DOI:
10.1039/C4BM00121D
(Paper)
Biomater. Sci., 2014,
2, 1222-1229
The bioactivity of composite Fmoc-RGDS-collagen gels
Received
12th April 2014
, Accepted 26th May 2014
First published on 4th June 2014
Abstract
The incorporation of small bioactive peptide motifs within robust hydrogels constitutes a facile procedure to chemically functionalise cell and tissue scaffolds. In this study, a novel approach to utilise Fmoc-linked peptide amphiphiles comprising the bio-functional cell-adhesion RGDS motif within biomimetic collagen gels was developed. The composite scaffolds thus created were shown to maintain the mechanical properties of the collagen gel while presenting additional bio-activity. In particular, these materials enhanced the adhesion and proliferation of viable human corneal stromal fibroblasts by 300% compared to non-functionalised gels. Furthermore, the incorporation of Fmoc-RGDS nanostructures within the collagen matrix significantly suppressed gel shrinkage resulting from the contractile action of encapsulated fibroblasts once activated by serum proteins. These mechanical and biological properties demonstrate that the incorporation of peptide amphiphiles provides a suitable and easy method to circumvent specific biomaterial limitations, such as cell-derived shrinkage, for improved performance in tissue engineering and regenerative medicine applications.
Introduction
The development of natural and synthetic biomaterials with applications in tissue engineering and regenerative medicine is a major area of academic and commercial interest. Biomaterials are often designed to reproduce the in vivo environment of tissues, namely by providing a surface that emulates the native extracellular matrix (ECM), and to which cells can attach and interact.1 Collagen gels are widely used as such biomaterials. At the molecular level, collagen is a triple helix of polypeptide chains that assemble hierarchically into highly ordered fibrils of ∼100 nm in diameter.2 Fibril assembly from isolated triple helical proteins can be replicated in vitro through appropriate changes in solution chemistry, with resulting fibrils forming gels with similar structural ordering to that found in vivo.3 The mechanical properties of collagen gels result from the molecular-level interactions between its comprising fibril network and the interstitial solution, typically, tissue culture medium. Upon gelation, collagen fibrils form a dense and highly entangled network that effectively resists shear and extension, whereas resistance to the interstitial flow of the solution through the network allows gels to withstand compressive loads.4 Thus, collagen gels form mechanically-stable scaffolds on which cells can attach and grow.5 Collagen gels have also been used extensively as three-dimensional (3D) scaffolds, allowing cells to be cultured while encapsulated to generate functional tissue constructs. Furthermore, the mechanical properties of collagen gels can be easily and precisely modulated using several methodologies, namely through plastic compression and chemical cross-linking.6 Despite their many suitable qualities as a biomaterial, the limitations of collagen gels should be considered. For example, in their naive form collagen gels do not contain all the biological cues necessary for extensively recreating particular native ECM environments, and consequently impair cell function and/or viability.7
Currently, the design of enhanced biomaterials is being supported by studies exploring the mechanisms controlling cell–ECM interactions. This knowledge has been successfully translated into improved surface chemistry functionalisation, i.e., the incorporation of biological motifs into biomaterials that serve as biological ligands, to better modulate cell behaviour.8 In recent years, a considerable number of motifs have been developed and tested in both fundamental and clinical research settings.9 These ligands have been used alone or in combination with other biomaterials to create artificial systems that better support growth and differentiation of cells and tissues in vitro.10–12 Among these artificial systems, peptide amphiphiles (PAs) represent a class of simple and versatile molecules that allow the incorporation of a multitude of functional motifs in self-assembling structures.13–15 Prominently, PAs comprising bioactive motifs linked to the aromatic N-(fluorenyl-9-methoxycarbonyl) (Fmoc) group have previously been investigated for the development of synthetic substrates with application in tissue engineering.16,17 Hydrogels containing the Fmoc group have been designed for rapid formulation via pH adjustments.18,19 Furthermore, previous work has proposed the use of Fmoc-PAs as 2D or 3D scaffolds for cell culture.16,20,21 In particular, it has been demonstrated that Fmoc-RGD peptide amphiphiles at 2 wt% can constitute a 2D scaffold for the support and growth of fibroblasts.18 In this context, the use of an RGDS motif was expected to result in a similarly-stable scaffold with significantly enhanced bioactivity.22,23 However, the mechanical and structural properties of these Fmoc-containing gels were found to be sub-optimal, forming scaffolds that often lack the durability required to adequately support cells for long periods of culture in vitro. Recently, it was reported that hydrogels produced from 2 wt% Fmoc-RGDS showed the onset of syneresis when subjected to shearing at low strains.24 Furthermore, these hydrogels were unable to withstand contact with cell culture media or temperatures at 37 °C without dissolving or becoming compliant. As such, we propose that self-assembling Fmoc-PAs can be used in combination with biologically-relevant scaffolds such as collagen gels to produce novel functional biomaterials with improved mechanical stability and increased/tuneable bioactivity.
Materials and methods
Preparation of PA-functionalised collagen gels
The bio-functional peptide amphiphile (fPA) comprised of the Fmoc molecule linked to the Arg-Gly-Asp-Ser (RGDS) cell adhesion peptide was supplied by CS Bio (Menlo Park, USA) and characterised elsewhere.24 The fPA was incorporated into collagen gels to create a composite biomaterial with increased functionality. To create these functionalised collagen gels, the lyophilised fPA was weighed, solubilised in ultrapure water at 1 wt% (1.52 × 10−2 M), and added to ice-cold rat tail collagen type-I (2 g L−1 in 0.6% acetic acid; First Link Ltd, UK) previously mixed with 10× Modified Essential Medium (Life Technologies, USA) and neutralised with 1 M NaOH at a 1
:
7
:
1
:
1 volume ratio, respectively. Non-functionalised (control) collagen gels were prepared by replacing the fPA solution with same volume of ultrapure water. The fPA-supplemented, and control collagen solutions were then cast into either 12 or 24 mm Ø Transwell plate inserts (Corning, USA), using 1 or 4 mL aliquots for the 1.12 or 4.67 cm2 surface areas, respectively, and allowed to polymerise at 37 °C and 5% CO2 for 30 minutes. The resulting gels were either maintained uncompressed or plastically-compressed as previously described,25 using a 134 g load for 5 minutes at room temperature.26 Fibronectin coating was performed by incubating non-functionalised compressed collagen gels with human fibronectin (R&D systems, USA). Gels were incubated overnight at room temperature with fibronectin solution (5 μg of protein per cm2 of gel surface), and then washed thrice with water to remove unabsorbed protein. Gels with encapsulated cells were produced as described above. In this instance, 1 wt% (1.52 × 10−2 M) fPA was solubilised in DMEM:F12 (Live Technologies, USA), and the resulting fPA solution was used to re-suspend cells.
Rheology of PA-functionalised collagen gels
The rheological properties of fPA-functionalised compressed and uncompressed collagen gels were investigated using a controlled stress AR-2000 rheometer (TA Instruments, USA) with a plate–plate geometry (20 mm Ø). Preliminary stress sweeps were carried out at a fixed frequency of 2π radians over a stress range of 1 × 10−1–102 Pa at 37 °C for all samples. Frequency sweep measurements were then performed at an angular frequency range of ×10−1–102 rad s−1 under a controlled stress corresponding to the linear viscoelastic region of each sample (0.7 and 1 Pa for uncompressed and compressed gels, respectively).
Expansion of cells on fPA-functionalised collagen gels in 2D culture
Human stromal corneal fibroblasts (hCSFs) from human corneal rings (kindly provided by Mr Martin Leyland, Royal Berkshire Hospital) were isolated as previously described,22 and seeded onto fPA-functionalised (+fPA), fibronectin-coated (+Fn), or non-functionalised (control) compressed collagen gels covering the entire surface of 24 mm Ø Transwell inserts. Briefly, trypsinised hCSFs were re-suspended in DMEM:F12 medium supplemented with 10% foetal bovine serum (FBS; Biosera, France), seeded onto the gels at a density of 1.5 × 104 cells per cm2 of gel surface, and maintained at 37 °C for 5 days. Culture media was replaced daily.
Live/dead double-staining of human corneal stromal fibroblasts (hCSFs)
The growth of hCSFs on fPA-functionalised compressed collagen gels was examined using the live/dead double-staining kit (Calbiochem, UK) following 5 days in culture. Briefly, the collagen gels were transferred onto microscope slides and incubated in the dark for 15 min at 37 °C with staining buffer supplemented with 1
:
1000 cyto-dye and propidium iodide solutions. Slides were then examined using an Axio Imager upright fluorescence microscope (Zeiss, Germany) coupled with a digital video camera (CoolSnap, RS Photometrics, USA) to observe the presence of live (green-stained) and dead (red-stained) cells. Fluorescence images (≤12 for each gel condition) were analysed using the ImageJ (v1.46) software to quantify the number of live and dead cells. Cell viability was subsequently calculated as the ratio between the number of live cells and the total number of cells, both live and dead. Cell proliferation was calculated as the number of live cells in each condition normalised by the number of live cells in non-functionalised (control) gels.
Encapsulation of hCSFs in fPA-functionalised collagen gels in 3D culture
Cells used for encapsulation were re-suspended in DMEM:F12 with or without 1 wt% fPA (+fPA or control gels, respectively) at 2 × 105 cells per mL of medium prior to collagen gel polymerization. Uncompressed gels were then transferred to 6-well polystyrene culture plates and maintained for seven days in DMEM:F12 without serum (SFM), with 5% FBS (+FBS), or supplemented with both serum and 5 × 10−5 M of fPA or cyclic-RGD peptide (cRGD; CS Bio). Total number of cells encapsulated within each gel condition was determined at day 7 using the Alamar Blue assay as previously described.22 Each condition was tested in triplicate, in three independent experiments.
Contraction of fPA-functionalised collagen gels
Uncompressed collagen gels containing encapsulated cells were imaged using a Nikon digital camera immediately after polymerisation and at day seven. Images were analysed using ImageJ (v.1.46) to determine the area of the gels, and contraction at day 7 calculated as percentage of initial gel area, and normalized by cell number. Each condition was tested in triplicate, in three independent experiments.
Immunofluorescence analysis
Encapsulated cells were fixed in 4% paraformaldehyde for 20 min, washed three times with PBS for 15 min, blocked for 1 h in PBS supplemented with 2% goat serum and 2% BSA. The 3D constructs were then incubated with anti-α-smooth muscle actin antibody (MAB1420, R&D systems) in blocking solution (1
:
1000) for 2 h, washed three times with PBS for 15 min, and incubated with 1
:
1000 goat anti-mouse IgG1 conjugated to Alexa 488 (A11001, Invitrogen) for an additional hour. Samples were mounted in VectaShield mounting media containing 4′,6-diamidino-2-phenylindole (DAPI) (Vector Labs, UK) to label cell nuclei and imaged using a AxioImager fluorescence microscope (Zeiss) coupled with a digital video camera (CoolSnap, RS Photometrics, USA).
Statistical analysis
Data (average ± S.D.) was evaluated using two-way ANOVA followed by Tukey's post-hoc tests. Significant differences were considered for p < 0.05.
Results and discussion
Mechanical characterisation of fPA-functionalised collagen gels
In this study we used the self-assembling Fmoc-Arg-Gly-Asp-Ser peptide amphiphile (henceforth designated fPA) in combination with compressed and uncompressed collagen type-I gels to create functionalised scaffolds presenting a more attractive environment for cell attachment and growth. The viscoelastic properties of fPA-functionalised gels were tested by rheology, as shown in Fig. 1. Compressed fPA-collagen gels were shown to be flexible, with the elastic modulus (G′) largely independent of shear frequency (Fig. 1). Notably, the elastic modulus values for compressed fPA-collagen gels reported here (Table 1) were comparable to the values obtained previously for non-functionalised compressed collagen gels.26 In contrast, uncompressed fPA-collagen gels showed increased elastic and viscous moduli (G′′) at higher shear frequencies (Fig. 1), an effect referred to as strain-stiffening. This effect is commonly observed in semi-flexible biological polymers, and has been associated with the capacity of tissues to prevent large deformations and consequent loss of integrity.27 Furthermore, the elastic modulus of uncompressed fPA-collagen gels (Table 1) was higher than that from non-functionalised gels at an equivalent angular frequency range,26 suggesting that the self-assembled nanostructures of fPA were integrated within the matrix formed by the collagen type-I fibrils. Taken together, these results showed that both compressed and uncompressed collagen gels maintained their mechanical structure and stability despite fPA functionalisation. Moreover, fPA functionalisation altered the mechanical properties of the uncompressed collagen gels, providing these constructs with the strain-stiffening attributes characteristic of organic tissues. This suggested that fPA can be incorporated into collagen gels for improved outcomes in a wide range of applications, particularly those involving the support of live cells.
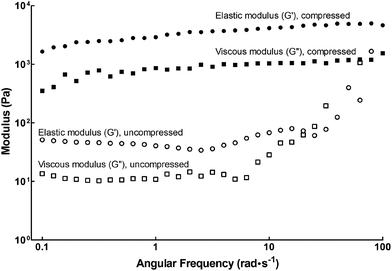 |
| Fig. 1 Rheology of fPA-functionalised collagen gels using frequency sweep measurements. Plastic compression altered the viscoelastic properties of fPA-functionalised gels, with compressed gels (black) having higher elastic (G′; circles) and viscous moduli (G′′; squares) compared to those left uncompressed (white symbols). | |
Table 1 Elastic modulus, G′ (Pa), of fPA-functionalised and non-functionalised collagen gels determined by shear rheology, using an angular frequency of 1 rad s−1
Collagen gels |
Elastic modulus, G′ (Pa) |
fPA-functionalised |
Non-functionalised26 |
Compressed |
2900 |
2900 |
Uncompressed |
40 |
2 |
Expansion and viability of cells grown on fPA-functionalised collagen gels
In order to test the capacity of fPA-collagen scaffolds to support cell adhesion and proliferation, human corneal stromal fibroblasts (hCSFs) were seeded onto functionalised (+fPA) and non-functionalised (control) collagen gels following plastic compression. In addition, non-functionalised compressed collagen gels were coated with fibronectin (+Fn) and used as positive controls for bioactivity. Fibronectin is a natural protein extensively used as surface coating5,28–30 to enhance cell attachment and promote cell proliferation and differentiation through the direct interaction between its cell-adhesion motif Arg-Gly-Asp (RGD) and several classes of integrins.10,31 Cells observed by phase-contrast microscopy 24 h after seeding were found attached to the surface of all collagen gels. After five days in culture, cells were then stained with calcein and propidium iodide and imaged by fluorescence microscopy (Fig. 2). As a cell-permeable dye, calcein is able to penetrate live cells, which then can be identified by emission of green fluorescence. In contrast, the red fluorescent propidium iodide dye is cell-impermeable, and is only incorporated by cells with porous membranes (i.e., dead cells). The analysis of images from the live/dead cell assay showed that +fPA gels significantly promoted the survival of hCSFs when compared to non-functionalised gels (Fig. 2). This effect corresponded to a 1.3-fold increase in the percentage of viable cells on +fPA (82 ± 7%) compared to control gels, where viability was significantly lower (61 ± 9%; Fig. 2b). In addition, the total number of cells growing on +fPA was significantly higher and corresponded to a 3-fold increase over control gels (Fig. 3). This indicated that the fPA nanostructures incorporated within the collagen gels were bio-functionally active, and that their RGDS motifs were capable of interacting with human cells to promote attachment and proliferation. This interpretation was supported by the differences in cell morphology observed between +fPA and control gels. Cells grown on functionalised composite gels appeared more elongated and spindle-shaped (Fig. 2a, arrows) compared to hCSFs on non-functionalised collagen gels, which appeared more rounded. The characteristic anchorage-dependent, elongated phenotype is controlled in vivo by interaction between cell adhesion molecules expressed at the cell membrane (e.g., integrins) and their corresponding binding partners present in the surrounding ECM. Previous studies have shown that, in vitro, the stiffness of compressed collagen substrates affects cell–substrate adhesion.26,32 However, and as discussed above, the mechanical properties of compressed collagen gels were unchanged by fPA functionalisation, indicating that enhanced cell adhesion and proliferation on +fPA gels was due to incorporation of the bio-functional RGDS motif carried by the fPA nanostructures, followed by downstream activation of integrin-mediated signalling pathways.33 This conclusion was reinforced by the prevalence of spindle-shaped cells on +Fn gels (Fig. 2a). The fibronectin-coated gels also enhanced hCSF proliferation significantly compared to the controls, although at lower levels than on +fPA (Fig. 3). However, this coating did not affect cell viability when compared to control gels (Fig. 2b). These discrepancies may be due to the lower density of functional motifs in +Fn compared to fPA-functionalised gels.
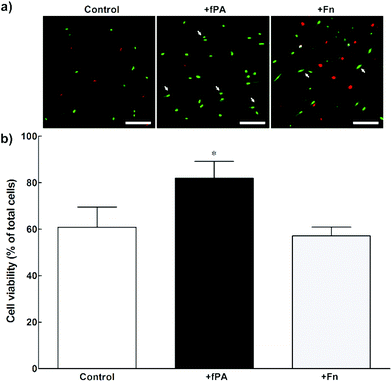 |
| Fig. 2 Viability of human corneal stromal fibroblasts (hCSFs) cultured on compressed collagen gels. Cells grown for five days on uncoated (control), fPA-functionalised (+fPA), or fibronectin-coated collagen gels (+Fn) were (a) imaged by fluorescence microscopy after calcein (green)/propidium iodide (PI, red) double staining. The arrows indicate spindle-shaped cells. Scale bars, 200 μm. (b) The percentage of live, calcein-positive/PI-negative cells was quantified for all three conditions, with differences in viability evaluated by two-way ANOVA followed by Tukey's post-hoc tests (average ± S.D. from three independent experiments, n = 3; *corresponded to p < 0.05). | |
 |
| Fig. 3 Effect of fPA in the proliferation of human corneal stromal fibroblasts (hCSFs) cultured on compressed collagen gels. The total number of live cells grown for five days on uncoated (control), fPA-functionalised (+fPA), or fibronectin-coated collagen gels (+Fn) was quantified and expressed as a percentage of control (uncoated compressed collagen gels). Differences in cell numbers were evaluated by two-way ANOVA followed by Tukey's post-hoc tests (average ± S.D. from three independent experiments, n = 3; * and ** corresponded to p < 0.05 and 0.01, respectively). | |
Contraction of fPA-functionalised collagen gels containing encapsulated cells
Collagen gels used as scaffolds for cell encapsulation or as vehicles for tissue grafting are usually prone to remodelling resulting from intrinsic or extrinsic activity of cells. Most commonly, collagen gels change their shape irreversibly in response to the contractile action of encapsulated cells.34 Consequently, transplanted collagen gels may contract, leading to delayed graft integration,35 tissue deformation,36 and scar contracture.37,38 In order to determine the ability of fPA functionalisation in circumventing this effect, we tested changes in shape from +fPA and control collagen gels containing encapsulated hCSFs. These cells were employed for this assay because they can efficiently differentiate from a non-contractile to a highly-contractile, alpha-smooth muscle actin (αSMA)-positive, phenotype simply by being incubated in FBS-supplemented culture medium (+FBS). Conversely, incubation of hCSFs in serum-free media (SFM) maintains the cells in a non-contractile, quiescent phenotype, where αSMA-positive stress fibres are absent.39 Uncompressed gels were used for this particular experiment to facilitate contraction whilst maintaining cell attachment and growth. Moreover, the uncompressed gels showed to be semi-flexible (Fig. 1), with mechanical properties comparable to other natural cellular and ECM polymers occurring in vivo (e.g., F-actin,40 vimentin,41 and fibrin42). After seven days in SFM culture, contraction of collagen gel was minimal, and independent of fPA functionalisation (Fig. 4a). In contrast, reduction of gel size was maximised in serum-containing conditions (+FBS) (Fig. 4). Nevertheless, the contraction of +fPA gels (to 28 ± 2% of the initial gel area) was significantly inhibited when compared to non-functionalised gels, which were reduced to just 6 ± 2% of their initial size (Fig. 4b). Collagen gel contraction was further inhibited by the supplementation of +FBS culture media with soluble fPA (+sPA) below the critical aggregation concentration24 or cyclic-RGD peptide (+cRGD) to block integrins from hCSFs. The use of such blockers is a well-described method to inhibit integrin-dependent cell binding to RGD-containing substrates.22 Both soluble factors significantly impaired contraction of fPA-functionalised and control gels, although this inhibition was much more evident for +fPA constructs (Fig. 4). These results were in line with previous studies where the inhibition of integrins reduced the contractile force exerted by cardiac myocytes,43 Chinese hamster ovary cells,44 mesangial cells,45 and human dermal fibroblasts.46
 |
| Fig. 4 Effect of fPA functionalisation in collagen gel contraction. Human corneal stromal fibroblasts (hCSFs) were encapsulated within PA-functionalised (+fPA) or non-functionalised (control) uncompressed collagen gels and cultured for seven days with serum-free (SFM) or serum-containing medium (+FBS), to avoid or elicit gel contraction, respectively. Inhibition of gel contraction through blocking of hCSFs’ integrins was also performed by adding 50 μM of soluble PA or cyclic-RGD peptide to serum-containing medium (+sPA or +cRGD, respectively). (a) Representative photographs of collagen gels at day 7 were superimposed over images taken from the same gels immediately after formation at day 0 (traced line). Scale bars, 4 mm. (b) Contraction at day 7 was evaluated relatively to each gel's initial area at day 0, and differences in size within differently-treated control (i) or +PA gels (ii), and between control and +PA gels (iii) were evaluated by two-way ANOVA followed by Tukey's post-hoc tests (average area ± S.D. from three independent experiments, n = 3; *, **, and *** corresponded to p < 0.05, 0.01, and 0.001, respectively). | |
The phenotype of encapsulated cells in fPA-functionalised gels
The levels of gel contraction were well correlated with the phenotype of the encapsulated cells. When observed by phase-contrast microscopy at day 7, encapsulated hCSFs cultured in SFM showed a dendritic morphology characteristic of non-contractile, quiescent cells (Fig. 5a, arrows), whereas cells in +FBS were extended and flattened (Fig. 5a), assuming the shape of myofibroblasts. Differences between non-contractile and contractile myofibroblastic phenotypes were confirmed by immunofluorescence microscopy (Fig. 5b), where SFM cells were αSMA-negative and +FBS cells exhibited defined αSMA-positive stress fibres. Gels maintained in +FBS supplemented with either +sPA or +cRGD had αSMA-positive cells (Fig. 5b). However, composite fPA-collagen gels incubated with integrin blockers still exhibited dendritic, non-contractile cells (Fig. 5a, arrows), whereas no dendritic hCSFs remained in +sPA or +cRGD control gels. Taken together, these results indicated that collagen gel contraction was induced by the contractile activity of myofibroblastic hCSFs, and that fPA prevented, in part, the formation of spindle-shaped cells and the action of such contractile cells on the collagen gel matrix.
 |
| Fig. 5 Effect of fPA functionalisation in the activation of human corneal stromal fibroblasts (hCSFs) cultured within collagen gels. The hCSFs were encapsulated within PA-functionalised (+fPA) or non-functionalised (control) uncompressed collagen gels and cultured for seven days with serum-free (SFM) or serum-containing medium (+FBS), to avoid or elicit cell activation, respectively. The effect of integrin blocking on hCSF activation was also tested by adding 50 μM of soluble PA or cyclic-RGD peptide to serum-containing medium (+sPA or +cRGD, respectively). (a) Representative phase-contrast micrographs of gels at day 7, with dendritic-shaped hCSFs (arrows). Scale bars, 50 μm. (b) Immunofluorescence microscopy analysis of collagen gels stained with anti-αSMA antibody (green) and DAPI (cyan). Scale bars, 20 μm. | |
Previously, materials developed to inhibit contraction in collagen-based gels were focused on functionalisation using glycosaminoglycans36,47 or hyaluronic acid (HA).37 Our approach differed, as it involved the combination of self-assembled structures of synthetic fPA within a collagen type-I gel matrix. The presence of fPA nanostructures not only enhanced adhesion, proliferation, and viability of hCSFs but also allowed contractile cells to be present within the collagen gels whilst minimising contraction of the biomaterial. These powerful effects might be due to the level of association between the fPA structures and the collagen fibres. Previous studies have shown that functionalising nanostructures within a 3D structural gel create a composite material performing as a scaffold-within-a-scaffold to which cells preferentially adhere.48 For example, HA gels were shown to improve cell attachment and proliferation by the non-cross-linked addition of recombinant gelatin.49 The collagen fibres forming the main structural component of our composite gel were not chemically cross-linked to fPA. This allowed the fPA-bound myofibroblastic hCSFs to contract whilst minimising the effects on the overall shape and density of the collagen matrix. Moreover, integrin blocking through the addition of soluble fPA and/or cRGD was also shown to reduce composite gel contraction. The direct inhibition of RGD-binding hCSF integrins such as αVβ3 and αVβ550 would impair interactions between these cells and their surrounding matrices. Therefore, inhibition of these integrins may also impair TGFβ1-mediated contractile activity, as recently reported with human cardiac fibroblasts.51 We propose that, in the future, the study of fPA interactions with collagen fibrils and cell integrins might contribute to better understand the pathways involved in the regulation of the cellular response to matrix mechanics.
Conclusions
This work demonstrates an alternative use of PAs comprising the Fmoc group coupled with bioactive motifs. When combined with collagen, the fPA provided functional cues incorporated within a polymerised scaffold, and produced a novel composite biomaterial that enhanced cell attachment, proliferation, and viability through specific cell–substrate interactions. This may be a more practical alternative application of Fmoc-PAs due to their hydrolytic capabilities under basic pH conditions. Furthermore, both compressed and uncompressed collagen gels have tissue-like qualities that make them highly amenable for use in transplantation. The use of fPA was shown to prevent the contraction resulting from intrinsic cellular activity in functionalised collagen scaffolds. This work highlights for the first time the enhanced bioactivity of amino acid sequences in functionalised collagen gels for use as biomaterials for engineering artificial tissue structures.
Acknowledgements
We are grateful to the BBSRC (grant number BB/I008187/1) for financial support. We kindly acknowledge M. Leyland, Royal Berkshire Hospital for supplying the post-mortem corneal rings.
Notes and references
- E. S. Place, N. D. Evans and M. M. Stevens, Nat. Mater., 2009, 8, 457–470 CrossRef CAS PubMed.
- B. R. Williams, R. A. Gelman, D. C. Poppke and K. A. Piez, J. Biol. Chem., 1978, 253, 6578–6585 CAS.
- K. E. Kadler, D. F. Holmes, J. A. Trotter and J. A. Chapman, Biochem. J., 1996, 316(Pt 1), 1–11 CAS.
- M. J. Buehler, Proc. Natl. Acad. Sci. U. S. A., 2006, 103, 12285–12290 CrossRef CAS PubMed.
- K. von der Mark and J. Park, Prog. Mater. Sci., 2013, 58, 327–381 CrossRef CAS PubMed.
- L. Cen, W. Liu, L. Cui, W. Zhang and Y. Cao, Pediatr. Res., 2008, 63, 492–496 CrossRef CAS PubMed.
- S. Gomes, I. B. Leonor, J. F. Mano, R. L. Reis and D. L. Kaplan, Prog. Polym. Sci., 2012, 37, 1–17 CrossRef CAS PubMed.
- M. P. Lutolf, P. M. Gilbert and H. M. Blau, Nature, 2009, 462, 433–441 CrossRef CAS PubMed.
- Q. Chen, S. Liang and G. A. Thouas, Prog. Polym. Sci., 2013, 38, 584–671 CrossRef CAS PubMed.
- U. Hersel, C. Dahmen and H. Kessler, Biomaterials, 2003, 24, 4385–4415 CrossRef CAS.
- A. Mahler, M. Reches, M. Rechter, S. Cohen and E. Gazit, Adv. Mater., 2006, 18, 1365–1370 CrossRef CAS.
- S. L. Mi, A. L. David, B. Chowdhury, R. R. Jones, I. W. Hamley, A. M. Squires and C. J. Connon, Tissue Eng., Part A, 2012, 18, 373–381 CrossRef CAS PubMed.
- I. W. Hamley, Chem. Rev., 2012, 112, 5147–5192 CrossRef CAS PubMed.
- D. A. Harrington, E. Y. Cheng, M. O. Guler, L. K. Lee, J. L. Donovan, R. C. Claussen and S. I. Stupp, J. Biomed. Mater. Res. A, 2006, 78, 157–167 CrossRef PubMed.
- J. B. Matson and S. I. Stupp, Chem. Commun., 2012, 48, 26–33 RSC.
- V. Jayawarna, S. M. Richardson, A. R. Hirst, N. W. Hodson, A. Saiani, J. E. Gough and R. V. Ulijn, Acta Biomater., 2009, 5, 934–943 CrossRef CAS PubMed.
- M. Zhou, A. M. Smith, A. K. Das, N. W. Hodson, R. F. Collins, R. V. Ulijn and J. E. Gough, Biomaterials, 2009, 30, 2523–2530 CrossRef CAS PubMed.
- G. Cheng, V. Castelletto, R. R. Jones, C. J. Connon and I. W. Hamley, Soft Matter, 2011, 7, 1326–1333 RSC.
- K. Thornton, A. M. Smith, C. L. R. Merry and R. V. Ulijn, Biochem. Soc. Trans., 2009, 37, 660–664 CrossRef CAS PubMed.
- T. Liebmann, S. Rydholm, V. Akpe and H. Brismar, BMC Biotechnol., 2007, 7 Search PubMed.
- Y. Wang, Z. Zhang, L. Xu, X. Li and H. Chen, Colloids Surf.,B, 2013, 104, 163–168 CrossRef CAS PubMed.
- R. M. Gouveia, V. Castelletto, S. G. Alcock, I. W. Hamley and C. J. Connon, J. Mater. Chem. B, 2013, 1, 6157–6169 RSC.
- M. J. Webber, J. Tongers, M. A. Renault, J. G. Roncalli, D. W. Losordo and S. I. Stupp, Acta Biomater., 2010, 6, 3–11 CrossRef CAS PubMed.
- V. Castelletto, C. M. Moulton, G. Cheng, I. W. Hamley, M. R. Hicks, A. Rodger, D. E. Lopez-Perez, G. Revilla-Lopez and C. Aleman, Soft Matter, 2011, 7, 11405–11415 RSC.
- R. A. Brown, M. Wiseman, C. B. Chuo, U. Cheema and S. N. Nazhat, Adv. Funct. Mater., 2005, 15, 1762–1770 CrossRef CAS.
- R. R. Jones, I. W. Hamley and C. J. Connon, Stem Cell Res., 2012, 8, 403–409 CrossRef CAS PubMed.
- C. Storm, J. J. Pastore, F. C. MacKintosh, T. C. Lubensky and P. A. Janmey, Nature, 2005, 435, 191–194 CrossRef CAS PubMed.
- P. A. Harper and R. L. Juliano, J. Cell Biol., 1980, 87, 755–763 CrossRef CAS.
- J. Sottile, D. C. Hocking and P. J. Swiatek, J. Cell Sci., 1998, 111, 2933–2943 CAS.
- T. P. Cooper and M. V. Sefton, Acta Biomater., 2011, 7, 1072–1083 CrossRef CAS PubMed.
- D. J. Leahy, I. Aukhil and H. P. Erickson, Cell, 1996, 84, 155–164 CrossRef CAS.
- E. Hadjipanayi, V. Mudera and R. A. Brown, J. Tissue Eng. Regen. Med., 2009, 3, 77–84 CrossRef CAS PubMed.
- R. O. Hynes, Cell, 2002, 110, 673–687 CrossRef CAS.
- J. Pedersen and M. Swartz, Ann. Biomed. Eng., 2005, 33, 1469–1490 CrossRef PubMed.
- H. Lin, Y. Yang, Y. Wang, L. Wang, X. Zhou, J. Liu and D. Peng, PLoS One, 2014, 9, e85672 Search PubMed.
- W. C. Hsu, M. H. Spilker, I. V. Yannas and P. A. Rubin, Invest. Ophthalmol. Vis. Sci., 2000, 41, 2404–2411 CAS.
- S. Y. Lee, J. H. Oh, J. C. Kim, Y. H. Kim, S. H. Kim and J. W. Choi, Biomaterials, 2003, 24, 5049–5059 CrossRef CAS.
- J. M. Patterson, A. J. Bullock, S. MacNeil and C. R. Chapple, Eur. Urol., 2011, 60, 856–861 CrossRef PubMed.
- J. L. Funderburgh, M. M. Mann and M. L. Funderburgh, J. Biol. Chem., 2003, 278, 45629–45637 CrossRef CAS PubMed.
- T. G. Mason, T. Gisler, K. Kroy, E. Frey and D. A. Weitz, J. Rheol., 2000, 44, 917–928 CrossRef CAS.
- M. Schopferer, H. Bar, B. Hochstein, S. Sharma, N. Mucke, H. Herrmann and N. Willenbacher, J. Mol. Biol., 2009, 388, 133–143 CrossRef CAS PubMed.
- W. Qi, B. Anindita, P. W. Jessamine, Y. Arjun and A. J. Paul, New J. Phys., 2007, 9, 428 CrossRef.
- V. Sarin, R. D. Gaffin, G. A. Meininger and M. Muthuchamy, J. Physiol., 2005, 564, 603–617 CrossRef CAS PubMed.
- J. Jokinen, E. Dadu, P. Nykvist, J. Kapyla, D. J. White, J. Ivaska, P. Vehvilainen, H. Reunanen, H. Larjava, L. Hakkinen and J. Heino, J. Biol. Chem., 2004, 279, 31956–31963 CrossRef CAS PubMed.
- S. Kagami, S. Kondo, K. Loster, W. Reutter, T. Kuhara, K. Yasutomo and Y. Kuroda, J. Am. Soc. Nephrol., 1999, 10, 779–789 CAS.
- R. A. Brown, K. K. Sethi, I. Gwanmesia, D. Raemdonck, M. Eastwood and V. Mudera, Exp. Cell Res., 2002, 274, 310–322 CrossRef CAS PubMed.
- E. Garcia-Gareta, N. Ravindran, V. Sharma, S. Samizadeh and J. F. Dye, BioRes. Open Access, 2013, 2, 412–420 CrossRef PubMed.
- X. Xin, A. Borzacchiello, P. A. Netti, L. Ambrosio and L. Nicolais, J. Biomater. Sci. Polym. Ed., 2004, 15, 1223–1236 CrossRef CAS PubMed.
- A. Tuin, J. Zandstra, S. G. Kluijtmans, J. B. Bouwstra, M. C. Harmsen and M. J. Van Luyn, Eur. Cell. Mater., 2012, 24, 320–330 CAS.
- S. K. Masur, J. K. Cheung and S. Antohi, Invest. Ophthalmol. Vis. Sci., 1993, 34, 2690–2698 CAS.
- V. Sarrazy, A. Koehler, M. Chow, E. Zimina, C. X. Li, H. Kato, C. A. Caldarone and B. Hinz, Cardiovasc. Res., 2014, 102, 407–417 CrossRef CAS PubMed.
Footnote |
† These authors contributed equally to this paper. |
|
This journal is © The Royal Society of Chemistry 2014 |
Click here to see how this site uses Cookies. View our privacy policy here.