DOI:
10.1039/C4BM00203B
(Paper)
Biomater. Sci., 2014,
2, 1761-1767
HPMC/PAA hybrid nanogels via aqueous-phase synthesis for controlled delivery of insulin
Received
6th June 2014
, Accepted 21st July 2014
First published on 6th August 2014
Abstract
A facile controlled-release delivery system has been developed with hydroxypropylmethylcellulose/poly(acrylic acid) (HPMC/PAA) hybrid nanogels by surfactant-free polymerization in aqueous solution. The effect of temperature, reaction time, and amount of cross-linker on the size and morphology of the nanogels has been studied. The results showed that when the reaction temperature is higher than the lower critical solution temperature, the particle size increases with increasing temperature. And in a certain range, the particle size decreases with increasing reaction time and amount of cross-linker, the prepared nanogel under each condition being spherical. Thus, the HPMC/PAA hybrid nanogels were prepared at 41 °C for 4 h, and possessed a size ranging from 250 nm to 615 nm. Then, insulin was loaded into the nanogel, and the pH and temperature sensitivity of the nanogel was studied, the results indicating that the release behavior of the nanogel can be regulated by pH and temperature. Finally, in streptozotocin-induced diabetic mice, insulin-loaded HPMC/PAA complex was able to maintain blood glucose concentration at a low level for the first week compared to a control group. Therefore, the nanogels have potential biological application as a long-term, sustained insulin delivery system.
1. Introduction
Nanogels are three-dimensional nano-sized polymer networks with good biocompatibility. These structures can not only serve as skeletal material to protect a loaded drug but also rapidly respond to micro-environmental stimuli such as temperature and pH, because of their small size, which can achieve the controlled release of the loaded drug. In recent years, nanogels with multiple responsiveness have become a focus of research in the fields of controlled-release drug delivery, bio-engineering and so on. Composite nanogels consisting of poly(acrylic acid) (PAA) and hydroxypropylmethylcellulose (HPMC) have simultaneous pH and temperature sensitivity, similar to PAA/hydroxypropylcellulose, PAA/poly(N-isopropyl acrylamide), poly(maleic acylating glucan/N-isopropyl acrylamide), etc.1–4 The two most common methods reported for fabricating nanogels are emulsion radical polymerization and seed emulsion polymerization. The usage of some organic solvents or surfactants during these preparation processes may limit a product's clinical application as a drug delivery system.
In the past few decades, the number of diabetic patients has rapidly increased. Most diabetic patients will ultimately receive insulin therapies, and receive insulin injections at least once a day, which entail patient troubles and pains. A controlled and prolonged release system is needed to reduce the injection frequency, and thus increase patient compliance.5–9 Chitosan and poly(γ-glutamic acid) conjugated with ethylene glycol tetraacetic acid has been used for oral insulin delivery. Poly(lactic acid)-b-Pluronic-b-poly(lactic acid)-based nanogels could be promising systems for oral delivery of insulin due to their prolonged hypoglycemic effect. Biodegradable nanogels loaded with insulin-phospholipid complex were prepared by a novel reverse micelle-solvent evaporation method, which could enhance oral bioavailability. The pH-responsive hydrogels prepared with poly[(methacrylic acid)-g-(ethylene glycol)] copolymers have shown potential as oral insulin delivery systems. Moreover, an insulin-loaded chitosan nanogel following intratracheal administration to rats induced a more pronounced and prolonged hypoglycemic effect. Methyl-β-cyclodextrin-complexed insulin was encapsulated onto poly(methacrylic acid)-chitosan-poly(ethylene glycol) microparticles by a diffusion filling method that could be effective in reducing blood glucose level in diabetic animal models. N-trimethylchitosan microparticles could be used as a promising vehicle for pulmonary delivery of insulin.10–16
Herein, we developed an aqueous-phase synthesis of HPMC/PAA hybrid nanogels without usage of organic solvents and surfactants. The obtained nanogel could efficiently encapsulate insulin. We show clear evidence that such an insulin loaded nanogel is capable of maintaining blood glucose concentration at a low level and can be an excellent long-term insulin delivery system.
2. Materials and methods
2.1 Materials
HPMC powder (average Mw = 1.0 × 105), acrylic acid (AA, 99%), N,N′-methylenebisacrylamide (MBA, 99%), N,N,N′,N′-tetramethylethylenediamine (TEMED), and potassium persulfate (KPS, 99%) were purchased from Tianjin Bodi Chemical Ltd and used without further purification. Insulin (INS, >99%) was obtained from Jinqiao IMC Pharmaceutical Co. Ltd (Xuzhou, China). Streptozotocin was purchased from Sigma (St. Louis, USA). Deionized water was used throughout.
2.2 Animals
Healthy 4–6-week-old male C57BL/6 mice (25–30 g) were purchased from Vital River Laboratory Animal Technology Co. Ltd. All animals received care in compliance with the guidelines outlined in the Guide for the Care and Use of Laboratory Animals and the procedures were approved by the University of Science and Technology of China Animal Care and Use Committee.
2.3 Synthesis of the HPMC/PAA nanogel
HPMC powder was dispersed in deionized water at a concentration of 0.5 wt% at 60–70 °C. After cooling to room temperature, 50 mL of the HPMC solution was added to a round-bottom flask with a certain amount of AA (AA/HPMC = 7, mass ratio) and appropriate amount of MBA (MBA/AA = 0.05, mass ratio). After the mixture was bubbled with N2 for 30 min, the polymerization of AA was initiated using KPS/TEMED as initiator (KPS having the same amount as MBA and TEMED/AA = 0.005, mass ratio). The polymerization was allowed to continue for 4–5 h at the lower critical solution temperature (LCST) of HPMC solution mixing with AA under N2 atmosphere.17,18
2.4 Insulin loading
Insulin-loaded nanogel can be prepared by two methods. The first method is adsorption of insulin into the obtained nanogel. Briefly, an appropriate amount of insulin (40 mg mL−1) was dissolved in 0.1 mol L−1 HCl solution, and then the solution was added dropwise to the prepared nanogel solution with constant stirring. After stirring at room temperature for 24 h, the solution was transferred into dialysis tubing (Spectra/Por, Float-A-Lyzer, with a molecular weight cut-off (MWCO) of 14 kDa) and dialyzed against ultra-purified water for 2 days at room temperature, then freeze-dried to obtain solid product.
In the second method, the insulin can be loaded into the nanogel during its preparation. Briefly, HPMC powder (0.5 g) was dispersed in deionized water (100 mL) at a concentration of 0.5 wt% at 60–70 °C. After cooling to room temperature and becoming clarified, the HPMC solution (50 mL) was transferred to a round-bottom flask with a certain amount of AA (AA/HPMC = 7, mass ratio) containing an appropriate amount of insulin (40 mg mL−1) and an appropriate amount of MBA (MBA/AA = 0.05, mass ratio). Experimental conditions were the same as for the preparation of the HPMC/PAA nanogel as described above.
2.5 Characterization
The size distribution and zeta potential of the nanogel were determined by dynamic light scattering using a Malvern Instruments Zetasizer Nano series instrument (ZS90) operating at an angle of 90° by using a He–Ne laser (22 mW) with a wavelength of 633 nm. Measurements were carried out at 25 °C. Dispersion Technology Software 5.03 (Malvern Instruments) was used to record and analyze the data to determine particle size distribution. Transmission electron microscopy (TEM) images were obtained using an H-800 electron microscope (Hitachi, Tokyo, Japan). The TEM sample was prepared by placing a dilute drop of the measured solution onto a copper grid and allowing it to dry. Then the dried sample was stained with mass faction of 1% phosphotungstic acid aqueous solution and allowing it to dry at room temperature.
2.6 Measurement of entrapment efficiency
Entrapment efficiency was measured by a UV spectrophotometer (Milton Roy 601, USA). The insulin-loaded nanogel (20 mg) was dissolved in 0.1 M HCl solution (5 mL), ultrasonically continuing for 24 h, then diluted in a 10 mL volumetric flask. The absorbance of the resulting solution was measured at a wavelength of 276 nm. Insulin content was subsequently calculated according to the standard curve equation.
2.7 MTT assay
The relative cytotoxicity of micelles was assessed with a methyl tetrazolium (MTT) viability assay against HEK293 cells. Cells were seeded in 96-well plates at 10
000 cells per well in 100 μL of complete Dulbecco's modified Eagle medium (DMEM) supplemented with 10% fetal bovine serum, and incubated at 37 °C in a 5% CO2 atmosphere for 24 h. To determine the cytotoxicity of the nanogel, 100 μL of blank nanogel in complete DMEM at different concentrations were used to replace the culture medium and cells were further cultured for 48 h. MTT stock solution was added to each well to achieve a final concentration of 1 g L−1, with the exception of the wells used as a blank, to which the same volume of phosphate-buffered saline (PBS; 0.01 M, pH 7.4) was added. After incubation for an additional 2 h, 100 μL of the extraction buffer (20% SDS in 50% N,N-dimethylformamide, pH 4.7, prepared at 37 °C) was added to the wells and incubated overnight at 37 °C. The absorbance was measured at 570 nm using a Bio-Rad 680 microplate reader (Bio-Rad, USA). Cell viability was normalized to that of cells cultured in the culture medium with PBS treatment.
2.8
In vitro release studies of INS-loaded nanogel
To demonstrate pH-sensitive insulin release, the nanogel (0.2 g, 20 mL) was incubated at pH 1.2 and pH 7.4, and then transferred into dialysis membrane tubing (MWCO of 8000). The tubing was immersed in 200 mL of each buffer and shaken (150 rpm) at 37 °C. At predetermined time, the external buffer was collected and replaced by fresh buffer. The concentration of insulin was determined by a UV spectrophotometer as described above. In addition, to study the temperature-sensitive release, the insulin-loaded nanogel (0.2 g, 20 mL) was incubated at different temperatures (30.0 °C, 37.0 °C, 41.0 °C, 47.0 °C) at pH 7.4, and the release profile was analyzed as described above.
2.9
In vivo studies in mice
To establish the mouse model of diabetes, C57BL/6 mice were fasted for more than 12 h with free access to water, and then streptozotocin dissolved in 0.1 M citrate buffer (pH 4.2) was intraperitoneally injected at a dose of 50 mg kg−1 on 5 consecutive days after overnight fasting. Diabetes syndrome was confirmed by measuring the blood glucose level using a glucometer (Glucometer Elite XL; Bayer, Elkhart, IN) following collection of around 2 μL of blood from the tail vein. A blood glucose level larger than 13.9 mM L−1 was considered to represent diabetes, as shown previously.19
The diabetic mice were randomly divided into two groups (five mice for each group), and fasted for 12 h with free access to water. Then, insulin-loaded nanogel suspended in an aqueous solution containing 1% sodium carboxymethylcellulose was injected subcutaneously at the hind region of the diabetic mice at an insulin dose of 20 IU per mouse. Insulin dose was approximately 4 IU per mouse for the control group. At predetermined time points, the blood glucose levels of the mice in the experimental groups were checked after 3 h of fasting and were compared with those of the diabetic or non-diabetic control groups without insulin treatment.19
3. Results and discussion
3.1 The effect of reaction temperature on the nanogel
The nanogel was synthesized at different temperatures, and the size distribution of the nanogel was measured by dynamic light scattering (Fig. 1A and B). The sizes of resulting HPMC/PAA nanogel were distributed between 295 nm and 1718 nm at 30 °C which is less than the LCST which may lead to the crosslinking reaction being incomplete. The sizes were distributed between 220 nm and 615 nm at 41 °C which is exactly the LCST. When the temperature is higher than 41 °C, the average particle size became gradually larger. It is speculated that when the reaction temperature just reaches the LCST, the polymer chains aggregate to form a stable nanogel, while on increasing the temperature above the LCST, the hydrophobic interaction force increases, which leads to an increase of driving force of polymer aggregation. Therefore, the size of the nanogel becomes larger. At the same time, hydrophobic interaction and electrostatic repulsion within the micelles reach a new equilibrium with the electrostatic repulsion between molecules increasing. The size of the nanogel synthesized at different temperatures was also confirmed by TEM. The nanogel exhibited a compact and spherical morphology, with the size of the nanogel being 900 nm, 400 nm and 600 nm, respectively, when the polymerization was performed at 30 °C, 41 °C and 45 °C.
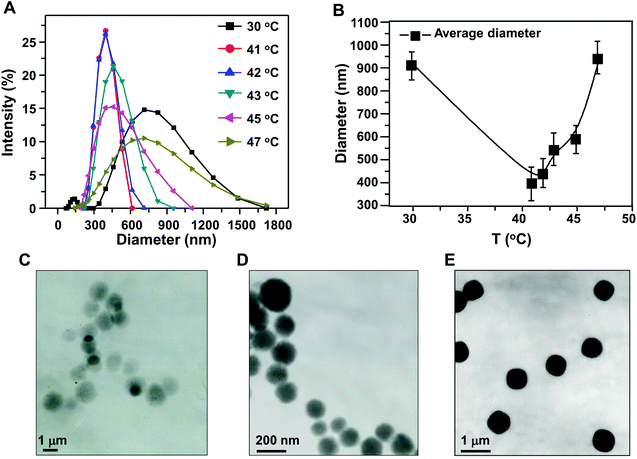 |
| Fig. 1 (A, B) Effect of reaction temperature on the particle size distribution of HPMC/PAA nanogel; TEM images of the nanogel obtained at different temperatures: (C) 30 °C; (D) 41 °C; (E) 45 °C. | |
3.2 The effect of reaction time on the nanogel
The reaction time is a very important factor to control the size of the obtained nanogel. After polymerization for different times at the LCST, the size distribution and TEM images of the nanogel were observed. As shown in Fig. 2A and B, the obtained nanogel exhibited a wide size distribution after 20 min, because AA had not yet completely polymerized and the chain was not fully cross-linked. Furthermore, the size distribution became narrower because of the formation of a semi-interpenetrating network structure between HPMC chains and PAA chains after 40 min and 60 min. As the reaction time increased, the particle size ranged from 255 nm to 615 nm after 3 h, and from 295 nm to 530 nm after 4 h. The particle size distributions were nearly identical after 4 h and 5 h, the cross-linking polymerization being complete at this time, and the particle size and size distribution did not change significantly. The size of the nanogel synthesized with different reaction times was also confirmed by TEM. The nanogel exhibited a compact and spherical morphology (Fig. 2C–E).
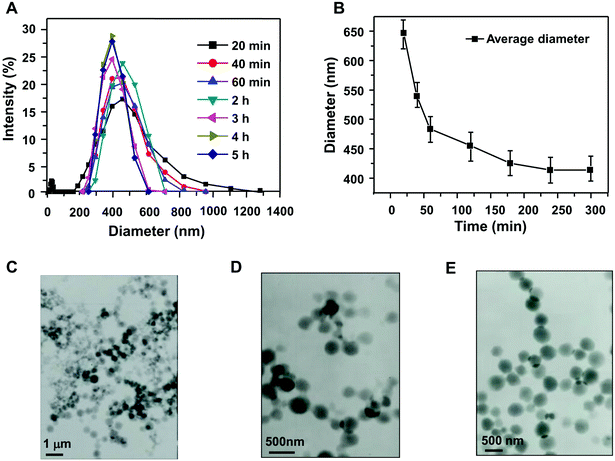 |
| Fig. 2 Effect of reaction time on the particle size distribution (A, B) and TEM micrographs of HPMC/PAA nanogel obtained at different times: (C) 20 min; (D) 60 min; (E) 5 h. | |
3.3 The effect of amount of cross-linker on the nanogel
As shown in Fig. 3, the HPMC/PAA nanogel prepared with 0.5 wt% (against AA) of cross-linker exhibited a 615–1990 nm size distribution with an average particle size of 1203 nm. A smaller amount of cross-linker led to fewer cross-linking points. When the amount of cross-linker was 1%, 2%, and 3%, the corresponding size distribution was 295–1281 nm, 295–955 nm, and 295–712 nm, respectively, becoming gradually narrower. Up to 5% cross-linker, the particle size was distributed between 220 nm and 615 nm with an average nanogel size of 385 nm. However, when the amount of cross-linker continued to increase, the size distribution became again wide, ranging from 190 nm to 825 nm with an average size of 489 nm. In conclusion, in a certain range, HPMC/PAA nanogel size decreased with increasing amount of cross-linker; when the amount of cross-linker increased more than 5% of the monomer AA, the particle size became large again and the size distribution broadened. Excessive cross-linker would make the crosslink density too large, leading to the reaction rate being too fast, resulting in uniform polymerization.
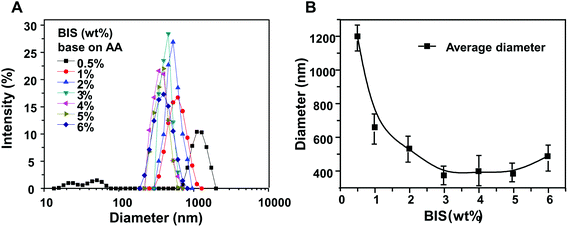 |
| Fig. 3 Effect of the amount of cross-linker (BIS) on the particle size distribution. | |
3.4 Entrapment efficiency and in vitro release behavior of insulin-loaded nanogels
Fig. 4A shows the particle size distribution of insulin-loaded adsorption nanogel and embedding nanogel, which exhibited an average particle size of 588 nm and 466 nm, respectively. This could be explained in that the drug loaded in the adsorption nanogel depends on being adsorbed on the surface of the obtained nanogel. In contrast, the embedding drug-loaded nanogel entraps the drug during the process of forming the nanogel. Thus the former nanogel was larger than the latter. As can be seen from Fig. 4C and D, the drug-loaded nanogels still showed regular spherical morphology.
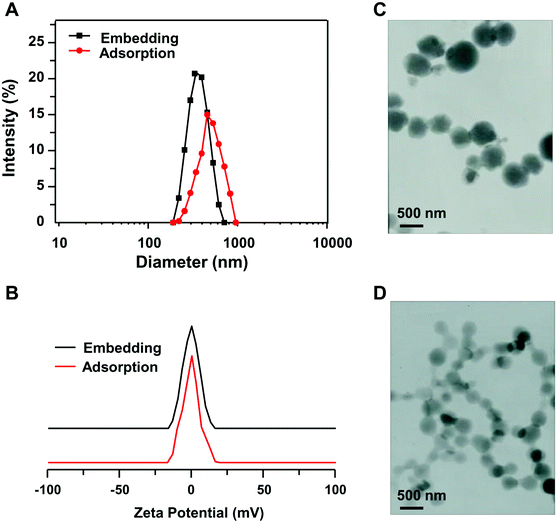 |
| Fig. 4 The particle size distributions (A) and zeta potentials (B) of insulin-loaded nanogels; TEM micrographs of adsorption (C) and embedding (D) insulin-loaded nanogels. | |
In this study, as an example, the cytotoxicity of blank nanogel to HEK293 cells was evaluated using the MTT method. As shown in Fig. 5, even at the higher concentration (1.0 mg mL−1), the nanogel did not show significant cytotoxicity to HEK293 cells, implying that it has low cytotoxicity to HEK293 cells.
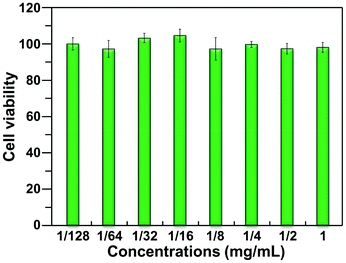 |
| Fig. 5 Cytotoxicity of blank micelles to HEK293 cells determined by MTT viability assay. | |
The insulin loading and encapsulating efficiency are shown in Table 1. It was found that the drug loading and entrapment efficiency of the embedding insulin nanogel were higher than those of the nanogel obtained by the adsorption method. This is because the drug in the adsorption nanogel primarily attached to the particle surface by adsorption force. Entrapping of the drug was completed simultaneously with nanogel formation in the embedding nanogel, and therefore its drug carrying capacity was better than that of the nanogel obtained by the adsorption method. A key benefit of this embedding process is that insulin loading can be accomplished without the use of organic solvents or the addition of external additives.
Table 1 The results of drug loading and entrapment efficiency
Drug-loaded nanogel |
Drug loading (%) |
Entrapment efficiency (%) |
Adsorption nanogel |
5.93 |
59.29 |
Embedding nanogel |
8.68 |
86.78 |
To demonstrate pH-sensitive release, the release of insulin was studied over a period of 108 h at 37 °C in acidic (HCl, pH 1.2) and neutral (PBS, pH 7.4) conditions. As shown in Fig. 6A, original insulin is completely released within 20 h. In contrast, insulin release of adsorption and embedding nanogels reached 60% and 40% at pH 7.4, while only 14% and 7% of the encapsulated insulin was released at pH 1.2. This may be because the carboxyl from PAA ionized at pH 7.4, which resulted in the electrostatic repulsion between charges, driving nanogel swelling, and then the encapsulated insulin readily diffused out from the nanogel. In contrast, the nanogel shrank as a result of hydrogen bonding under acidic conditions: the encapsulated insulin diffused out with more difficulty, resulting in lower release rate.
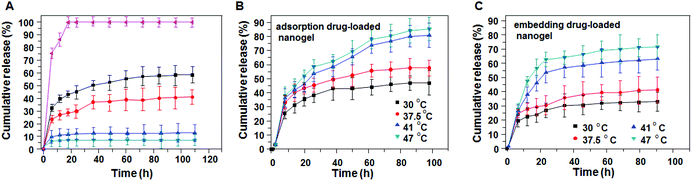 |
| Fig. 6 Cumulative release curves under different conditions. (A) ( ) INS; (■) adsorption nanogel in pH 7.4 PBS; ( ) embedding nanogel in pH 7.4 PBS; ( ) adsorption nanogel in pH 1.2; ( ) embedding nanogel in pH 1.2. (B) Adsorption drug-loaded nanogel at different temperatures. (C) Embedding drug-loaded nanogel at different temperatures. | |
Temperature-sensitive release from the nanogel was also studied. The release temperatures were selected as 30 °C, 37.5 °C, 41 °C and 47 °C. Among them, 41 °C is the LCST of initial nanogel. As shown in Fig. 6B and C, the insulin release rate of the nanogel increased with increasing temperature, there being a significant difference between 37.5 °C and 41 °C. The cumulative release rates of insulin from the adsorption nanogel were 40% and 50% within 20 h at 37.5 °C and 41 °C, respectively. In contrast, under the same conditions, the cumulative release rates of insulin from the embedding nanogel within 20 h were 30% and 50%, respectively. It is obvious that there is a “mutation” of drug release around the LCST. When the temperature was below the LCST, the insulin-loaded nanogel could be in a swollen state, and the insulin slowly diffused out from the network structure; when the temperature exceeded its LCST, the nanogel could shrink dramatically, subsequently squeezing drug from the nanogel, and thus the insulin release rate significantly increased.
3.5
In vivo studies of insulin-loaded nanogel in diabetic mice
Fig. 7 shows the blood glucose level versus time profiles after administration of different insulin formulations to diabetic mice. According to this figure, injection of the free-form insulin solution markedly reduced the blood glucose level within 2–3 days; subsequently the blood glucose level was increased. On the contrary, the insulin-loaded nanogel could more efficiently inhibit the increase in blood glucose level compared to the free insulin group. This result indicated that this nanogel is non-toxic and highly efficient, bringing the clinical application of long-acting insulin release one important step closer to reality.
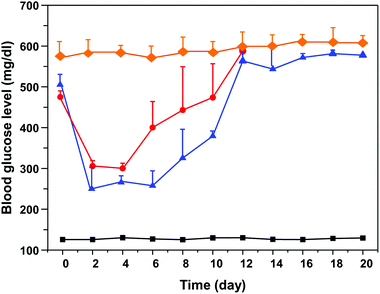 |
| Fig. 7
In vivo blood glucose level of INS-loaded nanogel in streptozotocin-induced diabetic mice: ( ) the diabetic control mice; ( ) the diabetic mice treated with INS at a dose of 4 IU kg−1; ( ) the diabetic mice treated with INS-loaded HPMC/PAA nanogel at a dose of 20 IU kg−1; (■) the normal mice. | |
4. Conclusions
In this work, HPMC/PAA nanogel was successfully synthesized by surfactant-free polymerization. We explored the effects of temperature, time and amount of cross-linker on the composite nanogel size and morphology. The results showed that when the temperature was 41 °C, the reaction time about 4 h, and the amount of cross-linker was 5 wt% with respect to AA, the obtained nanogel is satisfactory with a particle size of 250–615 nm, PDI of 0.112, which illustrated the particle size distribution was nearly monodisperse. The nanogel prepared under each condition showed regular spherical shape. The obtained nanogel can encapsulate insulin via adsorption and embedding methods. More importantly, the hypoglycemic effect of such an insulin-loaded nanogel in diabetic mice was more efficient following a single subcutaneous injection compared to the control group, indicating that this nanogel has a great potential to serve as a novel long-acting insulin release formulation.
Acknowledgements
This research was financially supported by Hefei Tianmai Biotechnology Development Ltd.
Notes and references
- X. Lu, Z. Hu and J. Gao, Macromolecules, 2000, 33, 8698–8702 CrossRef CAS.
- W. H. Blackburn and L. A. Lyon, Colloid Polym. Sci., 2008, 286, 563–569 CAS.
- Y. Kagawa, H. Minami, M. Okubo and J. Zhou, Polymer, 2005, 46(4), 1045–1049 CrossRef CAS PubMed.
- Q. Liao, Q. L. Shao, H. Y. Wang, G. Qiu and X. H. Lu, Polymer, 2012, 87, 2648–2654 CAS.
- Q. Peng, Z. R. Zhang, T. Gong, G. Q. Chen and X. Sun, Biomaterials, 2012, 33, 1583–1588 CrossRef CAS PubMed.
- Y. Yamaguchi, M. Takenaga, A. Kitagawa, Y. Ogawa, Y. Mizushima and R. Igarashi, J. Controlled Release, 2002, 81, 235–249 CrossRef CAS.
- M. M. Henricus, K. T. Johnson and I. A. Banerjee, Bioconjugate Chem., 2008, 19, 2394–2400 CrossRef CAS PubMed.
- R. G. Chaaya, L. Feng, B. Miroslav and W. K. Sung, J. Controlled Release, 1999, 59, 287–298 CrossRef.
- S. Ye, C. Y. Wang, X. X. Liu, Z. Tong, B. Ren and F. Zeng, J. Controlled Release, 2006, 112, 79–87 CrossRef CAS PubMed.
- Y. Chuang, K. J. Lin, F. Y. Su, F. L. Mi, B. Maiti, C. T. Chen, S. P. Wey, T. C. Yen, J. H. Juang and H. W. Sung, J. Controlled Release, 2013, 172(2), 513–522 CrossRef PubMed.
- S. Al-Qadia, A. Grenhab, D. Carrión-Recioa, B. Seijoa and C. Remuñán-López, J. Controlled Release, 2012, 157(3), 383–390 CrossRef PubMed.
- S. Sajeesh, K. Bouchemalb, V. Marsaudc, C. Vauthierc and C. P. Sharmaa, J. Controlled Release, 2010, 147(3), 377–384 CrossRef CAS PubMed.
- M. Amidi, K. M. Krudys, C. J. Snel, D. J. A. Crommelin, O. E. Della Pasqua, W. E. Hennink and W. Jiskoot, J. Controlled Release, 2008, 127(3), 257–266 CrossRef CAS PubMed.
- X. Y. Xiong, Y. P. Li, Z. L. Li, C. L. Zhou, K. C. Tam, Z. Y. Liu and G. X. Xie, J. Controlled Release, 2007, 120(1), 11–17 CrossRef CAS PubMed.
- A. Besheer, K. M. Wood, N. A. Peppas and K. Mäder, J. Controlled Release, 2006, 111(1), 73–80 CrossRef CAS PubMed.
- F. Cuia, K. Shia, L. Q. Zhanga, A. J. Taoa and Y. Kawashima, J. Controlled Release, 2006, 114(2), 242–250 CrossRef PubMed.
- R. S. Yao, J. J. Xu, X. H. Lu and S. S. Deng, J. Nanomater., 2011, 2011, 507542 Search PubMed.
- W. B. Zhang, R. S. Yao and W. Tao,
et al.
, Colloid Polym. Sci., 2014, 292, 317–324 CAS.
- D. Christiane, M. Philippe and U. Nathalie, J. Controlled Release, 2007, 117, 163–170 CrossRef PubMed.
|
This journal is © The Royal Society of Chemistry 2014 |
Click here to see how this site uses Cookies. View our privacy policy here.