DOI:
10.1039/C4BM00143E
(Paper)
Biomater. Sci., 2014,
2, 1412-1418
On-demand generation of singlet oxygen from a smart graphene complex for the photodynamic treatment of cancer cells†
Received
25th April 2014
, Accepted 27th May 2014
First published on 4th July 2014
Abstract
Graphene oxide (GO) has been proven to be a highly efficient long-range quencher for various fluorescence processes, which intrinsically work through a photophysical mechanism similar to that of singlet oxygen generation (SOG). Under our hypothesis that GO may be capable of quenching the SOG process, here we design and synthesize a novel nanocomplex consisting of GO, a photosensitizer and an aptamer. We demonstrate that GO is an ideal SOG controller, which can reversibly quench and recover SOG depending on the interaction intensity between GO and a photosensitizer. Additionally, it can simultaneously act as a carrier for the efficient loading and delivery of the photosensitizers to cancer cells. Thus, during the delivery process, SOG of the nanocomplex can be completely inhibited by the quenching capacity of the GO even though there is light present; however, when the nanocomplex enters into cancer cells where target molecules are present, SOG is triggered by a target binding event and singlet oxygen is reversibly released from the nanocomplex, ultimately inducing significant cell death in the presence of light. This proof-of-concept study provides a new chemical strategy for creating a highly selective photodynamic therapy with low toxicity, using hydrophilic GO-based systems.
1. Introduction
Photodynamic therapy (PDT) has emerged as a non-invasive therapeutic strategy that uses photosensitizers (PSs), light and molecular oxygen for the local treatment of various diseases including cancer.1–4 When illuminated by an appropriate light source, the excited PSs transfer their energy to surrounding molecular oxygen, generating cytotoxic reactive oxygen species, such as singlet oxygen (1O2), that can effectively kill cancer cells in situ. Therefore, PDT offers many advantages over conventional therapeutics, such as high levels of effectiveness, localized and specific tumor treatment and the ability to repeat the therapy without any cumulative toxicity.5,6 However, there are still many drawbacks in current PDTs for clinical applications.7,8 In practice, many of the commonly used PSs possess a hydrophobic nature and thus tend to aggregate in physiological solutions, creating great challenges for delivery processes and biocompatibility issues, and also causing unwanted collateral damage to normal tissues. In addition, the lifetime and diffusion range of 1O2 from photosensitizers (PSs) used in conventional PDT are very short: <200 ns and ∼20 nm, respectively.9,10 Thus, it is essential to establish a chemical strategy for a hydrophilic PS system with controllable singlet oxygen generation (SOG), to minimize harm to healthy cells/tissues, and enhance the PDT’s selectivity and efficacy.
To fully or partly meet the above requirements, nanomaterials such as gold, silica and carbon nanotubes have been proposed as vehicles for the delivery of PDT agents.10–13 Among them, graphene oxide (GO) has been implemented as a novel nanomaterial in the field of biomedicine. Owing to its unique 2-dimensional structure, abundant functional groups, large surface area, good biocompatibility and low cost,14,15 GO has been widely explored as a novel vehicle for the delivery of drugs and biomolecules, such as siRNA and aptamers, to target tissues.16–18 This, along with its ability for highly efficient passive targeting of tumors,19 means that using GO as a nanocarrier may enhance PS payload and improve tumor uptake compared to free PSs. Several groups have successfully employed GO as a carrier of PSs, such as Chlorin e6 (Ce6) and hyaluronic acid, for killing tumor cells in vitro by light irradiation. Unfortunately, it is still difficult to optimally regulate the SOG of PSs using a GO-based delivery system. Thus, it is rather important to further develop a GO-based photoactive switchable carrier for PDT, which may minimize the damage to healthy tissues.6,20–22 Recently, GO has been proved to be a highly efficient long-range quencher for various fluorescent probes.23 Considering that the photophysical mechanism of SOG should be similar to that of the fluorescence process (Fig. S1†), we hence hypothesize that GO may be capable of quenching SOG. If this idea works, GO can function as a “cage” for controlling SOG, by manipulating the distance between the GO and the PSs. Meanwhile, it can also be used as the vehicle for efficient delivery of PSs to the diseased tissues, ultimately delivering controllable and enhanced PDT effects.
In this work, we firstly engineered a novel nanocomplex that combined a PS, an aptamer and GO, for controllable SOG (named GO/AP-PS). Fig. 1 outlines how we incorporated the significant features of the GO, aptamer and PS to form a simple yet rather efficient and elegant PDT agent. First, the aptamer was synthesized and then covalently attached to a PS (AP-PS). Second, by means of π-stacking interactions, the PS-functionalized aptamer could strongly adsorb onto the GO surface. In the absence of a target molecule, PS would closely adhere to the GO surface, causing both fluorescence and SOG to be almost completely shut down. Significantly, the high affinity of the aptamer for the target can lead to an alteration of the structure conformation of the aptamer upon target binding.24 Therefore, upon the addition of the target, the binding between this and the aptamer will disturb the interaction of the aptamer and the GO, leading to the release of PSs from the GO surface and the restoration of SOG by the PSs for PDT applications. As a result, we realize the regulation of SOG by target molecule binding.
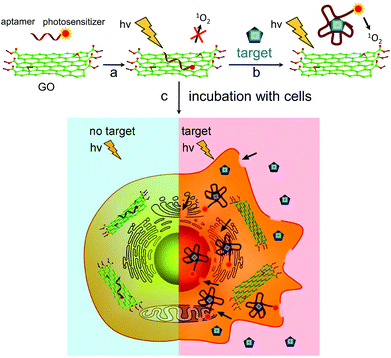 |
| Fig. 1 Schematic representation of the nanocomplex and the regulation of SOG upon target binding in solution and in cells: (a) AP-PS and GO were mixed together to form a GO/AP-PS nanocomplex. (b) Restoration of SOG due to target binding. (c) Left: GO inhibits SOG without target molecules in cells; right: GO restores SOG when target molecules are present, finally killing cancer cells in situ. | |
2. Experimental procedure
2.1. Materials
Graphite powder (99%) was purchased from Alfa Aesar. Adenosine 5′-triphosphate (ATP, ≥99%), uridine 5′-triphosphate (UTP, ≥96%), cytidine 5′-triphosphate (CTP, ≥95%), and guanosine 5′-triphosphate (GTP, ≥90%) were supplied by Sigma-Aldrich. Singlet oxygen sensor green (SOSG) and Hoechst were obtained from the Life Technologies Co. A reactive oxygen species assay kit was provided by the Beyotime Institute of Biotechnology (Shanghai, China). Other reagents were obtained from the Beijing Chemical Reagent Co. All the chemicals used in this work were of analytical grade and were used as received without further purification. Fresh deionized water was used throughout the experiments.
Chlorin e6 (Ce6)-labeled ATP aptamer (AP-Ce6), complementary DNA (cDNA) and random DNA (rDNA) were purchased from the Takara Biotechnology Co. Ltd (Dalian, China). The nucleic acids were high performance liquid chromatography (HPLC)-purified and freeze-dried. Stock solutions of DNA (10 μM) were prepared with Tris-HCl buffer (10 mM, containing 150 mM NaCl and 10 mM KCl, pH 7.40).
2.2. Preparation of graphene oxide
Graphite oxide was synthesized using a modified Hummers method according to previous reports.17,25 Then, the as-prepared graphite oxide dispersion (∼2.0 mg ml−1) was sonicated by an ultrasonic probe (650 W, 35%) for 4 h in an ice bath to obtain a stable GO dispersion. After sonication, the dispersion solution was centrifuged to exclude any graphite oxide residue and large GO sheets.
2.3. Characterization
Fluorescence measurements were recorded using a Horiba JobinYvon FluoroLog3 spectrometer (Japan). Tapping atomic force microscopy (AFM) images of the GO were taken with a scanning probe microscope (SPM, Multimode 8, Bruke, USA) under ambient conditions. Fluorescence microscopy images were obtained using an inverted fluorescence microscope (Olympus IX71). To measure the extent of co-localization between the fluorescence signals of Ce6 and dichlorodihydrofluorescein (DCF), an ImageJ plugin was used for the calculation of Pearson's correlation (Rr) and the overlap coefficient (R). An overlap coefficient of higher than 0.6 indicates co-localization.
2.4. Test of fluorescence quenching ability of the graphene oxide
In a typical measurement, the stock solution of AP-Ce6 was diluted 100-fold with Tris-HCl buffer (final concentration of AP-Ce6 was 100 nM), and then mixed with a certain concentration of GO. After the mixture was incubated at 37 °C for 1 h, the fluorescence was recorded from 600 nm to 700 nm with an excitation at 404 nm. As shown in Fig. S2,† the fluorescence intensity of the AP-Ce6 decreased sharply as the concentration of GO increased from 1 μg ml−1 to 4 μg ml−1. When the GO concentration reached 5 μg ml−1, the fluorescence intensity of the AP-Ce6 was quenched down to ca. 1.14% of the original fluorescence signal. As a result, 5 μg ml−1 was taken as the optimized concentration for GO. In these conditions, the loading ratio of AP-Ce6 to GO was ca. 23.1%.
2.5. Validation of fluorescence response and singlet oxygen generation of the nanocomplex towards ATP in solution
AP-Ce6 and GO were mixed in Tris-HCl buffer, and then the mixtures were incubated at 37 °C for 1 h to obtain the nanocomplex (GO/AP-Ce6). Thereafter, different concentrations of ATP were added to the above solution and the resulting mixtures were again incubated at 37 °C for 1 h. Finally, the fluorescence was measured with an excitation wavelength of 404 nm.
To evaluate the SOG of the GO/AP-Ce6 in solution, SOSG was used as an indicator for 1O2 by fluorescence enhancement. In a typical procedure, after incubation at 37 °C for 1 h, the mixture was centrifuged to remove any GO, which might have some effect on the detection of SOG. Then, SOSG was introduced into the above solution of GO/AP-Ce6 at a concentration of 2.0 μM. The mixture was placed in a cuvette and irradiated at 404 nm, which was the maximum absorption of Ce6, for 10 min. Finally, the fluorescence of SOSG was read out by measuring the fluorescence increase at 527 nm.
2.6. Selectivity
In order to investigate the amount of fluorescence restoration and SOG of the GO/AP-Ce6 which were caused by ATP alone, 0.1 mM each of ATP, GTP, CTP and UTP were mixed with GO/AP-Ce6. Thereafter, the mixtures were incubated at 37 °C for 1 h, and then the fluorescence of Ce6 was measured using the method described above. To test the SOG ability of the GO/AP-Ce6 after incubation with the ATP analogues, SOSG was added to the pre-treated solutions, and then the mixtures were transferred into cuvettes and irradiated at 404 nm for 10 min. The SOSG fluorescence was read out spectrophotometrically using a similar protocol to that suggested above.
2.7. Cell culture and incubation conditions
HepG2 (human hepatocellular liver carcinoma cell line) cells were purchased from Wuhan Boster Biological Technology Ltd (Wuhan, China). The cells were cultured in Iscove's Modified Dulbecco's Medium (IMDM), supplemented with 20% fetal bovine serum (FBS, Gibco, Gland Island, NY, USA), in a humidified atmosphere of 5% CO2 at 37 °C, and the medium was changed every day. Untreated cells were kept in the dark or under light irradiation, and taken as the reference standard. All the reported concentrations refer to the free AP-Ce6 equivalents.
2.8.
In vitro cytotoxic assay
To determine the cell viability under dark conditions (i.e. without light treatment), the cells were seeded in 96-well plates with a density of 4000 cells per well and cultured in IMDM under a humidified atmosphere with 5% CO2 at 37 °C for 24 h. Then seven groups of cell samples were set up as follows: group 1 was cells only (control); group 2 had GO added to the cells (labeled as GO); group 3 was incubated with ATP (labeled as ATP); group 4 had AP-Ce6 and GO added (labeled as AP-Ce6 + GO), group 5 was incubated with AP-Ce6 (labeled as AP-Ce6); group 6 was incubated with GO/AP-Ce6 (labeled as GO/AP-Ce6); and group 7 contained both GO/AP-Ce6 and ATP (labeled as GO/AP-Ce6 + ATP). Each group had five parallel samples. After 24 h incubation at 37 °C with 5% CO2, the cells were washed twice with phosphate buffered saline (PBS, pH 7.40), and then 200 μL of medium containing methyl thiazolyl tetrazolium tracer (MTT, 5 mg ml−1) was added into each well. Once the cells had been further incubated for 4 h at 37 °C, the medium was removed, and replaced with 150 μL of dimethylsulfoxide (DMSO) to dissolve the MTT tetrazolium dye. After shaking for 10 min, the fluorescence of each well was then measured on a microplate reader (SpectraMax M2 MDC, USA) using 480 nm excitation and 530 nm emission wavelength. Throughout this process, the cells were kept in a dark environment without any light exposure.
To determine the cell viability under exposure to light, the cells were plated into wells with a density of 4000 cells per well. After being incubated for 6 h at 37 °C, the seven groups were treated with a 650 nm light source at a power density of 50 mW cm−2, for 20 min. After incubation for another 24 h, the standard MTT assay, as mentioned above, was carried out to determine the cell viability.
2.9. Validation of fluorescence response and singlet oxygen generation of the nanocomplex towards ATP in cancer cells
A reactive oxygen species assay kit was applied to determine the level of intracellular 1O2. Briefly, the cells were seeded in 96-well culture plates and allowed to attach for 24 h. Then, after the medium was discarded and washed three times with PBS, 100 μL of fresh medium containing dihydrodichlorofluoresceindiacetate (DCFH-DA) was added into each well and the plates were incubated at 37 °C for 20 min. Subsequently, the medium was discarded and washed with PBS again. Then, the cells were treated with the seven conditions mentioned above, and incubated for another 6 h at 37 °C. Next, the seven groups were irradiated with a 650 nm light source at a power of 50 mW cm−2. After irradiation for 20 min, the cells were washed twice with PBS and the fluorescence of the DCF was measured on a microplate reader using 488 nm excitation and 525 nm emission wavelength. Cells treated with Rosup (a positive control reagent, 50 μg ml−1) for 20 min were used as a positive control.
All of the above experiments were repeated at least in triplicate and were statistically significant (p < 0.05).
3. Results and discussion
GO nanosheets were synthesized by the sonication-assisted exfoliation of graphite oxide, and possessed a highly hydrophilic nature with a narrow size distribution (ca. 80 nm, Fig. 2). AFM measurements reveal that the thickness of the GO nanosheets is ∼1.2 nm, indicating that single-layer GO nanosheets were obtained.26 Ce6 was used as the PS owing to its high photosensitizing efficacy.27,28 To test the feasibility of GO for the regulation of SOG, ATP was used as a proof-of-concept target molecule. ATP is produced on the extracellular surface of cancer cells besides the mitochondrial matrix and is related to a large number of diseases.29,30 Therefore, an ATP-specific linker was designed and then linked to the Ce6.
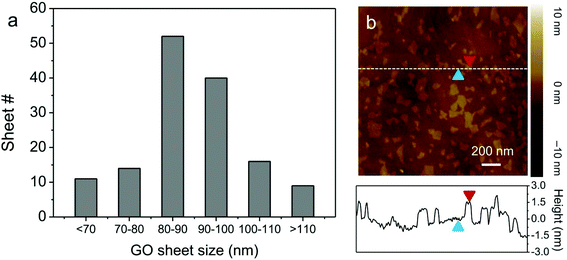 |
| Fig. 2 Characterization of the GO nanosheets. (a) The lateral size histogram for the GO nanosheets observed by AFM. (b) AFM image and height profile of the GO nanosheets. The line profile for the nanosheets is marked by the white line in the image, indicating the thickness of the GO nanosheets. | |
The AP-Ce6 exhibits strong fluorescence emission in Tris-HCl buffer (pH 7.40) under 404 nm excitation. However, as expected, its fluorescence gradually decreases with the addition of GO in increasing concentrations (Fig. S2†). For example, up to 99% quenching of the fluorescence emission is observed when the concentration of GO is increased to 5 μg ml−1. However, after the addition of ATP (0.1 mM), the fluorescence is largely retained due to the formation of an aptamer–ATP complex, which is increased by more than 18 times compared to that of the AP-Ce6 in the presence of GO. These results imply that the interaction of AP-Ce6 and GO is much weaker than that of AP-Ce6 and ATP, which causes the AP-Ce6 to detach from the GO surface, ultimately resulting in the retention of its fluorescence (Fig. 3a). That is to say, the 1O2 production ability of the AP-Ce6 is largely restored after the addition of the target molecule. SOG was subsequently assayed by incubating the sample with SOSG and then irradiating at 404 nm for 10 min. SOG is evaluated in terms of the fluorescence intensity changes (F1/F0 − 1) of SOSG, where F0 and F1 are fluorescence intensities at 527 nm in the absence and presence of ATP, respectively, after subtracting the buffer background. In the case of GO/AP-Ce6 alone, no noticeable fluorescence enhancement of SOSG was found (Fig. 3a). Meanwhile, after the introduction of ATP (0.1 mM), the SOSG fluorescence of GO/AP-Ce6 exhibited a 25-fold enhancement. Furthermore, as the ATP concentration increased, the SOSG fluorescence was intensified, indicating more 1O2 production after irradiation. When the concentration of ATP reached up to 0.5 mM, no further fluorescence increase could be observed, as it had reached a plateau. It is worth noting that, when using free Ce6 instead of AP-Ce6, the quenching effect of SOG from the GO/Ce6 complex appears to be less drastic compared to that of the GO/AP-Ce6 complex, which is in agreement with the report by Liu et al.6 (Fig. S3†). Conclusively, these data support the hypothesis that both fluorescence and SOG of AP-Ce6 can be efficiently inhibited by GO. More significantly, they can be reversibly and quantitatively recovered on specific target treatments (Fig. 3b and Fig. S4†).
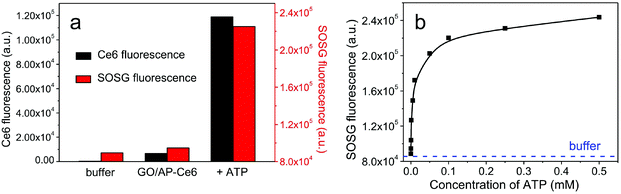 |
| Fig. 3 SOG regulation by an aptamer-binding target. (a) The Ce6 and SOSG signal readouts after 10 min of irradiation with excitation at 404 nm. (b) The SOSG signal plotted as a function of the target molecule (ATP) concentration. The blue dotted line indicates the buffer's SOSG signal. | |
It is known that aptamers have a high binding affinity and specificity.31,32 To test this, GTP, CTP and UTP were used to evaluate the selectivity and target specificity of the as-prepared GO/AP-Ce6. The results show that GO/AP-Ce6 exhibits a much lower fluorescence enhancement response to the three analogues compared to its response to ATP (Fig. 4). This suggests that the 1O2 production from GO/AP-Ce6 is indeed only triggered by ATP, without any detectable interference from the other analogues.
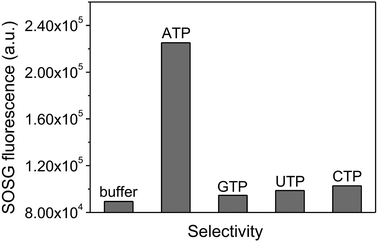 |
| Fig. 4 SOG specificity. The 1O2 production of GO/AP-Ce6 shows a high selectivity to ATP over different ATP analogues: GTP, UTP, and CTP. | |
We further evaluated the ability of GO/AP-Ce6 to generate 1O2 in cells. Human HepG2 cells were first incubated with AP-Ce6 (0.4 μM) and GO/AP-Ce6 + ATP (0.4 μM GO/AP-Ce6 and 40 μM ATP) for 6 h, respectively, and then irradiated with light (∼650 nm) for 20 min. The intracellular 1O2 levels in the HepG2 cells were then measured using the DCFH-DA method. The cells treated with Rosup were presented as a positive control. As shown in Fig. 5, AP-Ce6 and GO/AP-Ce6 + ATP triggered an increase in the 1O2 amount in the cells by 245% and 411%, respectively. As a control, HepG2 cells were also cultured with GO/AP-Ce6, and almost no increase of 1O2 was observed in each sample. This is in good agreement with the previous investigations in the solution, further confirming that SOG of GO/AP-Ce6 could be triggered by the addition of ATP. More evidence was obtained by fluorescence microscopy studies of the HepG2 cells after drug incubation (Fig. 6 and Fig. S5†). Fluorescence images show a strong fluorescence of Ce6 in HepG2 cells incubated with AP-Ce6 and GO/AP-Ce6 + ATP. However, HepG2 cells treated with or without GO/AP-Ce6 did not exhibit Ce6 fluorescence. Furthermore, the high co-localization of DCF and Ce6 fluorescence further demonstrates that GO/AP-Ce6 is internalized near the cell nuclei and then AP-Ce6 is cleaved by ATP, whereas almost no cleavage is found in the cells incubated with GO/AP-Ce6 (Table S1†). These results indicate that GO/AP-Ce6 can enter cells, and that its cleavage is specifically mediated by ATP at the cell level.
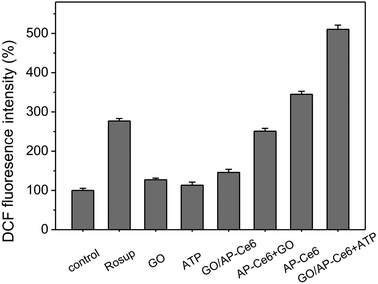 |
| Fig. 5 DCF-fluorescence intensity in HepG2 cells after treatment with the nanocomplex. | |
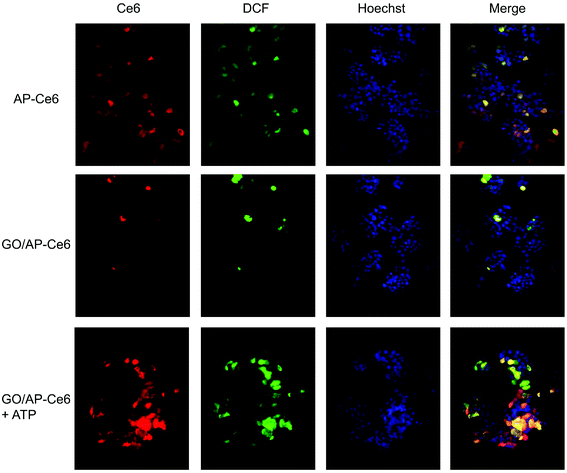 |
| Fig. 6 Fluorescence microscopy images of HepG2 cells incubated with AP-Ce6, GO/AP-Ce6 and GO/AP-Ce6 + ATP. | |
Next, we investigated the PDT efficacy of GO/AP-Ce6. A standard MTT assay was applied to determine the relative cell viability 6 h after various treatments, normalized to the viability of cells not treated with the drug or light (Fig. 7). Without light exposure, nearly all of the cells were viable, exhibiting negligible dark toxicity (Fig. 7a). Upon PDT treatment, GO/AP-Ce6 presented limited photodynamic cytotoxicity in the HepG2 cells; however, GO/AP-Ce6 + ATP significantly reduces the viability of the HepG2 cells (Fig. 7b). These data indicate that GO/AP-Ce6 is specifically photoactivated by ATP, and that its photodynamic cytotoxicity is ATP sequence-specific. Moreover, it was found that the cells treated with GO/AP-Ce6 + ATP exhibited an enhanced cytotoxicity compared to the HepG2 cells treated with AP-Ce6. This advance can probably be attributed to both the improved cellular uptake of Ce6 with the assistance of GO, and the efficient protection of the aptamers from enzymatic cleavage.33 The fluorescence images clearly indicate that GO/AP-Ce6 + ATP treated cells show stronger Ce6 and DCF fluorescence compared to the cells treated with AP-Ce6, corresponding to a greater uptake of Ce6 by cancer cells and more 1O2 production. Similarly, the PDT efficiency of GO/AP-Ce6 against MDA, HeLa and ECV cells is better than that of AP-Ce6 alone (Fig. S6†).
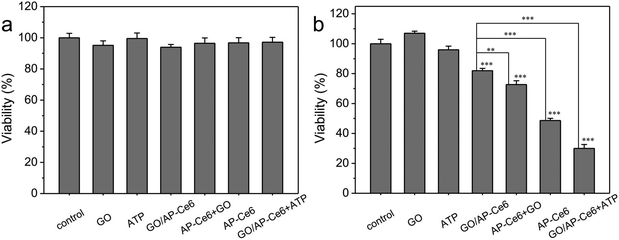 |
| Fig. 7 The cellular viability of HepG2 cells treated without (a) and with (b) light (t test, *p < 0.05; **p < 0.01; ***p < 0.001). | |
4. Conclusion
In conclusion, we engineered a novel molecular nanocomplex of a PS, an aptamer and GO for controllable SOG. The fluorescence and photoactivity of the PS loaded on the GO surface are quenched by the GO in aqueous solution and cells, but are reversibly recovered after introducing a target molecule, which can bind to the aptamer to weaken the interaction between the aptamer and GO. This results in the release of the adsorbed PS from GO. Moreover, this nanocomplex shows an enhanced uptake by HepG2 cells compared to free PS, and in the absence of light it exhibits no major toxicity towards cells. In contrast, it induces significant cell death only in the presence of target molecules (e.g., after it has reached the target sites) with light irradiation. These findings demonstrate that GO can function as a “cage” capable of controlling 1O2 generation by the strong interaction between the target molecules and the AP-PS, which is stronger than the binding force between AP-PS and GO.
The present system displays several important advantages: (i) the design for the selective regulation of SOG is versatile, since different aptamer sequences can be designed for different target molecules (Fig. S7 and 8†). (ii) It is a hydrophilic system that possesses a better biocompatibility than hydrophobic SOG systems. (iii) The use of GO as a nanocarrier to load drugs has been experimentally proved to be able to enhance drug loading efficiency and tumor uptake, and to protect aptamers from enzymatic cleavage. This directly results in an enhancement of the PDT efficiency and minimizes its side-effects. (iv) The GO/AP-Ce6 nanocomplex has the potential to integrate with contrast agents and theranostic drugs to form a theranostic agent, which would enable a multimodal biomedical imaging system with cancer therapy by PDT.
Acknowledgements
This work was supported by the National Basic Research Programs of China (973 program, no. 2012CB932504), and the National Natural Science Foundation of China (no. 21320102003, 21001108 and 21177128).
References
- J. Chen, K. Stefflova, M. J. Niedre, B. C. Wilson, B. Chance, J. D. Glickson and G. Zheng, Protease-triggered photosensitizing beacon based on singlet oxygen quenching and activation, J. Am. Chem. Soc., 2004, 126, 11450–11451 CrossRef CAS PubMed.
- M. Colilla, B. González and M. Vallet-Regí, Mesoporous silica nanoparticles for the design of smart delivery nanodevices, Biomater. Sci., 2013, 1, 114–134 RSC.
- D. K. Chatterjee, L. S. Fong and Y. Zhang, Nanoparticles in photodynamic therapy: An emerging paradigm, Adv. Drug Delivery Rev., 2008, 60, 1627–1637 CrossRef CAS PubMed.
- N. M. Idris, M. K. Gnanasammandhan, J. Zhang, P. C. Ho, R. Mahendran and Y. Zhang, In vivo photodynamic therapy using upconversion nanoparticles as remote-controlled nanotransducers, Nat. Med., 2012, 18, 1580–1585 CrossRef CAS PubMed.
- D. E. J. G. J. Dolmans, D. Fukumura and R. K. Jain, Photodynamic therapy for cancer, Nat. Rev. Cancer, 2003, 3, 380–387 CrossRef CAS PubMed.
- B. Tian, C. Wang, S. Zhang, L. Feng and Z. Liu, Photothermally enhanced photodynamic therapy delivered by nano-graphene oxide, ACS Nano, 2011, 5, 7000–7009 CrossRef CAS PubMed.
- K. Baba, H. E. Pudavar, I. Roy, T. Y. Ohulchanskyy, Y. Chen, R. K. Pandey and P. N. Prasad, New method for delivering a hydrophobic drug for photodynamic therapy using pure nanocrystal form of the drug, Mol. Pharm., 2007, 4, 289–297 CrossRef CAS PubMed.
- L. Zhou, W. Wang, J. Tang, J.-H. Zhou, H.-J. Jiang and J. Shen, Graphene oxide noncovalent photosensitizer and its anticancer activity in vitro, Chem. – Eur. J., 2011, 17, 12084–12091 CrossRef CAS PubMed.
- T. Takemura, N. Ohta, S. Nakajima and I. Sakata, Critical importance of the triplet lifetime of photosensitizer in photodynamic therapy of tumor, Photochem. Photobiol., 1989, 50, 339–344 CrossRef CAS PubMed.
- H. Park and K. Na, Conjugation of the photosensitizer Chlorin e6 to pluronic F127 for enhanced cellular internalization for photodynamic therapy, Biomaterials, 2013, 34, 6992–7000 CrossRef CAS PubMed.
- M. Ferrari, Cancer nanotechnology: opportunities and challenges, Nat. Rev. Cancer, 2005, 5, 161–171 CrossRef CAS PubMed.
- Z. Zhu, Z. Tang, J. A. Phillips, R. Yang, H. Wang and W. Tan, Regulation of singlet oxygen generation using single-walled carbon nanotubes, J. Am. Chem. Soc., 2008, 130, 10856–10857 CrossRef CAS PubMed.
- S. Y. Park, H. J. Baik, Y. T. Oh, K. T. Oh, Y. S. Youn and E. S. Lee, A smart polysaccharide/drug conjugate for photodynamic therapy, Angew. Chem., Int. Ed., 2011, 50, 1644–1647 CrossRef CAS PubMed.
- L. Yan, F. Zhao, S. Li, Z. Hu and Y. Zhao, Low-toxic and safe nanomaterials by surface-chemical design, carbon nanotubes, fullerenes, metallofullerenes, and graphenes, Nanoscale, 2011, 3, 362–382 RSC.
- L. Yan, Y. B. Zheng, F. Zhao, S. J. Li, X. F. Gao, B. Q. Xu, P. S. Weiss and Y. L. Zhao, Chemistry and physics of a single atomic layer: strategies and challenges for functionalization of graphene and graphene-based materials, Chem. Soc. Rev., 2012, 41, 97–114 RSC.
- Z. Liu, J. T. Robinson, X. Sun and H. Dai, PEGylated nanographene oxide for delivery of water-insoluble cancer drugs, J. Am. Chem. Soc., 2008, 130, 10876–10877 CrossRef CAS PubMed.
- L. Yan, Y.-N. Chang, L. Zhao, Z. Gu, X. Liu, G. Tian, L. Zhou, W. Ren, S. Jin, W. Yin, H. Chang, G. Xing, X. Gao and Y. Zhao, The use of polyethylenimine-modified graphene oxide as a nanocarrier for transferring hydrophobic nanocrystals into water to produce water-dispersible hybrids for use in drug delivery, Carbon, 2013, 57, 120–129 CrossRef CAS PubMed.
- K. Yang, L. Feng, X. Shi and Z. Liu, Nano-graphene in biomedicine: Theranostic applications, Chem. Soc. Rev., 2013, 42, 530–547 RSC.
- K. Yang, S. Zhang, G. Zhang, X. Sun, S.-T. Lee and Z. Liu, Graphene in mice: Ultrahigh in vivo tumor uptake and efficient photothermal therapy, Nano Lett., 2010, 10, 3318–3323 CrossRef CAS PubMed.
- P. Huang, C. Xu, J. Lin, C. Wang, X. Wang, C. Zhang, X. Zhou, S. Guo and D. Cui, Folic Acid-conjugated Graphene Oxide loaded with Photosensitizers for Targeting Photodynamic Therapy, Theranostics, 2011, 1, 240–250 CrossRef CAS PubMed.
- L. Zhou, H. Jiang, S. Wei, X. Ge, J. Zhou and J. Shen, High-efficiency loading of hypocrellin B on graphene oxide for photodynamic therapy, Carbon, 2011, 50, 5594–5604 CrossRef PubMed.
- F. Li, S.-J. Park, D. Ling, W. Park, J. Y. Han, K. Na and K. Char, J. Mater. Chem. B, 2013, 1, 1678–1686 RSC.
- E. Morales-Narváez and A. Merkoçi, Graphene oxide as an optical biosensing platform, Adv. Mater., 2012, 24, 3298–3308 CrossRef PubMed.
- C. H. Lu, H. H. Yang, C. L. Zhu, X. Chen and G. N. Chen, A graphene platform for sensing biomolecules, Angew. Chem., Int. Ed., 2009, 48, 4785–4787 CrossRef CAS PubMed.
- W. S. Hummers and R. E. Offeman, Preparation of graphitic oxide, J. Am. Chem. Soc., 1958, 80, 1339–1339 CrossRef CAS.
- X. Li, G. Zhang, X. Bai, X. Sun, X. Wang, E. Wang and H. Dai, Highly conducting graphene sheets and Langmuir-Blodgett films, Nat. Nanotechnol., 2008, 3, 538–542 CrossRef CAS PubMed.
- J. D. Spikes and J. C. Bommer, Photosensitizing properties of mono-l-aspartyl chlorin e6 (NPe6): A candidate sensitizer for the photodynamic therapy of tumors, J. Photochem. Photobiol., B, 1993, 17, 135–143 CrossRef CAS.
- R. Rotomskis, J. Valanciunaite, A. Skripka, S. Steponkene, G. Spogis, S. Bagdonas and G. Streckyte, Complexes of functionalized quantum dots and chlorin e6 in photodynamic therapy, Lith. J. Phys., 2013, 53 Search PubMed.
- T.-C. Huang, H.-Y. Chang, C.-H. Hsu, W.-H. Kuo, K.-J. Chang and H.-F. Juan, Targeting therapy for breast carcinoma by ATP synthase inhibitor aurovertin B, J. Proteome Res., 2008, 7, 1433–1444 CrossRef CAS PubMed.
- Y. He, Z.-G. Wang, H.-W. Tang and D.-W. Pang, Low background signal platform for the detection of ATP: When a molecular aptamer beacon meets graphene oxide, Biosens. Bioelectron., 2011, 29, 76–81 CrossRef CAS PubMed.
- D. E. Huizenga and J. W. Szostak, A DNA aptamer that binds adenosine and ATP, Biochemistry, 1995, 34, 656–665 CrossRef CAS.
- X. Liu, F. Wang, R. Aizen, O. Yehezkeli and I. Willner, Graphene oxide/nucleic-acid-stabilized silver nanoclusters: Functional hybrid materials for optical aptamer sensing and multiplexed analysis of pathogenic DNAs, J. Am. Chem. Soc., 2013, 135, 11832–11839 CrossRef CAS PubMed.
- Z. Tang, H. Wu, J. R. Cort, G. W. Buchko, Y. Zhang, Y. Shao, I. A. Aksay, J. Liu and Y. Lin, Constraint of DNA on functionalized graphene improves its biostability and specificity, Small, 2010, 6, 1205–1209 CrossRef CAS PubMed.
Footnote |
† Electronic supplementary information (ESI) available. See DOI: 10.1039/c4bm00143e |
|
This journal is © The Royal Society of Chemistry 2014 |
Click here to see how this site uses Cookies. View our privacy policy here.