DOI:
10.1039/C4BM00161C
(Minireview)
Biomater. Sci., 2014,
2, 1145-1153
Microsphere based scaffolds for bone regenerative applications
Received
7th May 2014
, Accepted 10th May 2014
First published on 12th June 2014
Abstract
Microspheres are characteristically flowing particles composed of inorganic and/or polymeric materials. Conventionally, microspheres are used as vehicles for controlled release because they can overcome the drawbacks of conventional therapy and enhance the efficacy of the given drug. Recently, due to the rapid development of tissue engineering, microspheres have been used in tissue engineering scaffolds. In microsphere-based/containing scaffolds, microspheres play an important role in both drug release and cell residence. There are two types of microspheres mainly exhibiting scaffold function, which are microsphere sintered or dissolved scaffolds and microsphere incorporated scaffolds. Taken together, the development of microsphere-based/containing scaffolds may open a new door for constructing drug release scaffolds for bone regenerative applications.
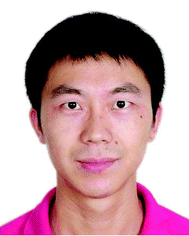 Wei Huang | Wei Huang is a research fellow in the Research Institute of Tsinghua University in Shenzhen and Shenzhen Lando Biomaterials Co, Ltd. He received his masters degree from the South China University of Technology. At that time, he received an outstanding diploma thesis award in Guangdong province. His core interests are in drug carriers and bone scaffolds, and he currently directs projects on controlled release biomaterials and maxillofacial applications. |
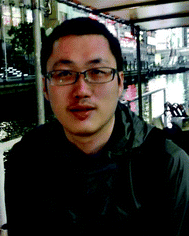 Xuetao Shi | Xuetao Shi graduated from the South China University of Technology (China) in 2010. After obtaining his PhD, he moved to Japan and worked in Tohoku University as a Research Associate. He was promoted as an Assistant Professor in WPI-AIMR, Tohoku University in 2012. His research interests mainly focus on developing polymer microspheres for various biomedical applications. Additionally, he also works on biomicrofluidics and tissue engineering. |
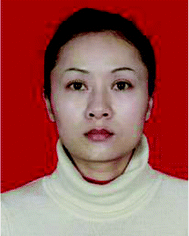 Chen Lai | Chen Lai completed her PhD in materials science in Hunan University in 2006. From 2006 to 2008 she conducted her postdoctoral research at the South China University of Technology. Then, she moved to the Shenzhen Key Laboratory of Human Tissue Regeneration and Repair in Peking University as a researcher. Her research interest focuses on developing new methods to design and synthesize inorganic biomaterials and inorganic/polymer composite biomaterials for bone tissue engineering applications. |
Introduction
Bone regeneration is a complex and dynamic process that includes recruiting osteogenic cells such as mesenchymal stem cells and osteoblastic cells, cell differentiation and proliferation, bone matrix formation, and finally, bone remodeling.1–3 Bone tissue engineering aims to support new functional bone regeneration via the systematical combination of biomaterials, cells, and growth factors. Three-dimensional scaffolds are the first factor in bone tissue engineering, as they serve as porous niches for cell residence and vehicles for the delivery of functional molecules (growth factors, cell differentiation factors, and proteins) to promote bone reconstruction.4–7 Various types of methods have been developed to fabricate 3D scaffolds with high porosity, good biocompatibility and mechanical strength. Among them, microsphere scaffolds have attracted most attention in recent years because microspheres are excellent vehicles for drug release. Therefore, using microspheres to make three-dimensional scaffolds would be an ideal choice in terms of both drug release and cell residence.8 By briefly summarizing three-dimensional microsphere scaffolds, we hope more attention might be drawn and more achievements would be realized in this field.
Sintered microsphere three-dimensional scaffolds for bone tissue engineering
In 2001, Borden et al. developed sintered microsphere scaffolds (SMSs), which combine the advantages of microspheres for controlled release with the capacity of porous scaffolds for cell loading and delivery.9 In addition, compared with conventional methods for producing three-dimensional scaffolds, SMS methods can significantly enhance the initial mechanical properties of the developed scaffolds. The mechanical properties such as compressive strength are nearly equal to those of cancellous bone.10
A schematic for the preparation of SMSs is shown in Fig. 1. Currently, there are two methods to fabricate SMSs. The difference in these two routes is the method of fusing the microspheres together.11,12 The first route accomplishes this via heating. Briefly, we obtain polymer microspheres by traditional single/double emulsification. Then, the microspheres are poured into a Teflon mold and heated to a specific temperature above the polymer's glass transition temperature (Tg) for several hours, and subsequently the mold is removed to obtain the SMS. The second route uses a solvent to bond microspheres together. Different solvents including methylene chloride and acetone can be used to fuse polymer microspheres together. Compared with heat sintering, solvent sintering provides an alternative and milder method for inserting active molecule-laden microspheres into the SMS.
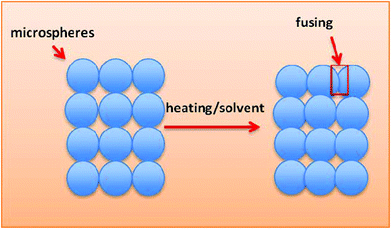 |
| Fig. 1 Schematic of the generation of PLGA sintered microsphere scaffolds. | |
It is well-known that the mechanical properties and porosity of scaffolds are of primary importance for bone regeneration. To obtain SMSs based on polymer microspheres with the appropriate porous structure and mechanical properties, several factors should be taken into consideration. These factors include the polymer's physical properties, such as Tg, viscosity, molecular weight, crystallinity and surface tension.13 The solvent concentration for solvent sintering and the heating conditions for heat sintering are also crucial factors. Higher solvent concentration, higher heating temperature, and extended heating time lead to a stronger bonding thanks to the stronger surface fusion of the microspheres.11 Although the stronger bonding will achieve an SMS with superior mechanical properties, the smaller porous diameter and lower porosity will hinder cell migration and vascularization. Hence, regulating the sintering conditions (temperature and time) allows the development of SMSs with an adaptable porous structure and mechanical characteristics for bone regeneration.
Poly(lactic-co-glycolic acid) (PLGA) is one of the most popular biomaterials for SMSs because of its good biocompatibility and degradability, as well as its excellent drug loading and delivery capabilities.14–16 In addition, polyphosphazenes, poly(ε-caprolactone) (PCL) and chitosan are also used for preparing SMSs due to their comparability to PLGA.17 Compared with traditional tissue engineering scaffolds, PLGA SMSs not only inherit the superior characteristics of PLGA and PLGA microspheres (such as their excellent degradation, processability and drug release), but they also possess other features, such as superior mechanical properties and porosity.18–21 Kofron et al.22,23 investigated the gradation of PLGA SMSs generated by amorphous and semi-crystalline PLGA. Both of the scaffolds exhibited a median porosity and pore size that mimic those of native bone. After six months of being implanted into created bone defects in rabbits, the SMSs from amorphous PLGA, which contained D,L-lactide, facilitated the generation of mineralized tissue in the matrix interior more so than the SMS produced by semi-crystalline PLGA SMS, which contained L-lactide, indicating that amorphous PLGA is beneficial for bone regeneration.
PLGA/lecithin sintered microsphere three-dimensional scaffolds for bone tissue engineering
Synthetic biopolymers such as PLGA, polylactic acid (PLA), and PCL lack moieties for cell attachment; therefore, modification of these polymeric matrices is highly required.24,25 However, because most of these polymers can only be dissolved in organic solvents, such as acetone and methylene chloride, using natural macromolecules such as collagen or gelatin to modify these synthetic polymers is very difficult. Though surface modification of synthetic microspheres using bioactive molecules such as collagen, gelatin, fibronectin has been widely reported, most of them are used in other fields rather than bone regeneration, so they are not discussed here. In the near future, these bioactive molecules would be an ideal option for creating composite sintered microsphere scaffolds. Lecithin is a composite of phosphatidylserine, phosphatidylcholine, phosphatidylethanolamine, and inositolphosphatides and is widely used in the food industry.26 Lecithin can be easily dissolved in various organic solvents. Shi et al.27,28 incorporated lecithin into bulk PLGA to improve its bioactivity and controlled release. Although lecithin has been considered a bioactive biomacromolecule, incorporating higher contents of lecithin into PLGA decreases the cell attachment and proliferation because the incorporation of higher lecithin contents into PLGA/lecithin composites will significantly enhance their surface hydrophilicity. It is well-known that biomaterials with moderate wettability (a water contact angle between 40° and 70°) favor cell attachment. For 20% (w/w) lecithin-incorporated PLGA, the water contact angle of the composite is nearly zero, as opposed to that of pristine PLGA (a water contact angle of approximately 85°). To achieve an ideal surface of PLGA/lecithin SMSs, Shi et al.28 optimized the content of lecithin in bulk PLGA. They found that incorporating 5% (w/w) lecithin into bulk PLGA decreased the wettability to the ideal range for cell attachment. Osteoblasts were seeded onto PLGA/lecithin scaffolds with varying lecithin contents (0, 5%, 10% and 20%), and it was found that the SMSs with 20% lecithin not only promoted cell attachment and proliferation but also remarkably enhanced the expression of specialized osteogenic markers, including alkaline phosphatase activity and calcium deposition, as well as the gene expression of type I collagen and ALP. The controlled release of the PLGA/lecithin SMSs was also studied, and the PLGA/lecithin SMSs showed good protein and drug encapsulation efficiency and similar release kinetics compared to pure PLGA SMSs, indicating that PLGA/lecithin SMSs are promising for protein/drug controlled release-based tissue engineering therapeutics for bone. Additionally, incorporating lecithin into PLGA SMSs does not compromise the excellent mechanical properties of the scaffolds.
PLGA/hydroxyapatite sintered microsphere three-dimensional scaffolds for bone tissue engineering
Hydroxyapatite (Ca10(PO4)6(OH)2, HA) is a naturally occurring mineral form of calcium apatite. Approximately 50% of bone is composed of HA or a modified form of HA.29–31 Because of the higher biocompatibility and bioactivity of HA, various HA-incorporated SMSs have been produced. Normally, there are three different methods to introduce HA into polymer-based SMSs. The first and simplest method is to directly mix PLGA in an organic solvent with HA particles, followed by stirring for several hours until the HA particles are uniformly distributed in the PLGA solution.32,33 The PLGA/HA composite solution is used to produce PLGA/HA SMSs (Fig. 2). This method has been widely used to generate SMSs and various tissue engineering scaffolds. Tubular PLGA/HA SMSs have also been developed using this method.22 The aim of generating tubular scaffolds is to simulate the marrow cavity of bone tissue. In contrast to PLGA SMSs, PLGA/HA SMSs require a higher sintering temperature and time due to the higher fusion temperature of the matrix materials induced by the incorporation of HA. Kofron et al. evaluated the effect of the sintering conditions on the morphology and porosity of PLGA/HA SMSs, and they found that the elastic modulus and compressive strength significantly increased with the increase of the sintering temperature and time. Although PLGA/HA SMSs sintered at 105 °C for 2 h possess poor mechanical properties, these scaffolds have an adaptable pore structure (100 μm to 300 μm), which allows bone and vessel ingrowths. Lv et al. evaluated the osteogenesis of PLGA/HA SMSs in rotating bioreactors. The PLGA/HA (20% w/w) SMS sintered for 3 h at 90 °C was chosen as an ideal scaffold for bone repair owing to its excellent mechanical properties and moderate pore structure. Furthermore, human mesenchymal stem cells (HMSCs) were planted onto the scaffold. The HMSCs on PLGA/HA SMSs showed increased proliferation and osteogenic commitment compared with the cells on PLGA SMSs.
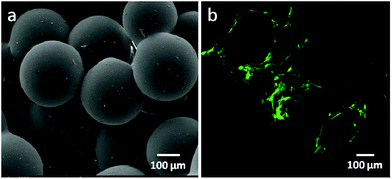 |
| Fig. 2 PLGA/HA sintered microsphere scaffolds. (a) Scanning electron microscopy of PLGA/HA sintered microsphere scaffolds; (b) osteoblasts proliferated on the PLGA/HA sintered microsphere scaffold. | |
The second route to incorporating HA or apatite into bulk PLGA is biomineralization. In vivo, the biomineralization of apatite in bone is a dynamic and well-orchestrated process controlled by bone-related cells and proteins.34,35In vitro, Kokubo et al.36 developed a simulated body fluid (SBF) with a similar ion concentration to blood plasma. SBF has been widely used for generating apatite on various surfaces of biomaterials including bioceramics, biopolymers and biometals. Jabbarzadeh et al.37,38 deposited apatite onto the surface of PLGA SMSs using SBF with a 10-fold concentration of calcium and phosphate ions. Using this method, apatite can be applied to all the inner or outer surfaces of PLGA SMSs, which benefits the initial cell adhesion onto the scaffold. Additionally, the authors confirmed that the mechanical properties of the mineralized PLGA SMSs were not significantly different from those of PLGA SMSs. The generated apatite-coated PLGA SMSs not only exhibited superior characteristics of protein adsorption and delayed release, but they also exhibited excellent cell adhesion and proliferation when compared with PLGA SMSs. An ideal bone tissue engineering scaffold can induce and support osteogenesis and also induce vascular infiltration to supply nutrients for bone remolding. Therefore, Jabbarzadeh et al. also loaded vascular endothelial growth factor (VEGF) onto the apatite layer of the mineralized PLGA SMSs. They found increased proliferation of endothelial cells on the VEGF-laden SMSs, indicating the combined effects of the mineralized SMSs on both osteogenesis and angiogenesis.
The third route to incorporate HA or apatite into PLGA is a self-assembly method.39 It is well-known that apatite or HA is easily synthesized with various calcium and phosphate-containing chemicals under different conditions. Cushnie et al. developed apatite-PLGA SMSs using a self-assembly method. They used ammonium hydrogen phosphate and calcium nitrate tetrahydrate as calcium and phosphate sources, assembled them into apatite during the emulsifying process to generate PLGA microspheres, and then used the as-prepared PLGA/HA microspheres to generate SMSs. The SMSs produced by this method showed weaker mechanical properties due to the introduction of a water phase into the PLGA microspheres, and the formed PLGA/HA microspheres have a close pore structure. Additionally, the close pores in the PLGA/HA microspheres will seriously influence the porosity and pore size of the PLGA/HA SMSs due to deformation of the microspheres during the sintering process. To improve the mechanical properties, the volume of the water phase for apatite formation should be decreased. The advantage of this method is that the generated PLGA/HA SMSs possess better degradability than the PLGA/HA SMSs produced by the first and second routes. In addition, during the process of apatite assembly, functional molecules, such as growth factors and osteogenic factors, can be loaded into the bulk PLGA to form drug release SMSs.
The three methods for generating PLGA/HA (or apatite) SMSs have their merits and demerits. The scaffolds prepared using the first method will have excellent mechanical properties, but with a plain surface composed mostly of PLGA. The SMSs generated by the second method will have a surface composed of apatite that promotes protein adsorption and cell attachment. However, after a couple of weeks of in vivo or in vitro culture, the apatite-covered surface will degrade, and then the bioactivity of the scaffolds will significantly decrease. Along with the bioactivity decrease, the mechanical stability of the apatite coating on polymer microspheres might be another major concern. The third method can form an SMS with a good controlled release function, but with the weakest mechanical properties. By considering the intended use of the SMS, we can choose an appropriate method to generate PLGA/HA (or apatite) SMSs for bone repair.
PLGA/bioactive glass sintered microsphere three-dimensional scaffolds for bone tissue engineering
Bioactive glasses are a group of inorganic biomaterials developed by Larry Hench and his colleagues in the late 1960s.40 The mineral phase generated on the surface of the bioactive glasses has a similar composition to vertebrate bone, which makes them an appropriate biomaterial for bone repair.40 However, the stumbling block for in vivo applications of bioactive glass as an implant is the formation of an alkaline microenvironment around bioactive glass blocks or scaffolds when transplanted into bone defects, which may induce a serious inflammatory response. To overcome this obstacle, bioactive glasses may be blended with PLGA. The degradation products are lactic acid and glycolic acid. Under normal physiological conditions, the by-products create an acidic environment in vivo, which can neutralize the alkaline ions produced by the bioactive glass. Yao et al.41 developed PLGA/bioactive glass SMSs. They found that after the PLGA/bioactive glass SMS was immersed in SBF for two weeks, a calcium phosphate layer was formed. The formed layer is beneficial for binding to bone. Additionally, the composite SMS with 30% bioactive glass significantly promoted the osteogenic commitment of marrow stromal cells nurtured on it. Lu et al. confirmed that the PLGA/bioactive glass SMSs not only support cell attachment, growth and differentiation42 but also possess superior mechanical properties to PLGA SMSs.
PLGA/chitosan sintered microsphere three-dimensional scaffolds for bone tissue engineering
Chitosan is a glycosaminoglycan derived from chitin.43 Chitosan has good biocompatibility and is easily chemically modified by introducing various proteins or peptides for specific purposes. Jiang et al.44,45 developed a PLGA/chitosan composite SMS. Although the chitosan/PLGA scaffold did not promote cell proliferation, the osteogenic markers such as alkaline phosphatase osteopontin produced by the cells nurtured on the PLGA/chitosan SMS significantly increased compared with those produced by the cells on pure PLGA SMSs.
PLGA/mesoporous silica sintered microsphere three-dimensional scaffolds for bone tissue engineering
Mesoporous silica materials possess a more porous structure and vast surface areas, making them popular materials for controlled release.46,47 A new material incorporating hydroxyapatite into mesoporous silica has been developed;48 this material not only maintains the porosity of mesoporous silica but also shows higher bioactivity induced by the hydroxyapatite. Shi et al. introduced this material into PLGA SMSs, and the generated PLGA/mesoporous silica-hydroxyapatite SMSs exhibited superior drug release and cell proliferation.49
Drug sintered microsphere three-dimensional scaffolds for bone tissue engineering
PLGA SMSs not only support the cell residence but also can release various molecules from the PLGA microsphere. Various drugs, such as alendronate, dexamethasone (Dex), alendronate/Dex, and Dex/β-glycerophosphate/ascorbic acid, as well as growth factors, were loaded into the PLGA-based SMSs for bone/cartilage regeneration applications.50 Dex, β-glycerophosphate and ascorbic acid are important additives in traditional osteogenic media. For the osteogenic differentiation of stem cells on a three-dimensional scaffold, the osteogenic media should be changed every three days. However, when implanting a stem cell-laden scaffold into a bone defect induced by a tumor or other bone-related diseases, it is difficult to differentiate stem cells into specialized cells in a damaged microenvironment. Therefore, loading osteogenic differentiation factors such as Dex, β-glycerophosphate and ascorbic acid into the scaffolds so that the scaffold releases the drugs during the process of bone reconstruction is ideal. Shi et al. loaded Dex, β-glycerophosphate and ascorbic acid into PLGA SMSs (Fig. 3). HMSCs were nurtured on the drug-loaded SMS. The results indicated that the released cell differentiation factors significantly promoted the osteogenic commitment of the HMSCs. Alendronate, a nitrogenous bisphosphonate, is known to promote the activity of osteoblasts and to induce the osteogenic differentiation of stem cells. However, due to its superhydrophilicity, loading it directly into a PLGA matrix is very ineffective. Due to the strong binding of alendronate with hydroxyapatite, alendronate-laden PLGA/HA and PLGA/mesoporous-HA SMSs have been developed.51 Both of these scaffolds showed a significantly enhanced alendronate loading rate and a longer drug release period compared with pure PLGA SMSs. Additionally, when culturing stem cells onto these scaffolds, the stem cells were easily into osteoblastic cells in situ due to the sustained release of alendronate. Compared with stem cells on PLGA/HA SMSs, stem cells on the PLGA/mesoporous silica-HA SMSs exhibited stronger osteogenic differentiation, indicating a superior controlled release of alendronate from the PLGA/mesoporous silica-HA SMSs. Growth factors such as transforming growth factor beta I and insulin-like growth factor I have been encapsulated into PLGA SMSs that were produced by solvent sintering instead of heat sintering due to the higher sensitivity of these growth factors to temperature. The controlled release of growth factors from SMSs also facilitates tissue regeneration.
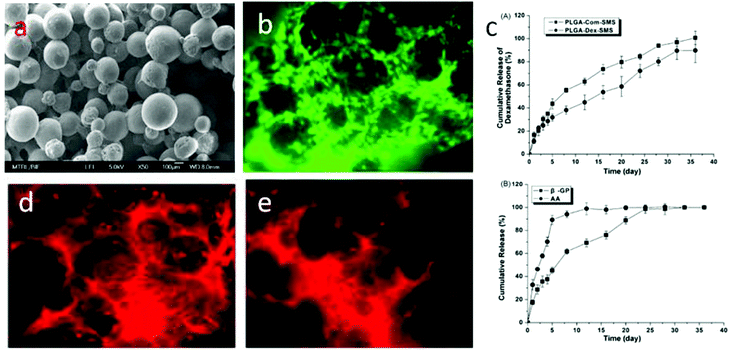 |
| Fig. 3 Dex/β-glycerophosphate/ascorbic acid laden PLGA sintered microsphere scaffolds for inducing osteogenesis of stem cells. (a) Scanning electron microscopy of Dex/β-glycerophosphate/ascorbic acid laden PLGA sintered microsphere scaffolds. (b) Proliferation of human bone marrow mesenchymal stem cells (hMSCs) on Dex/β-glycerophosphate/ascorbic acid laden PLGA sintered microsphere scaffolds. (c) Cumulative release of Dex, β-glycerophosphate and ascorbic acid from the drug-laden PLGA sintered microsphere scaffold. (d) and (e) Immunofluorescence staining of type I collagen and osteocalcin secreted by hMSCs cultured on Dex/β-glycerophosphate/ascorbic acid laden PLGA sintered microsphere scaffolds. | |
Human bone tissue is an exquisite assembly of cells and extracellular matrix. The proliferation, differentiation, and arrangement are regulated in vivo.52 To simulate the morphology of periosteum, which covers the surface of bone and plays an important role in osteogenesis, Shi et al. developed free-standing grooved micropatterns for bone repair (Fig. 4). The grooved micropatterns were generated by sintering PLGA microspheres using heat or a solvent. They used a Teflon mold to produce the micropatterns instead of the traditional polydimethylsiloxane (PDMS) because of the weak resistance of PDMS to organic solvents. The developed grooved micropatterns can not only encapsulate both hydrophilic (such as growth factors) and hydrophobic (such as Dex) osteogenic factors but can also control cell spatial arrangement. Adipose-derived stem cells were cultured onto the micropatterns, and the combination of spatial (grooved pattern) and biochemical (the release of osteogenic factors) cues significantly promoted the osteogenesis of stem cells on the micropattern, indicating the potential application of these free-standing micropatterns in bone repair.
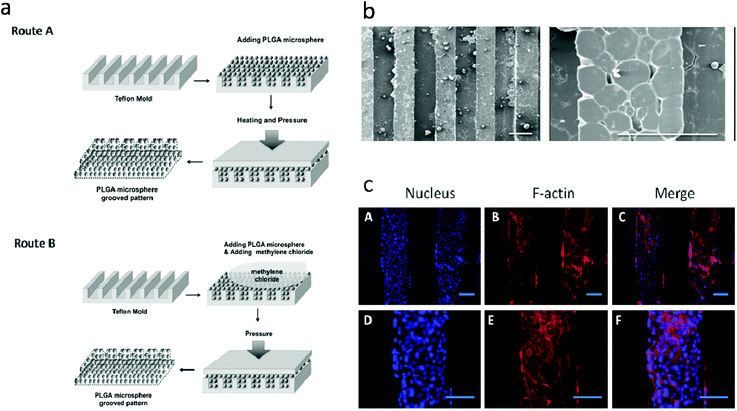 |
| Fig. 4 Generation of polymer-microsphere-based micropatterns. (a) Schematic of generation of polymer-microsphere-based micropatterns; (b) scanning electron microscopy of polymer-microsphere-based micropatterns; (c) nuclear and F-actin staining of adipose-derived stem cells on osteogenic factor laden PMGMs with different groove spacings after 14 days of culture. Scale bars are 200 μm. Reproduced from ref. 52 with permission. | |
Microsphere-containing scaffolds for bone tissue engineering
Another kind of microsphere scaffolds is one which is composed of one or more matrices and decorated or incorporated with microspheres, especially drug containing microspheres. Polymer or inorganic microspheres also can be integrated into scaffolds composed of organic materials, such as chitosan, collagen, PLGA, etc.
Bone is a complex system composed of 70% inorganic hydroxyapatite (HA) and 30% organic proteins (such as collagen).53 A number of studies have focused on the bone repair applications of inorganic materials. Due to their excellent properties for drug encapsulation and controlled release, inorganic microspheres have drawn great attention. HA is a major constituent of bone and possesses excellent biocompatibility compared with most inorganic materials. In addition to its biocompatibility, HA possesses another unique characteristic in that it can absorb chemicals or biomolecules such as drugs, DNA, or proteins on its surface.54–56 HA can be used as a drug release vehicle for delayed drug release and the promotion of bone repair. Various chemical species have been loaded onto HA microspheres. HA microspheres can be generated by different methods including water/oil double emulsion, spray drying, template synthesis, and so on.57–59 Similar to the oil/water double emulsion for producing polymer microspheres, the water/oil double emulsion for preparing HA microspheres uses calcium and phosphate sources as the water phase and stirs the water phase into the oil phase during the solidification process of HA. Pradeesh et al.60 combined oil/water emulsion and solvent evaporation techniques to produce HA microspheres. They used ethylene vinyl acetate (EVA) as a binder material. After binding HA particles, the HA/EVA composite microspheres were sintered at 1150 °C to remove the EVA to obtain HA microspheres. Wang et al. used spray drying to produce HA microspheres of different sizes. The advantage of this method is that the particle size can be well controlled in a single step. Oliveira et al.61 developed a method for preparing large HA microspheres (more than 100 μm). They mixed HA particles with a Na-alginate solution under stirring and then injected the HA-alginate paste into a calcium-containing cross-linking bath. The size of the microspheres was controlled by the extrusion flow rate using a syringe pump. After that, the achieved microspherical gels were sintered at a high temperature to generate HA microspheres. Wang et al.62 used polyelectrolytes to regulate the formation of HA microspheres. These types of microspheres can be used as injectable scaffolds for bone regeneration applications. Poly(styrene sulfonate) (PSS) was added at different concentrations in calcium and phosphate sources, and then the produced paste was heated at different temperatures. At increased concentrations of PSS, the morphologies of the HA particles change from nanofibers to nanorods and then to microspheres. When the concentration of PSS reaches 9.6%, microspheres with good morphology can be achieved. Compared with solid HA microspheres, hollow HA microspheres have a larger surface area, which may significantly enhance drug adsorption. Generally, the template method is used to produce hollow HA microspheres. Using borate glass as a template is one popular method.63 By incubating borate glass microspheres in a dilute phosphate solution at room temperature, the borate glass is converted into hollow HA microspheres.
Tan et al.64,65 integrated PLGA microspheres into gelatin/chitosan/hyaluronan scaffolds fabricated by freeze-drying. The scaffolds with a high content of PLGA microspheres (up to 50%) have a higher density and compressive modulus but a lower porosity and weight loss. PLGA has also been integrated into fibrin hydrogels. When compared with pure fibrin hydrogels, the composite PLGA microsphere/fibrin hydrogel has a significantly increased elastic modulus. However, the cell proliferation and extracellular matrix production of the composite is comparable to those of the pure fibrin hydrogel. Kempen et al.66 incorporated BMP-2-laden PLGA microspheres into gelatin, poly(propylene fumarate) (PPF), and gelatin and PPF. The osteoblast-laden composite scaffolds were investigated in vivo for 12 weeks in a rat subcutaneous implantation model. The results indicated that the gelatin/microsphere composite showed a weaker maintenance of the BMP-2 activity when compared with other composite scaffolds. After 12 weeks of culture in vivo, the new bone formation in the microsphere/PPF scaffolds remained remarkably higher than in the other scaffolds. The aforementioned composite materials blend synthetic organosoluble microspheres into natural, water-soluble scaffolds. Huang et al. developed a scaffold which is produced by blending organosoluble microspheres into an organic-solvent–solvent matrix. Poly(β-hydroxybutyrate-co-β-hydroxyvalerate) (PHBV) microspheres were thus blended into a PLGA scaffold. In contrast to PLGA, PHBV cannot be dissolved into acetone.67,68 Therefore, blending PHBV microspheres into a PLGA/acetone solution is feasible. Integrating 30% PHBV microspheres into a PLGA matrix not only significantly increases the compressive strength of the scaffold but also has a smaller effect on the cell behavior and the porosity. Additionally, PHBV microspheres can also encapsulate and release drugs for bone regeneration.
Polymer microspheres can also be used as injectable scaffolds. Conventionally, polymer microspheres are used for encapsulating various molecules such as growth factors, proteins, and cell differentiation chemicals to enhance or promote bone repair. For example, BMP-2 loaded PLGA microspheres have been used for bone repair. After injecting BMP-2-laden PLGA microspheres into bone defects, significant new bone formation was observed. Na et al.69,70 developed a nanostructured microsphere scaffold for tissue engineering and drug delivery applications. They coated heparin/poly(L-lysine) nanoparticles onto polyethyleneimine-treated PLGA microspheres via a layer-by-layer method. Hydrophilic (such as growth factors) and hydrophobic (such as Dex) chemicals or proteins can be loaded into the heparin nanoparticles and PLGA microspheres, respectively. Park et al. used this system to investigate cartilage repair. They loaded Dex and transforming growth factor-β3 (TGF-β3) into the double microsphere system. The dual release of TGF-β3 and Dex can be used to promote cartilage regeneration.
Due to the high bioactivity and charged properties of inorganic materials such as HA, developing inorganic/organic composite microspheres would be an ideal choice for both drug delivery and bone repair. Different methods have been used to fabricate PLGA/HA microspheres. Xu et al.71 developed negatively charged PLGA microspheres by an oil/water emulsion method using an anionic surfactant. An HA coating was applied to the charged PLGA microspheres by a dual constant composition method. Shen et al.72 developed PLGA/HA microspheres by directly blending HA particles with PLGA solution and then forming microspheres by emulsion. To expose the HA particles on the surface of microspheres, they treated the composite microspheres with aqueous NaOH and ethanol. The resulting microspheres exhibited excellent properties for loading BMP-2, and the BMP-2-loaded microspheres significantly promoted cell growth and osteoblastic differentiation of osteoblast-like cells. An apatite coating on the surface of the PLGA microspheres can also be formed by incubating PLGA microspheres in SBF solution. The resulting composite microspheres can enhance osteoblast attachment and protein loading.
To sum up, microspheres can not only be used to form a three-dimensional scaffold but also be incorporated into scaffold matrices for bone regeneration. Although lots of research has been done in this field, it is far from achieving a perfect system which can substitute bone tissue itself entirely. Scaffolds based on microspheres or functional microspheres as molecular carriers could be promising portfolios for bone tissue engineering.
Acknowledgements
This work was supported by 973 project (2012CB933600) and Shenzhen Biological, Internet, new energy industry development special fund (CXB201104250055A).
References
- F. F. Parrish, Clin. Orthop. Relat. Res., 1982, 87, 36 Search PubMed.
- H. Petite, V. Viateau, W. Bensaid, A. Meunier, C. de Pollak, M. Bourguignon, K. Oudina, L. Sedel and G. Guillemin, Nat. Biotech., 2000, 18, 959 CrossRef CAS PubMed.
- R. C. Thomson, M. J. Yaszemski, J. M. Powers and A. G. Mikos, Biomaterials, 1998, 19, 1935 CrossRef CAS.
- J. J. Drury and D. J. Mooney, Biomaterials, 2002, 24, 4337 CrossRef.
- Z. Ma, M. Kotaki, R. Inai and S. Ramakrishna, Tissue Eng., 2005, 11, 101 CrossRef CAS PubMed.
- D. W. Hutmacher, Biomaterials, 2000, 21, 2529 CrossRef CAS.
- S. J. Hollister, Nat. Mater., 2005, 4, 518 CrossRef CAS PubMed.
- L. E. Freed, G. Vunjak-Novakovic, R. J. Biron, D. B. Eagles, D. C. Lesnoy, S. K. Barlow and B. Langer, Nat. Biotech., 1994, 12, 689 CrossRef CAS PubMed.
- M. Borden, S. F. EI-Amin, M. Attawia and C. T. Laurencin, Biomaterials, 2003, 24, 597 CrossRef CAS.
- X. T. Shi, K. Su, R. R. Varshney, Y. Wang and D.-A. Wang, Pharm. Res., 2011, 28, 1224 CrossRef CAS PubMed.
- M. Borden, M. Attawia, Y. Khan and C. T. Laurencin, Biomaterials, 2002, 23, 551 CrossRef CAS.
- J. L. Brown, L. S. Nair and C. T. Laurencin, J. Biomed. Mater. Res., Part B, 2008, 86, 396 CrossRef PubMed.
- X. T. Shi, Y. J. Wang, L. Ren, C. Lai, Y. H. Gong and D. A. Wang, J. Biomed. Mater. Res., Part A, 2010, 92, 963 Search PubMed.
- J. M. Anderson and M. S. Shive, Adv. Drug Delivery Rev., 1997, 28, 5 CrossRef CAS.
- S. Ando, D. Putnam, D. W. Pack and R. Langer, J. Pharm. Sci., 1999, 88, 126 CrossRef CAS PubMed.
- P. Johansen, Y. Men, H. P. Merkle and B. Gander, Eur. J. Pharm. Biopharm., 2000, 50, 129 CrossRef CAS.
- A. Luciani, V. Coccoli, S. Orsi, L. Ambrosio and P. A. Netti, Biomaterials, 2008, 29, 4800 CrossRef CAS PubMed.
- P. B. Malafaya, A. J. Pedro, A. Peterbauer, C. Gabriel, H. Redl and R. L. Reis, J. Mater. Sci. Mater. Med., 2005, 16, 1077 CrossRef CAS PubMed.
- P. B. Malafaya and R. L. Resi, Acta Biomater., 2009, 5, 644 CrossRef CAS PubMed.
- P. B. Malafaya, T. C. Santos, M. V. Griensven and R. L. Resi, Biomaterials, 2008, 29, 3914 CrossRef CAS PubMed.
- W. I. Abdel-Fattah, T. Jiang, G. E. EI-Bassyouni and C. T. Laurencin, Acta Biomater., 2007, 3, 503 CrossRef CAS PubMed.
- M. D. Kofron, J. A. Cooper Jr., S. G. Kumbar and C. T. Laurencin, J. Biomed. Mater. Res., Part A, 2007, 82, 415 CrossRef CAS PubMed.
- M. D. Kofron, A. Griswold, S. G. Kumbar, K. Martin, X. Wen and C. T. Laurencin, Adv. Funct. Mater., 2009, 19, 1351 CrossRef CAS.
-
M. Muller, J. Voros, G. Csucs, E. Walter, G. Danuser, H. P. Merkle, N. D. Spencer and M. Textor, J. Biomed. Mater. Res., Part A, 2003, 66A, 55 Search PubMed.
- T. M. Fahmy, R. M. Samstein, C. C. Harness and W. M. Saltzman, Biomaterials, 2005, 26, 5727 CrossRef CAS PubMed.
- P. Wan, Y. Zhao, H. Tong, Z. Zhu, X. Shen and J. Hu, Mater. Sci. Eng., C, 2009, 29, 222 CrossRef CAS PubMed.
- N. Zhu, F. Z. Cui, K. Hu and L. Zhu, J. Biomed. Mater. Res., Part A, 2007, 82, 455 CrossRef CAS PubMed.
- X. T. Shi, Y. J. Wang, L. Ren, W. Huang and D. A. Wang, Int. J. Pharm., 2009, 373, 85 CrossRef CAS PubMed.
- G. L. De Lange, C. De Putter and F. L. J. A. De Wijs, J. Biomed. Mater. Res., Part A, 1990, 24, 829 CrossRef CAS PubMed.
- D. M. Roy and S. K. Linnenan, Nature, 1997, 247, 220 CrossRef.
- M. I. Kay, R. A. Young and A. S. Posner, Nature, 1964, 204, 1050 CrossRef CAS.
- X. T. Shi, Y. J. Wang, L. Ren, Y. H. Gong and D. A. Wang, Pharm. Res., 2009, 26, 422 CrossRef CAS PubMed.
- Q. Lv, L. Nair and C. T. Laurencin, J. Biomed. Mater. Res., Part A, 2009, 91, 679 CrossRef PubMed.
- S. Weiner, W. Traub and H. D. Wagner, J. Struct. Biol., 1999, 126, 241 CrossRef CAS PubMed.
- T. Kokubo, Mater. Sci. Eng., C, 2005, 25, 97 CrossRef PubMed.
- T. Kokubo and H. Takadama, Biomaterials, 2006, 27, 2907 CrossRef CAS PubMed.
- E. Jabbarzadeh, L. S. Nair, Y. M. Khan, M. Deng and C. T. Laurencin, J. Biomater. Sci., Polym. Ed., 2007, 18, 1141 CrossRef CAS PubMed.
- E. Jabbarzadeh, M. Deng, Q. Lv, T. Jiang, Y. M. Khan, L. S. Nair and C. T. Laurencin, J. Biomed. Mater. Res., Part B, 2012, 100, 2187 CrossRef PubMed.
- E. K. Cushnie, Y. M. Khan and C. T. Laurencin, J. Biomed. Mater. Res., Part A, 2008, 84, 54 CrossRef PubMed.
- L. L. Hench and H. A. Paschall, J. Biomed. Mater. Res., 1973, 7, 25 CrossRef CAS PubMed.
- J. Yao, S. Radin, P. S. Leboy and P. Ducheyne, Biomaterials, 2005, 26, 1935 CrossRef CAS PubMed.
- H. H. Lu, S. F. EI-Amin, K. D. Scott and C. T. Laurencin, J. Biomed. Mater. Res., Part A, 2003, 64, 465 Search PubMed.
- M. N. V. R. Kumar, React. Funct. Polym., 2000, 46, 1 CrossRef CAS.
- T. Jiang, W. I. Abdel-Fattah and C. T. Laurencin, Biomaterials, 2006, 27, 4894 CrossRef CAS PubMed.
- T. Jang, Y. Khan, L. S. Nair, W. I. Adbel-Fattah and C. T. Laurencin, J. Biomed. Mater. Res., Part A, 2010, 93, 1193 Search PubMed.
- H. Yang, N. Coombs and G. A. Ozin, Nautre, 1997, 386, 692 CrossRef CAS.
- I. I. Slowing, B. G. Trewyn, S. Giri and V. S. Y. Lin, Adv. Funct. Mater., 2007, 17, 1225 CrossRef CAS.
- X. T. Shi, Y. J. Wang, K. Wei, L. Ren and C. Lai, J. Mater. Sci. Mater. Med., 2008, 19, 2933 CrossRef CAS PubMed.
- X. T. Shi, Y. J. Wang, L. Ren, N. R. Zhao, Y. H. Gong and D. A. Wang, Acta Biomater., 2009, 5, 1697 CrossRef CAS PubMed.
- X. T. Shi, Y. J. Wang, R. R. Varshney, L. Ren, Y. H. Gong and D. A. Wang, Eur. J. Pharm. Sci., 2010, 39, 59 CrossRef CAS PubMed.
- X. T. Shi, Y. J. Wang, R. R. Varshney, L. Ren, F. Zhang and D. A. Wang, Biomaterials, 2009, 30, 3996 CrossRef CAS PubMed.
- X. T. Shi, S. Chen, J. H. Zhou, H. J. Yu, L. Li and H. K. Wu, Adv. Funct. Mater., 2012, 22, 3799 CrossRef CAS.
- R. Muller, H. Van Campenhout, B. Van Damme, G. Van Der Perre, J. Dequeker, T. Hildebrand and P. Ruegsegger, Bone, 1998, 23, 59 CrossRef CAS.
- Q. Wang, W. Huang, D. Wang, B. W. Darvell, D. E. Day and M. N. Rahaman, J. Mater. Sci. Mater. Med., 2006, 17, 641 CrossRef CAS PubMed.
- Y. Boonsongrit, H. Abe, K. Sato, M. Naito, M. Yoshimura, H. Ichikawa and Y. Fukumori, Mater. Sci. Eng., B, 2008, 148, 1 CrossRef PubMed.
- M. Sivakumar and K. P. Rao, Biomaterials, 2002, 23, 3175 CrossRef CAS.
- A. J. Wang, Y. P. Lu, R. F. Zhu, S. T. Li and X. L. Ma, Powder Technol., 2009, 191, 1 CrossRef CAS PubMed.
- W. Paul and C. P. Sharma, J. Colloid Interface Sci., 1995, 174, 224 CrossRef CAS.
- R. Sun, Y. Lu and K. Chen, Mater. Sci. Eng., C, 2009, 29, 1088 CrossRef CAS PubMed.
- T. S. Pradeesh, M. C. Sunny, H. K. Varma and P. Ramesh, Bull. Mater. Sci., 2005, 28, 383 CrossRef CAS.
- S. M. Oliveira, C. C. Barrias, I. F. Almeida, P. C. Costa, M. R. P. Ferreira, M. F. Bahia and M. A. Barbosa, J. Biomed. Mater. Res., Part B, 2008, 87, 49 CrossRef PubMed.
- Y. Wang, M. S. Hassan, P. Gunawan, R. Lau, X. Wang and R. Xu, J. Colloid Interface Sci., 2009, 339, 69 CrossRef CAS PubMed.
- W. Huang, M. N. Rahaman, D. E. Day and B. A. Miller, J. Mater. Sci. Mater. Med., 2009, 20, 123 CrossRef CAS PubMed.
- H. Tan, J. Wu, L. Lao and C. Gao, Acta Biomater., 2009, 5, 328 CrossRef CAS PubMed.
- X. Hu, J. Zhou, N. Zhang, H. Tan and C. Gao, J. Mech. Behav. Biomed. Mater., 2008, 1, 352 CrossRef PubMed.
- D. H. Kempen, L. Lu, A. Heijink, T. E. Hefferan, L. B. Creemers, A. Maran, M. J. Yaszemski and W. J. Dhert, Biomaterials, 2009, 30, 2816 CrossRef CAS PubMed.
- D. H. R. Kempen, L. Lu, A. Heijink, T. E. Hefferan, L. B. Creemers, A. Maran, M. J. Yaszemski and W. J. A. Dhert, Biomaterials, 2009, 30, 2816 CrossRef CAS PubMed.
- D. H. R. Kempen, L. Lu, T. E. Hefferan, L. B. Creemers, A. Maran, K. L. Classic, W. J. A. Dhert and M. J. Yaszemski, Biomaterials, 2008, 29, 3245 CrossRef CAS PubMed.
- K. Na, S. Kim, K. Park, K. Kim, D. G. Woo, I. C. Kwon, H. M. Chung and K. H. Park, J. Am. Chem. Soc., 2007, 129, 5788 CrossRef CAS PubMed.
- J. S. Park, K. Na, D. G. Woo, H. N. Yang and K. H. Park, Biomaterials, 2009, 30, 4796 CrossRef CAS PubMed.
- Q. Xu and J. T. Czernuszka, J. Controlled Release, 2008, 127, 146 CrossRef CAS PubMed.
- H. Shen, X. Hu, F. Yang, J. Bei and S. Wang, Acta Biomater., 2010, 6, 455 CrossRef CAS PubMed.
|
This journal is © The Royal Society of Chemistry 2014 |
Click here to see how this site uses Cookies. View our privacy policy here.