-coil heptad repeat sequences and correct positioning of hydrophobic residues in the a and d positions of the abcdefg heptad repeats. In addition a rigorous conservation of Lysine positions is often apparent (K residues highlighted in red). All 6 proteins contain only two Cysteine residues each, whose positions are also highly conserved. The precise role of these few cysteines in the assembly and mechanics of ECPs remains to be determined but warrants investigation. Fig. S1 and S2† provide a summary of the presence and location of hydrophobic and charged residues respectively in the aligned ECP sequences. These data provide an additional view of the high degree of positional conservation of both hydrophobic and charged residues in the ECPs, which are likely key requirements for effective coiled-coil assembly.
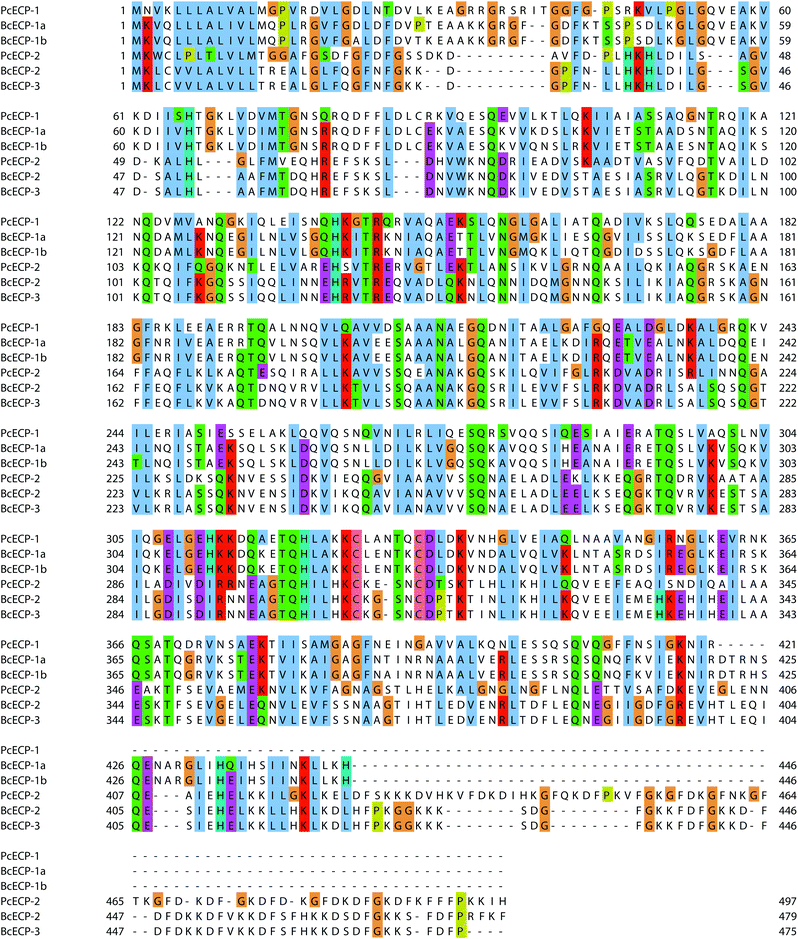 |
| Fig. 6 ClustalW sequence alignments of all known ECPs. | |
Previous studies have shown that all ECP sequences contain extensive heptad repeats, and SCORER 2.0 has been used to predict the location of coiled-coils within the ECPs from both species.27,28 This predictive tool also suggested the presence of trimeric coiled-coils for both the BcECPs and PcECPs. Fig. 7 shows a comparison of the SCORER 2.0 predicted domain architectures of all known ECPs and includes for comparison a generalized depiction of the established domain architecture of intermediate filament (IF) proteins.39 While the primary amino acid sequences of IF-proteins can vary widely, they generally contain a central α-helical rod domain that is flanked by non-helical head and tail domains that vary in length between IFs and the related Lamin proteins.39,40 The central rod contains several distinct coiled coil forming regions, C1A, C1B and C2. C1A and C1B are interrupted by a linker (L1) and C1 and C2 are separated by another linker region, L12. The linkers are believed to result in local non-coiled coil regions within the rod that may result in increased flexibility gained by the presence of parallel helices. The C2 domains of IF proteins also contain a conserved stutter where 3 consecutive residues are absent from the heptad repeat (thin pink rectangle). The stutter has been suggested to enable the α-to-β transformation in IFs by creating a local disruption in the helix and coil structures that facilitate the unwinding of these structures with imposed stress.41 In Fig. 7B we provide the SCORER 2.0 predicted domain architectures of the known ECPs. Given the potential limitations of structural simulations, we provide only generalized observations of the predicted domain architectures. A complete understanding of the relationship between sequence design, assembly and structure will evidently require comprehensive studies similar to those that have shaped our view of IFs over the past few decades.18,40 The figure suggests several interesting aspects of the ECPs. First, the predicted domain architectures of the ECPs appear quite different from known IF proteins. For example, it is not possible to clearly assign head or tail regions to the ECPs; however, it is notable that all of the known ECPs contain N-terminal regions that do not predict strongly for coiled-coil structures. In the case of the C-terminal regions, PcECP-1 and -2 and BcECP-2 and -3 also do not predict for coiled-coil structure, while BcECP-1a and b do. The number and relative positions of predicted coiled-coil forming domains are also of interest. Here, contrary to IF proteins where domain architecture is conserved across diverse Phyla and between distinct classes of IFs, the domain architectures of the ECPs are predicted to vary within and between species. For example the predicted coiled-coil domains of the PcECPs appear to occupy a more central region compared to the BcECPs. In addition PcECP-1 and BcECP-1a and -b are predicted to contain 2 coiled-coil domains, while PcECP-2 predicts for 4 and BcECP-2 and -3 predict for 3 coiled coil domains. Fig. 7B also suggested the presence of “linker” regions between the coiled-coils; however, homology blast searches did not provide any matches to known linker sequences. The position of the stutters in the ECPs is also predicted to vary within and between species suggesting that local unwinding and strain induced α-to-β transformations may take place in a range of different regions in self-assembled ECP-based oligomers. Given the key roles for primary sequence and domain architecture in the self-assembly and mechanical properties of IFs, it is clear that the ECPs warrant detailed future experimentation to clearly identify key local primary sequences and the precise domain architectures in each of these proteins. Nevertheless, the current observations suggest some intriguing possibilities for biomimetic engineering. If a range of primary sequence designs and domain architectures are in fact used by marine snails to generate ECP-based filaments, then it is possible that protein engineers may be afforded a degree of flexibility in the design and assembly of ECP-based elastomers.
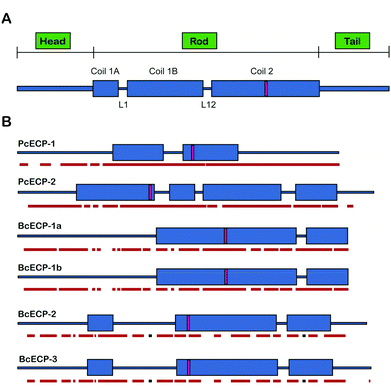 |
| Fig. 7 Predicted domain architecture of ECPs. (A) Domain architecture of the intermediate filament protein.39 (B) Scorer 2.0 predicted domain architecture of known ECPs. Predicted stutter locations are indicated with a pink rectangle. JPRED-3 predicted helical regions are underlined in red and β-sheet predicted regions in black. | |
3.7 Self-assembly of ECPs
The engineering of ECP-based materials with mechanical properties that mimic the native egg case will ultimately require control over the folding and oligomeric assembly of native or recombinantly engineered ECPs. Presently our understanding of the molecular mechanisms that underlie ECP self-assembly is extremely limited. Fortunately, the extensive body of work pertaining to the self-assembly of IFs and other α-helical proteins provides an important backdrop for future investigations. As a step in this direction we re-suspended the denatured and lyophilized HPLC fractions containing mixtures of the most abundant PcECPs and BcECPs in an “assembly” buffer (5 mM citrate/10 mM phosphate buffer, pH 5) at ∼1 mg ml−1. Fig. 8A shows the ATR-FTIR spectra for these samples measured in solution and includes ATR-FTIR spectra of native P. cochlidium and B. canaliculatus egg cases in the same assembly buffer at pH 5 for comparison. All samples yielded clear amide I and II bands and curve fitting indicated that the majority of the amide I band was represented by a ∼1650 cm−1 peak, suggesting the presence of about 55–60% α-helix in both the native egg case and ECPs (Fig. 8B–F). Each of the four different samples was also found to contain ∼20–30% β-sheet structure and ∼10% random-coil or β-turn. The data suggested that denatured ECPs readily re-folded into α-helix dominated structures, potentially a key step in our long-term goal of gaining control over the assembly pathway of native and recombinant ECPs.
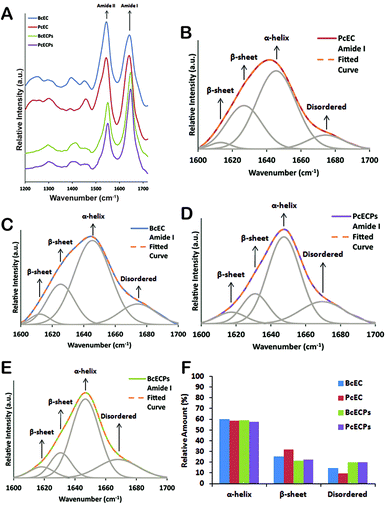 |
| Fig. 8 Structural characterization of the egg case and ECPs by ATR-FTIR. (A) ATR-FTIR spectra of P. cochlidium (Pc) and B. canaliculatus (Bc) egg cases and ECPs in assembly buffer at pH 5. (B) Fitted amide I peak of a P. cochlidium egg case spectrum. (C) Fitted amide I peak of a B. canaliculatus egg case spectrum. (D) Fitted amide I peak of PcECP mixture. (E) Fitted amide I peak of BcECP mixture. (F) Relative proportion of α-helix, β-sheet and random coil structures estimated from curve fittings shown in (B) to (E). | |
We then subjected the same ECP samples to circular dichroism (CD) (Fig. 9). The CD spectra suggested that both PcECP and BcECP samples were dominated by α-helical structure with minima at 208 and 222 nm.42 The 222
:
208 nm ratios for PcECP and BcECP samples were 1.05 and 1.1, respectively, suggesting the presence of a coiled-coil structure under these conditions.43,44 Taken together, the FTIR and CD data support the view that denatured ECPs readily self-assembled into α-helical coiled-coil conformations upon addition of 5 mM citrate/10 mM phosphate buffer at pH 5. To further investigate the oligomeric state of these self-assembled ECPs, we conducted MALDI-TOF. ECPs exposed to assembly buffer yielded clear peaks that correspond to dimers and tetramers (Fig. 10A). Based on the estimated molecular weights of the observed dimers and tetramers, the data suggest the presence of heteropolymers of PcECP-1 and -2 and BcECP-1a/b with BcECP-2/3. We interpret these data with caution, because non-specific protein–protein interactions may occur during MALDI-TOF experiments, especially under high vacuum conditions which may influence the apparent assembly states. Nevertheless only monomeric ECPs were observed in the denatured starting ECP material (Fig. 5B and C). The data therefore strongly suggest that it is possible to efficiently fold denatured ECPs into α-helical structures and assemble them into oligomeric coiled-coil constructs.
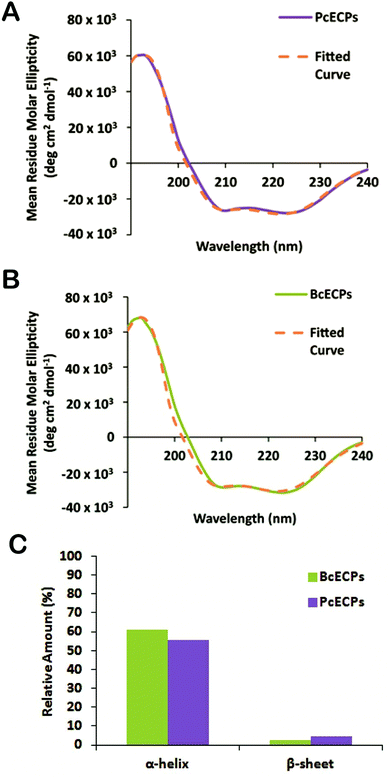 |
| Fig. 9 Circular dichroism of self-assembled ECPs. (A) CD spectrum of self-assembled PcECPs. (B) CD spectrum of self-assembled BcECPs in solution. (C) Estimated relative proportion of α-helix and β-sheet structure. | |
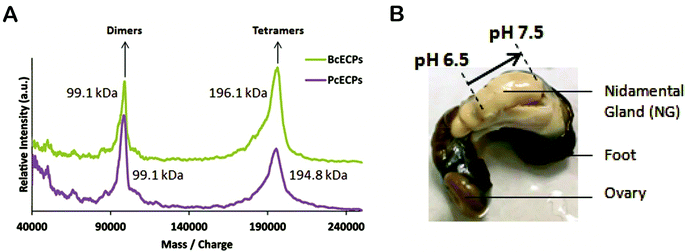 |
| Fig. 10 (A) MALDI-TOF mass spectra of self-assembled P. cochlidium and B. canaliculatus ECPs. (B) Image of the P. cochlidium nidamental gland indicating the locations of pH measurements and their respective values. | |
The apparent facile assembly of native ECPs represents an important milestone on the road toward the self-assembly of ECP-based materials through hierarchical oligomeric states. However, many open questions remain. For example, it will be key to learn about the dimensions of the fundamental native assembly unit and to determine (i) if they assemble preferably into dimers or trimers in vivo, (ii) if the native oligomers occur as homopolymers or heteropolymers, and (iii) whether the native ECP coiled-coils contain parallel, antiparallel or both types of ECP orientations. We also aim to understand the roles that specific domains and local motifs play in oligomerization. For example the N-terminal domain and trigger motifs of IFs are known to play an important role in dimer assembly40 and the possibility remains that several as yet unidentified sequence designs are encrypted in the ECP sequences that facilitate the process of their assembly. The role of potential chaperones must also be considered as well as the micro-environment that the proteins are subjected to in the nidamental gland, during export to the ventral pedal gland, and during the final sclerotization processing in the ventral pedal gland.
3.8 Toward biomimetic coiled-coil elastomers
The coiled-coil design offers significant potential for the engineering of a wide range of functional materials.10,12 Coiled-coil proteins have already found use in applications ranging from nano-scale sensors and switches to components of hydrogels and building blocks that facilitate ligand interactions. The primary sequence designs of ECPs therefore represent unique building blocks for coiled-coil based biomimetic engineering where their propensity for oligomerisation through multiple length scales may be exploited in a variety of applications. The unique combination of shock absorbing potential combined with reversible extensibility exhibited by the snail egg case represents highly desirable properties with a suite of potential applications and these properties may be tunable. For example, there are four main mechanical parameters that one can envisage controlling. These include the initial stiffness of the material, its transformation stress, the range of the elastic strain plateau, and the amount of absorbed elastic energy during a loading/unloading cycle.22 Notably, fibrin, myosin, IFs, hagfish threads and marine snail egg case membranes all contain coiled-coil motifs as a dominant motif, yet they exhibit distinct differences in their mechanical behavior, particularly during load cycling.22 Therefore, a detailed understanding of the relationship between primary sequence and mechanical properties of the egg case provides new biomimetic design strategies and unique insights into structure–function relationships of coiled-coil based materials in general.
In addition, a detailed understanding of the processing strategies used by marine snails to produce their egg capsules offers the potential to provide a foundation for biomimetic processing of ECP-based materials. The ECPs are synthesized in nidamental gland tissues and exported to the nidamental gland lumen where they are assembled in a concentrated viscous mixture whose rheological properties and meso-scale organization remain to be characterized. During egg case production this mixture of self-assembled proteins is transferred to the ventral pedal gland where the egg case precursor is likely subjected to cross-linking.26 To gain further insights into this processing mechanism, we used a micro-electrode to measure the pH within the nidamental gland (Fig. 10B). At the proximal end of the gland the pH was found to be 6.5 whereas further along the gland in the distal region, the pH was 7.5. Therefore there appears to be a precise level of control over glandular micro-environment during ECP assembly and/or processing. By comparison, spider dragline silk secretions are known to be subjected to a pH gradient along the length of the gland that likely influences protein solubility and β-sheet formation during spinning. In the case of silk glands, however, the pH was found to decrease.45 Clearly, knowledge of the unique micro-environmental conditions used by marine snails to process their elastomers can offer new insights into the conditions that could be used by bioengineers to efficiently assemble and arrange ECP-based filaments into useful load bearing structures. These natural processing strategies may ultimately be mimicked to engineer a range of functional materials including robust semi-permeable membranes, elements of composite materials and multifunctional elastomers with sensing or cell-binding functionalities for tissue engineering applications for example.
4. Conclusions
The mechanical properties and structural hierarchy of P. cochlidium egg cases were found to be very similar to those of other known marine snail egg cases. These observations provide a foundation for defining the key structural and functional elements in snail egg cases and we suggest that they also establish P. cochlidium as an important model organism for the study of α-helical solid–solid phase transforming elastomers. In addition, the primary egg case protein sequences from the different species were predicted to exhibit notable differences in coiled-coil domain organization that may influence the self-assembly, hierarchical organization and the α-to-β phase transformation potential of these materials. The precise orientation and degree of polypeptide packing of ECP-based fibrils in the native egg case remains an open question and could provide unique insights into the structure–function relationships of these materials. From a biomimetic engineering perspective the observed range of different local sequence designs and predicted domain architectures points to the possibility that several primary sequence designs may be exploited to engineer elastomers with elasticity driven by a solid–solid phase transformation. However, precise control over engineered ECP-based material properties will require a deeper understanding of the self-assembly mechanisms of these proteins, the role that localized sequence perturbations play in the α-to-β transition and the role of native physico-chemical processing conditions in egg case processing. Fortunately, genetic/protein engineering and decades of research on the assembly, structure and mechanics of IFs, among many other coiled-coil proteins, offer a strong foundation to experimentally approach these questions.
Acknowledgements
This research was funded by the Singapore National Research Foundation (NRF) through a NRF Fellowship awarded to A.M.
References
- E. Moutevilis and D. N. Woolfson, J. Mol. Biol., 2009, 726–732 CrossRef PubMed.
- O. J. L. Rackham, M. Madera, C. T. Armstrong, T. L. Vincent, D. N. Woolfson and J. Gough, J. Mol. Biol., 2010, 403, 480–493 CrossRef CAS PubMed.
- M. Pechar and R. Pola, Biotechnol. Adv., 2013, 31, 90–96 CrossRef CAS PubMed.
- R. Pola, R. Laga, K. Ulbrich, I. Sieglova, V. Kral, M. Fabry, M. Kabesova, M. Kovar and M. Pechar, Biomacromolecules, 2013, 14, 881–889 CrossRef CAS PubMed.
- C. Fortier, G. De Crescenzo and Y. Durocher, Biomaterials, 2013, 34, 1344–1353 CrossRef CAS PubMed.
- J. M. Fletcher, R. L. Harniman, F. R. Barnes, A. L. Boyle, A. Collins, J. Mantell, T. H. Sharp, M. Antognozzi, P. J. Booth, N. Linden, M. J. Miles, R. B. Sessions, P. Verkade and D. N. Woolfson, Science, 2013, 340, 595–599 CrossRef CAS PubMed.
- H. Chao, D. L. Bautista, J. Litowski, R. T. Irvin and R. S. Hodges, J. Chromatogr., B: Biomed. Sci. Appl., 1998, 715, 307–329 CrossRef CAS.
- H. Chao, M. E. Houston, Jr., S. Grothe, C. M. Kay, M. O'Connor-McCourt, R. T. Irvin and R. S. Hodges, Biochemistry, 1996, 35, 12175–12185 CrossRef CAS PubMed.
- R. R. Naik, S. M. Kirkpatrick and M. O. Stone, Biosens. Bioelectron., 2001, 16, 1051–1057 CrossRef CAS.
- D. N. Woolfson, Adv. Protein. Chem., 2005, 70, 79–112 CrossRef CAS.
- Y. Yang and P. Burkhard, J. Nanobiotechnol., 2012, 10, 42 CrossRef CAS PubMed.
- D. N. Woolfson and M. G. Ryadnov, Curr. Opin. Chem. Biol., 2006, 10, 559–567 CrossRef CAS PubMed.
- B. Apostolovic, D. Maarten and H.-A. Klok, Chem. Soc. Rev., 2010, 39, 3541–3575 RSC.
- D. D. Root, V. K. Yadavalli, J. G. Forbes and K. Wang, Biophys. J., 2006, 90, 2852–2866 CrossRef CAS PubMed.
- I. Schwaiger, C. Sattler, D. R. Hostetter and M. Rief, Nat. Mater., 2002, 1, 232–235 CrossRef CAS PubMed.
- Y. Taniguchi, B. S. Khatri, D. J. Brockwell, E. Paci and M. Kawakami, Biophys. J., 2010, 99, 257–262 CrossRef CAS PubMed.
- L. Kreplak, H. Bär, J. Leterrier, H. Herrmann and U. Aebi, J. Mol. Biol., 2005, 354 Search PubMed.
- H. Herrmann, H. Bär, L. Kreplak, S. V. Strelkov and U. Aebi, Nat. Rev. Mol. Cell Biol., 2007, 6, 562–573 CrossRef PubMed.
- J. W. Weisel, Adv. Protein. Chem., 2005, 247–299 CAS.
- A. E. X. Brown, R. I. Litvinov, D. E. Discher, P. K. Purohit and J. W. Weisel, Science, 2009, 325 Search PubMed.
- J. R. Houser, N. E. Hudson, L. Ping, E. T. O'Brien, R. Superfine, S. T. Lord and M. R. Falvo, Biophys. J., 2010, 99, 3038–3047 CrossRef CAS PubMed.
- A. Miserez and P. A. Guerette, Chem. Soc. Rev., 2013, 42, 1973–1995 RSC.
- A. Miserez, S. Wasko, C. Carpenter and J. H. Waite, Nat. Mater., 2009, 8, 910–916 CrossRef CAS PubMed.
- M. J. Harrington, S. Wasko, A. Masic, F. D. Fisher, H. S. Gupta and P. Fratzl, J. Royal Soc. Interface, 2012, 9, 2911–2922 CrossRef CAS PubMed.
- H. S. Rapoport and R. E. Shadwick, Biomacromolecules, 2002, 3, 42–50 CrossRef CAS PubMed.
- H. S. Rapoport and R. E. Shadwick, J. Exp. Biol., 2007, 210, 12–26 CrossRef CAS PubMed.
- P. A. Guerette, S. Hoon, Y. Seow, M. Raida, A. Masic, F. T. Wong, V. H. Ho, K. W. Kong, M. C. Demirel, A. Pena-Francesch, S. Amini, G. Z. Tay, D. Ding and A. Miserez, Nat. Biotechnol., 2013, 31, 908–915 CrossRef CAS PubMed.
- S. Wasko, G. Z. Tay, A. Schwaighofer, C. Nowak, J. H. Waite and A. Miserez, Biomacromolecules, 2014 DOI:10.1021/bm401598z.
- D. J. Colgan, W. F. Ponder, E. Beacham and J. Macaranas, Mol. Phylogenet. Evol., 2007, 42, 717–737 CrossRef CAS PubMed.
- K. S. Tan and C. L. Phuah, J. Mollus. Stud., 1999, 65, 499–501 CrossRef PubMed.
- A. Karunaratne, C. R. Esapa, J. Hiller, A. Boyde, R. Head, J. H. Bassett, N. J. Terrill, G. R. Williams, M. A. Brown, P. I. Croucher, S. D. Brown, R. D. Cox, A. H. Barber, R. V. Thakker and H. S. Gupta, J. Bone Miner. Res., 2012, 27, 876–890 CrossRef PubMed.
-
A. P. Hammersley, ESRF Internal Report, ESRF97HA02 T, FIT2D: An Introduction and Overview, 1997.
- T. Lefevre, M. E. Rousseau and M. Pézolet, Appl. Spectrosc., 2006, 60, 841–846 CrossRef CAS PubMed.
- M. A. Larkin, G. Blackshields, N. P. Brown, R. Chenna, P. A. McGettigan, H. McWilliam, F. Valentin, I. M. Wallace, A. Wilm, R. Lopez, J. D. Thompson, T. J. Gibson and D. G. Higgins, Bioinformatics, 2007, 23, 2947–2948 CrossRef CAS PubMed.
- A. M. Waterhouse, J. B. Procter, D. M. Martin, M. Clamp and G. J. Barton, Bioinformatics, 2009, 25, 1189–1191 CrossRef CAS PubMed.
- C. Cole, J. D. Barber and G. J. Barton, Nucleic Acids Res., 2008, 36(Suppl. 2), W197–W201 CrossRef CAS PubMed.
- C. T. Armstrong, T. L. Vincent, P. J. Green and D. N. Woolfson, Bioinformatics, 2011, 27, 1908–1914 CrossRef CAS PubMed.
- C. Louis-Jeune, M. Andrade-Navarro and C. Perez-Iratxeta, Proteins: Struct., Funct., Bioinf., 2011, 80, 374–381 CrossRef PubMed.
- H. Herrmann and S. V. Strelkov, BMC Biol., 2011, 9, 16 CAS.
- H. Herrmann and U. Aebi, Annu. Rev. Biochem., 2004, 73, 749–789 CrossRef CAS PubMed.
- M. Arslan, Z. Qin and M. J. Buehler, Comput. Methods Biomech. Biomed. Eng., 2011, 14, 1–7 CrossRef PubMed.
- N. J. Greenfield, Nat. Protocols, 2006, 1, 2876–2890 CAS.
- N. R. Zaccai, B. Chi, A. R. Thomson, A. L. Boyle, G. J. Bartlett, M. Bruning, N. Linden, R. B. Sessions, P. J. Booth, R. L. Brady and D. N. Woolfson, Nat. Chem. Biol., 2011, 7, 935–941 CrossRef CAS PubMed.
- C. Xu, R. Liu, A. K. Mehta, R. C. Guerrero-Ferreira, E. R. Wright, S. Dunin-Horkawicz, K. Morris, L. C. Serpell, X. Zuo, J. S. Wall and V. P. Conticello, J. Am. Chem. Soc., 2013, 135, 15565–15578 CrossRef CAS PubMed.
- C. Dicko, F. Vollrath and J. M. Kenney, Biomacromolecules, 2004, 5, 704–710 CrossRef CAS PubMed.
Footnotes |
† Electronic supplementary information (ESI) available. See DOI: 10.1039/c3bm60264h |
‡ These two authors contributed equally to this manuscript. |
|
This journal is © The Royal Society of Chemistry 2014 |
Click here to see how this site uses Cookies. View our privacy policy here.