DOI:
10.1039/C3CS60193E
(Tutorial Review)
Chem. Soc. Rev., 2014,
43, 135-147
The influence of fluorine in asymmetric catalysis
Received
10th June 2013
First published on 25th October 2013
Abstract
All domains of chemistry are increasingly impacted by organofluorine molecules, often favorably. In asymmetric synthesis of fluorinated compounds, significant achievements are the result of extensive research efforts toward appropriate experimental conditions rather than of the rationalization of fluorine effects. Most of the time, the influence of fluorine is inspected retrospectively. When elaborating a synthetic plan, the question should not be only when and how to introduce fluorine but also how to use the effects of fluorine for a desirable result. The subtle effects of fluorine atom(s) on the course of asymmetric reactions are outlined in this tutorial review. We present some selected examples of asymmetric reactions that involve fluorinated components either as reactants, catalysts, solvents or additives, and a comparative study of the stereochemical outcomes with reactions carried out in the presence of non-fluorinated analogues.
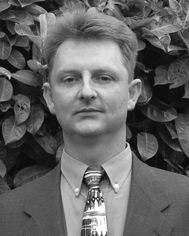
Dominique Cahard
| Dominique Cahard was born in 1968 in Fécamp. He is a CNRS Research Director. He received his PhD degree from the University of Rouen under the direction of Pr. Jean-Marie Poirier and Pr. Pierre Duhamel. He enjoyed two postdoctoral positions with Pr. Chris McGuigan in Southampton (U.K.) and then in Cardiff (Wales), and with Pr. Tadashi Nakata at RIKEN, Tokyo (Japan). In 1996, he joined the CNRS as « Chargé de Recherche », completed his Habilitation in 2001, and was promoted to « Directeur de Recherche » in 2007. He has published about 100 papers, patents, and book chapters. His current research interests concern innovative methodologies for asymmetric synthesis of fluorinated molecules. |
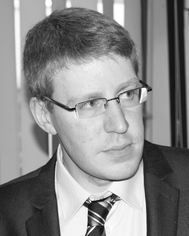
Vincent Bizet
| Vincent Bizet received his MSc in 2009 from the University of Caen, and completed his PhD in 2012 in UMR CNRS 6014 COBRA under the direction of Dr Dominique Cahard. He is currently a postdoctoral research associate with Pr. Carsten Bolm at the RWTH Aachen University and was awarded an Alexander von Humboldt Fellowship. His current research interests concern the asymmetric synthesis of fluorinated molecules and sulfoximines. |
Key learning points
(1) The chemistry of non-fluorinated molecules cannot be simply translated to the chemistry of fluorinated molecules.
(2) A comparison of the stereochemical outcomes of reactions run with fluorinated versus non-fluorinated components and a rationalisation of the influence of fluorine provide relevant mechanistic information.
(3) Judicious installation of fluorine atom(s) is a powerful tool to orient the reactivity of substrates as well as to develop novel highly stereodiscriminating catalysts.
(4) A better understanding of the factors governing the stereoselectivity is key in the development of asymmetric organofluorine chemistry.
|
Introduction
So many superlatives have been written and said about fluorine: “Fabulous fluorine”, “Fervid fluorine”, “Seductive fluorine”, “A magic atom”, “A small atom with a big ego”, as well as the neologism “Flustrates” has been coined by Prof. D. Seebach to refer to fluorine-containing substrates.1 “Fluorine leaves nobody indifferent; it inflames emotions, be that affections or aversions” said Prof. M. Schlosser in 1998.2 Fluorine is definitely a phenomenon atom and the more we learn about fluorine compounds the more we realize how exciting fluorine really is. Indeed, fluorine chemistry is an invitation to a fascinating science. Surprises often emerge from experiments in the field of fluorine chemistry because organofluorine compounds quite often fail to follow empirical generalizations derived from the behavior of similar organic combinations containing hydrogen or other halogen in place of fluorine. A typical example is the alkylation reaction by means of alkyl halides that act as electrophilic alkyl reagents, whereas perfluoroalkyl halides RfX do not behave similarly because the synergistic electronegativities of the fluorine atoms are higher than those of the X halide. The polarisation is thus reversed and reaction with a nucleophile is prohibited at the carbon centre; instead, a halophilic reaction takes place with attack of the nucleophile at the X halogen of RfX. Many such contradictions have been encountered by chemists in the early stage of development of organofluorine chemistry and even today. As a consequence, chemists who are unfamiliar with fluorine are surprised at the difficulties frequently faced in this class of compounds. Despite advances in our understanding of the “fluorine effect”, further studies on the reactivity of fluorine molecules are highly relevant since the chemistry of non-fluorinated molecules cannot be simply translated to the chemistry of fluorinated molecules.
Fluorine is undeniably the element that has experienced the highest recent interest in several aspects of chemistry as evidenced by the huge number of publications that deal with efficient synthetic methods, sophisticated approaches, and novel applications. The reasons for such a craze for fluorine chemistry are to be found in the intrinsic properties of the atom. The effect of fluorine as a structural element is very subtle and a comprehensive understanding of the properties of fluorine is regarded as a prerequisite to control reaction pathways in a desired sense. Fluorine is the most electronegative element on the Pauling scale (χ = 3.98), so the C–F bond is highly polarised with a strong ionic character making it the strongest single bond in organic chemistry (C–F binding energy = 115.7 kcal mol−1versus 98.0 kcal mol−1 for C–H). Fluorine and hydrogen atoms have van der Waals radii of 1.47 and 1.20 Å, respectively; hence, replacement of a hydrogen by a fluorine causes minimal steric alteration. However, its strong electronegativity and hydrogen-bonding aptitude make a fluorine atom more akin to a hydroxyl group. In any event, introduction of fluorine atom(s) into a molecule modifies its physico-chemical properties through steric, electronic, and conformational effects. Indeed, the partial negative charge on the fluorine atom suggests intra- and intermolecular electrostatic interactions that have obvious consequences on the conformation of the molecules. Dipole–dipole interactions are rather weak when observed intermolecularly while such interactions taking place within an organofluorine compound become significantly stronger. Electrostatic interactions are more pronounced with charged adjoining substituents such as an ammonium or a protonated alcohol. This effect is well known to cause the gauche preference in FCH2CH2X systems. In addition, stereoelectronic aspects should be taken into account to assess the influence of the C–F bond on the conformation of fluorinated molecules, on the reaction mechanisms, and on intermediates or transition states. A consequence of the highly polarised C–F bond is the low-energy σ*C–F antibonding orbital located in alignment and opposite of the C–F bond. This vacant orbital can accommodate electron density from electron rich heteroatom lone pairs (O, N), C–H and π bonds, as well as nucleophiles. This effect is known as hyperconjugation, and it significantly stabilises certain conformations. For example, the axial preference and anomeric stabilisation for 2-fluoropyran (Δeq–ax ≈ 2.8–2.9 kcal mol−1) are consistent with oxygen lone pair donation into the σ*C–F orbital.3 A case study is the 1,2-difluoroethane that has two main staggered conformations, with the fluorine atoms either gauche or anti. Although one might think that the two fluorine atoms should repel because of electrostatic repulsion, the gauche conformer was determined to be about 0.5–0.9 kcal mol−1 more stable than the anti conformer. In this case, the hyperconjugative interaction goes beyond electrostatic destabilising effects. For a wider coverage of the fundamental aspects of the C–F bond properties, we recommend the reader to refer to the Chem. Soc. Rev. tutorial review of Prof. David O'Hagan.3
A deeper understanding of fluorine effects is a continuing challenge to tame chemical reactions of organofluorine molecules in a desired sense, ultimately in a predictable fashion. In bioorganic and medicinal chemistry, the many roles of fluorine are deeply explored and have been the subject of a number of excellent reviews.4,5 In asymmetric synthesis, the potential of fluorine as a tool to control newly created stereogenic centers is seldom investigated. In this tutorial review, the stereochemical behaviour of fluorinated molecules used as reactants, catalysts, or solvents in asymmetric syntheses is described and the results are compared, when the data are available, with the results of equivalent reactions in fluorine-free chemistry. The first section provides examples of asymmetric reactions that involve fluorinated substrates with a discussion of the role and the impact of fluorine substitution. A comparison of the stereochemical outcomes of reactions run with non-fluorinated substrates is described. In the second part, we comment on the use of fluorinated catalysts which by means of privileged conformations or binding affinities pre-organise and minimise reaction intermediates or transition states. The third part is devoted to the beneficial use of fluorinated solvents, cosolvents and additives in asymmetric reactions accompanied by a comparison with the commonly employed solvents. The presentation is by no way an exhaustive review of the examples available in the literature but will help to integrate the subject and to critically interpret results in fluorine chemistry.
1. Fluorinated versus non-fluorinated substrates
The literature is abound with examples of highly efficient asymmetric syntheses of which a number of methods have been applied to substrates that feature a single fluorine atom often in place of a hydrogen atom or a fluorocarbon moiety, CH2F, CHF2, CF3, or Rf.6 It is a gross understatement to say that fluorinated reactants do not behave similar to their non-fluorinated analogues; nevertheless, a detailed comparison with rationalisation of the influence of fluorine is rarely mentioned. Hereafter, we have compiled meaningful examples found by a direct inspection of the literature and by computer search of databases. A comparison of reaction yields, diastereo- or enantiomeric ratios, and mechanistic pathways is provided.
Catalytic enantioselective Diels–Alder reaction: a kinetic effect
The enantioselective Diels–Alder reaction of cyclopentadiene with fluorinated dienophiles has been studied by Haufe et al.7 and later by Shibatomi, Yamamoto et al.8 It was first observed that α-fluoroenones and α-fluoroacrylates reacted significantly slower than the non-fluorinated dienophiles because the fluorine atom increases the π-electron density of the C
C bond by its p–π interaction (+M effect). In terms of enantioselectivity, the reaction best performed with 2-fluorohept-1-en-3-one (1) and cyclopentadiene in the presence of a catalytic amount of a Lewis acid-activated oxazaborolidine (Scheme 1).8 Reaction of 1 with cyclopentadiene gave the [4+2] Diels–Alder adduct 2 in good yield with unexpected high exo selectivity and 94% ee for the exo adduct (endo/exo nomenclature is related to the stereochemistry of the carbonyl substituent). On the other hand, ethyl vinyl ketone (3) and cyclopentadiene gave almost exclusively the normal endo selective cycloaddition product 4 in a better yield for a shorter reaction time and with a higher ee value.
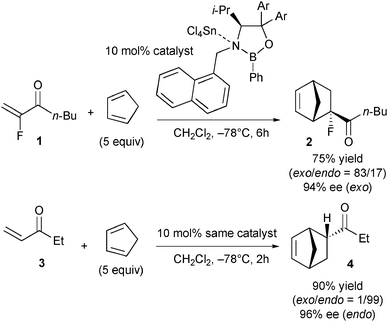 |
| Scheme 1 Lewis acid-catalysed enantioselective Diels–Alder reaction. | |
The origin of the exo selectivity for the fluorinated Diels–Alder adduct 2 is not clearly documented. It could be assigned to secondary orbital interactions between the diene and the fluorine atom, or to a combination of solvent effects and electrostatic forces, but certainly not to hydrogen bonds nor to steric interactions. Haufe et al. investigated the influence of the fluoro substituent on the barrier and reaction energy by density functional theory calculations of the cycloaddition of cyclopentadiene with but-3-enone and 3-fluorobut-3-enone. The results suggested a kinetic effect of the fluorine rather than a higher thermodynamic stability of the exo product.9
Ruthenium-catalysed hydrogenation of 2-fluoro-2-alkenoic acids: a directing effect
The ruthenium(II)-catalysed hydrogenation of fluorinated alkenoic acids Z- and E-5 afforded the corresponding fluorinated acid 6 as the same major (R) enantiomer whatever the double-bond geometry of the alkenoic acid 5 with 91 and 83% ee, respectively (Scheme 2).10 The hydrogenation occurred on the Si face at C-2 and the fact that the same enantiomer was obtained regardless of the configuration of the alkenoic acid 5 implies that the enantiofacial differentiation is controlled by the C-2 prostereogenic centre of the substrate.
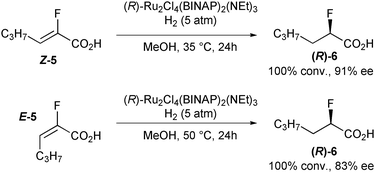 |
| Scheme 2 BINAP-Ru-catalysed enantioselective hydrogenation of 2-fluoro-2-hexenoic acid. | |
For a comparison of the stereochemical outcome with a non-fluorinated substrate, we found the ruthenium(II)-catalyzed hydrogenation of 2-methyl-2-butenoic acid E-7 (tiglic acid) and Z-7 (angelic acid).11 The ruthenium(II)-catalysed hydrogenation of the E diastereoisomer led to the (R)-2-methylbutanoic acid 8 with 91% ee whereas the Z diastereoisomer led to the opposite enantiomer (S)-8 albeit with a lower ee value, at only 57% ee (Scheme 3).
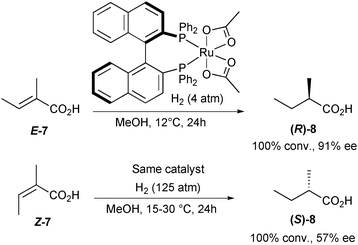 |
| Scheme 3 BINAP-Ru-catalysed enantioselective hydrogenation of 2-methyl-2-butenoic acid. | |
In sharp contrast to the Ru-BINAP hydrogenation of 2-fluoro-2-alkenoic acids, the opposite configuration obtained for non-fluorinated substrates implied that the enantiofacial differentiation is preferentially controlled by the C-3 carbon centre of the substrate. Although the catalysts are not identical and we are comparing reactants that feature a fluorine atom or a methyl group, this case study illustrates the influence of a C–F bond in the key stereodifferentiating step.
Enantioselective reduction of prochiral fluorine-containing carbonyl compounds: a size effect
Reduction with organoboranes.
Brown, Ramachandran et al. observed a difference of enantiofacial selectivity during the reduction of non-fluorinated and fluoroalkylated ketones catalysed by (−)-B-chlorodiisopinocampheylborane [(−)-DIP-Chloride™] (Scheme 4).12 Reduction of acetophenone led to the corresponding (S) alcohol with 98% ee. In a comparative experiment, the reduction of 2,2,2-trifluoroacetophenone unexpectedly gave the (S) isomer with 90% ee. Due to CIP stereochemistry rules, the absolute configuration is the same but the reduction occurred on the opposite face of the 2,2,2-trifluoroacetophenone. A methyl to CF3 substitution significantly increases the steric bulk since the van der Waals volume of CF3 is greater than that of a methyl and similar to that of an isopropyl group. The CF3 group acts as the enantiocontrolling larger group as compared to the methyl group. In addition, the pivalophenone substrate having a t-Bu group gave the (R) enantiomer. This clearly indicates that the t-Bu group in the favored transition state is placed in the same position as the CF3 group on 2,2,2-trifluoroacetophenone. Hence, steric factors appear to govern the stereochemical outcome of these reactions although it means that the volume of the CF3 group would be greater than that of the phenyl group, which is questionable and does not take into account the shape and flexibility of each substituent. An alternative explanation to rationalise the effect of the CF3 group in the inversion of stereochemistry would be to involve a chelating effect of the fluorine and the strong Lewis acidic boron atom in the borane–ketone charge–dipole complex.13 However, smaller fluorinated groups such as CH2F and CHF2 displayed the same enantiofacial selectivity observed for acetophenone and gave the corresponding (R) alcohols with 95% and 85% ee, respectively (Scheme 4).
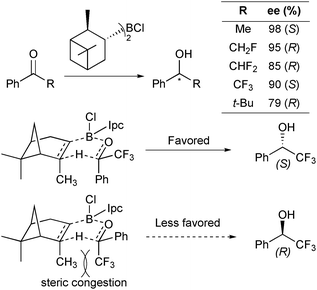 |
| Scheme 4 Diverging effects of steric congestion on DIP-chloride™ enantioselective reduction of fluoromethyl ketones. | |
Several other chiral reducing agents have been evaluated in the enantioselective reduction of this type of ketone but no general trend emerged because alcohols of opposite configurations were obtained depending on the reagent. For example, the structurally related (−)-B-isopinocampheyl-9-borabicyclo[3.3.1]nonane [R-alpine-borane] gave the same sense of asymmetric induction for tri-, di- and monofluoroacetophenone albeit with different ee values, 32, 97 and 89%, respectively.
Enantioselective hydroboration of prochiral ketones by means of chiral oxazaborolidines
The Corey–Bakshi–Shibata (CBS) reduction by means of a catalytic amount of oxazaborolidine with catecholborane as the stoichiometric reductant is a very useful and efficient approach for the reduction of prochiral ketones. Under these conditions, trifluoracetylmesitylene 9 was reduced into alcohol (R)-10 and its non-fluorinated analogue acetylmesitylene 11 gave alcohol (R)-12 in quantitative yields with ee values of 100% or nearly so (Scheme 5).14 The sense of enantioselectivity is thus reversed for 9 and 11. According to the well-established reaction mechanism of the CBS reduction, the catalyst coordinates the oxygen lone pair anti to the larger keto substituent. In the case of 9, the stereochemistry of the alcohol (R)-10 implies that the oxazaborolidine coordinates with 9 selectively at the lone pair anti to the CF3 group rather than at the lone pair syn to CF3. It means that the CF3 group is considered to be the larger group of the keto substituents. The preference for this transition state, which must be significantly high in view of the excellent ee value obtained, may not only be the result of the very large spherical space of the CF3 group but also the result of substantial electrostatic repulsion between the electron rich fluorine atoms of the CF3 group and the negatively charged boron in the ketone–oxazaborolidine complex that favors coordination of the catalyst at the lone pair anti to the CF3 group. To further gain information on the stereochemical behaviour of trifluoromethylketones, an X-ray crystallographic study of 9-anthryl trifluoromethyl ketone revealed a pronounced displacement of the carbonyl oxygen toward the CF3 group and away from the 9-anthryl carbon. Such distortion can be caused by hyperconjugation of a lone pair on oxygen with the anti-bonding σ* orbital of the C–C bond between C
O and CF3 groups (Scheme 5).
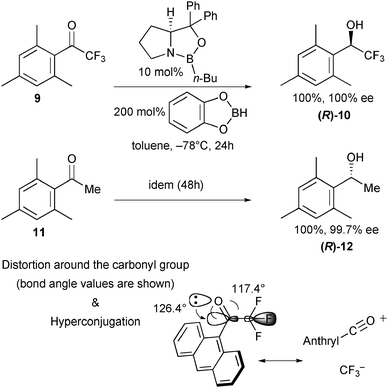 |
| Scheme 5 Reverse sense of enantioselectivity in hydroboration of CF3vs. Me ketones. | |
Transition metal-catalysed enantioselective hydrogenation
The enantioselective hydrogenation of trifluoromethyl ketones catalysed by chiral rhodium(I)-(amidephosphine-phosphinite) complexes was studied by Kuroki et al.15 Interestingly, the challenging hydrogenation of aliphatic ketones gave high enantioselectivities despite similar steric demand of both aliphatic side chains. For instance, hydrogenation of trifluoromethyl n-octyl ketone gave the corresponding alcohol in 99% yield with 97% ee. Under the same reaction conditions, di- and monofluoro analogues are quantitatively converted but the enantioselectivities dramatically dropped to 27% and 15%, whereas the corresponding non-fluorinated ketone remained unchanged in the process (Scheme 6). No explanation was given to account for the reactivity and enantioselectivity differences; however, the electron-withdrawing effect of at least one fluorine atom appeared strong enough to increase the electrophilicity of the carbonyl function and thus its reactivity. Regarding the enantioselectivity the more fluorine atoms there were, the higher the enantioselectivity was.
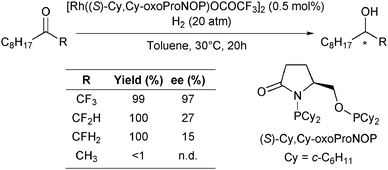 |
| Scheme 6 Cumulative effects of fluorine atoms on enantioselective hydrogenation of fluoromethyl alkylketones. | |
Nevertheless, these observations are specific to this catalytic system because the asymmetric hydrogenation of various fluorinated and non-fluorinated ketones with Noyori's ruthenium complexes gave comparable results in terms of reactivity and enantioselectivity.16
Transition metal-catalysed enantioselective transfer hydrogenation
The asymmetric transfer hydrogenation (ATH) is a valuable method and an excellent alternative to hydrogenation by means of hydrogen gas. The asymmetric version, which was first developed by Noyori,17 did not escape investigations with fluoroalkyl ketones. For example, [Ru(p-cym)(S,S)-(piperidinylSO2DPEN)] (DPEN = 1,2-diphenylethylenediamine) in the presence of the HCO2H–NEt3 azeotropic mixture reduced the benzyl trifluoromethyl ketone with 97% ee whereas phenyl acetone was reduced with only 5% ee and a poor 13% conversion (Scheme 7a). In contrast, 2,2,2-trifluoroacetophenone was reduced with only 38% ee whereas acetophenone gave the alcohol in 95% ee (Scheme 7a).18 The sense of asymmetric induction is the opposite for benzylic and arylic substrates indicating that the CF3 group is smaller than the phenyl and bigger than the benzyl. Here, the steric bulk difference of the two keto group substituents was found to be the major factor in determining enantiofacial selectivity as well as ee level. In particular, the size of the phenyl group appeared to be not much different from the CF3 group.
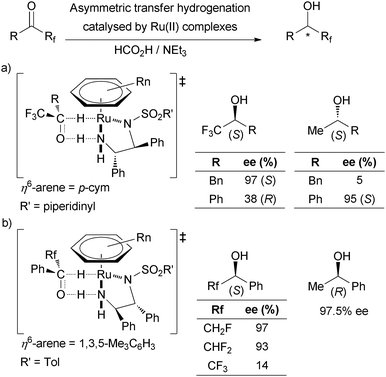 |
| Scheme 7 Sense of asymmetric induction in transfer hydrogenation. | |
The steric effect was further evidenced in the ATH of phenyl ketones featuring 0, 1, 2, or 3 fluorine atoms (Scheme 7b).19 In all cases, the hydride delivery took place on the same relative face providing the (S) enantiomer for fluorinated alcohols and the (R) enantiomer for 1-phenylethanol. The enantioselectivity decreased with the increase of the steric bulk in the order Me < CH2F < CHF2 < CF3 < Ph. Hence, the lower selectivity observed in the reduction of 2,2,2-trifluoroacetophenone relative to that of acetophenone could be attributed to the smaller size difference between the two substituents.
Organocatalytic asymmetric Strecker reaction of fluorinated ketoimines: hydrogen-bonding effect
The asymmetric Strecker reaction of difluoro- and trifluoromethyl ketoimines reported by Wang, Zhou et al. allowed a direct comparison with the Strecker reaction of the corresponding methylketoimine. The substrates were reacted with trimethylsilyl cyanide in the presence of a chiral urea derivative of quinine to give the expected products in high yield albeit with totally different enantioselectivities (Scheme 8).20 Indeed, the difluoromethylated α-aminonitrile product was obtained in 73% yield with 87% ee and the trifluoromethylated α-aminonitrile was obtained in 97% yield with 94% ee whereas the non-fluorinated α-methyl-α-aminonitrile was isolated as a racemic mixture. The bifunctional catalyst features a Brønsted acid moiety for H-bonding interactions with the imine and a Lewis base moiety to activate the nucleophile TMSCN. In addition, hexafluoroisopropanol (HFIP) was used as an additive to accelerate the reaction without affecting the enantioselectivity. Calculations showed different hydrogen-bonded complexes. In the case of fluorinated ketoimines, the urea established hydrogen bonds with the imine nitrogen N⋯H–N and with a fluorine atom C–F⋯H–N (Scheme 8) while the non-fluorinated imine forms two H-bonds with both urea hydrogens. According to the authors, this recognition model may account for the strong fluorine effect on enantioselectivity observed between non-fluorinated and fluorinated ketoimines.
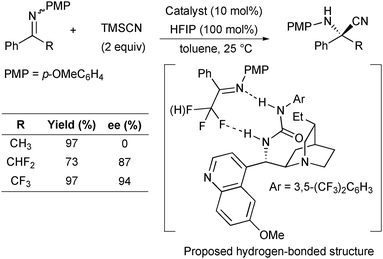 |
| Scheme 8 Fluorine effect on enantiofacial selectivity of ketoimines in the Strecker reaction. | |
Enantioselective versus enantiospecific isomerisation of CF3-allylic alcohols: an electron-withdrawing effect
Ikariya et al. reported the enantioselective isomerisation of secondary allylic alcohols catalysed by ruthenium complexes (Scheme 9a).21 Of particular interest is the observation that the chiral Cp*Ru(PN) catalyst 13 derived from proline isomerised efficiently the secondary allylic alcohols 14 into the corresponding ketone 15 in 95% yield with up to 74% ee; 15 is an intermediate in the total synthesis of muscone.
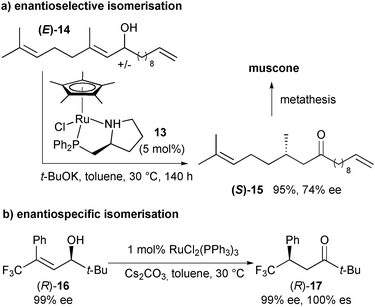 |
| Scheme 9 Fluorine-induced enantiospecific isomerisation of γ-CF3 allylic alcohols (enantiospecificity: es = 100× ee reactant/ee product). | |
In our group, we recently evaluated the isomerisation of γ-CF3 secondary allylic alcohols in order to develop the enantioselective synthesis of β-CF3 ketone. Unfortunately, all our attempts led to racemic ketones. The origin of this problem is to be found in the rate-determining step of the reaction. A detailed mechanistic study with a model γ-CF3 secondary allylic alcohol ascertained the operative pathway in which an intramolecular syn-specific 1,3 hydride shift takes place within the coordination sphere of the ruthenium center.22 In a comparison with non-fluorinated allylic alcohols, a specific fluorine effect was observed that permutes the rate determining step from insertion for non-fluorinated allylic alcohols to β-elimination for fluorinated allylic alcohols (Scheme 10). Overall, the reaction with γ-CF3 allylic alcohols is very much accelerated relative to non-fluorinated substrates due to the presence of the strong electron-withdrawing CF3 group. As a consequence, the enantioselective isomerisation of γ-CF3 allylic alcohols produced only racemic ketones. Very efficiently, the problem was circumvented through the discovery of an enantiospecific isomerisation process in which the stereochemical information of the starting optically enriched γ-CF3 allylic alcohols is entirely transferred to the chiral isomerisation products. For example, the enantioenriched γ-CF3 allylic alcohol (R)-16 with 99% ee was isomerised with achiral RuCl2(PPh3)3 to give the corresponding β-CF3 ketone (R)-17 with 99% ee as well, and thus with 100% es (Scheme 9b).22
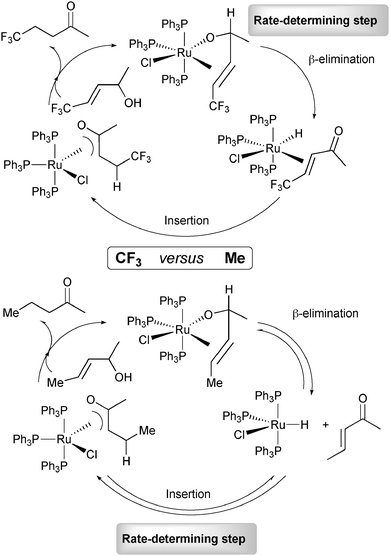 |
| Scheme 10 A comparative study of the reaction mechanisms of isomerisation of allylic alcohols. | |
Diastereoselective allylation of 4-fluoroproline methyl ester: stereoelectronic and chelation effects
This example illustrates the manifestation of the fluorine-induced conformational control in cyclic structures. The diastereoselective allylation of 4-fluoroproline methyl ester, as reported by Filosa et al., showed high level of diastereofacial selection for both (4R)- and (4S)-4-fluoro-N-Boc-L-proline methyl esters (18). The allylation proceeded anti to the fluorine atom with excellent diastereomeric ratios (d.r. up to 97
:
3 from (4R)-18) (Scheme 11).23 To account for the high diastereoselectivity, the authors proposed the formation of a transitory cyclic species, in which the fluorine is chelated to lithium. The allylation took place on the Si face of the enolate. Alternatively, a transition state that implies a fluorine-amide gauche effect, with allylation occurring from the less sterically crowded convex face, was suggested by Gilmour et al.24 Furthermore, we cannot exclude a synergistic combination of both effects to account for the excellent diastereoselection.
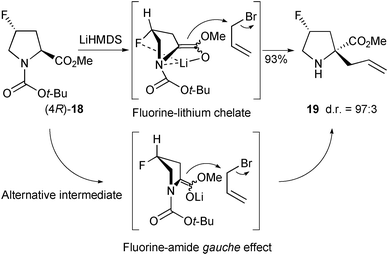 |
| Scheme 11 Illustration of fluorine-induced conformationally organised intermediates. | |
Diastereoselective alkylation by assistance of a potassium–fluorine interaction: a chelation with metal effect
The diastereoselective alkylation of α-alkoxyesters25 and glycinates26 that feature fluorinated chiral auxiliaries has been studied by Yamazaki et al. The presence of at least one fluorine atom was responsible for a significant improvement in the diastereoselection from 2–56% to up to 90% de (Scheme 12). After deprotonation of the substrate with KHMDS, the configuration of the resulting potassium enolate is frozen (exclusively Z) by intramolecular chelation with the α-heteroatom (O or N) and further forms a bicyclo [3.3.0]-type structure through intramolecular potassium⋯fluorine interactions. Ab initio computation of a model lithium enolate indicated a Li⋯F distance of 1.879 Å, 0.043 Å shorter than the Li⋯O distance, and a concomitant elongation of the C–F bond (0.072–0.078 Å).25 The electrophilic addition occurred preferentially by the more accessible convex face (Re-face) of the enolate (Scheme 12). The size of the cation was revealed to be crucial for the reactivity and a potassium atom appeared to be necessary to get high yields. In this reaction, the fluorine atom has a minimum steric effect but imparts excellent discrimination of the enolate faces thus leading to significantly improved diastereoselectivities relative to the use of non-fluorinated substrates. The number of fluorine atoms was also investigated and gave diastereoisomeric excesses in the same range for CH2F, CHF2 and CF3 groups.
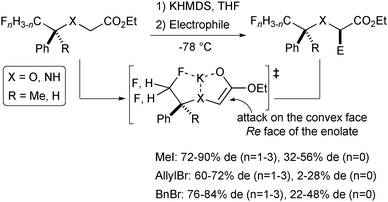 |
| Scheme 12 Assistance of intramolecular potassium⋯fluorine interactions in diastereoselective alkylations of frozen Z enolates. | |
2. Fluorinated catalysts
The fluorine atom acts as a chemically inert steering group able to induce conformational organisation of catalysts through stereoelectronic effects by stabilisation of privileged conformations and thus minimising transition-state intermediates. Indeed, the judicious introduction of a fluorine atom at the appropriate place on a catalyst skeleton can dramatically enhance diastereo- and enantiofacial discrimination; hence, fluorine is regarded as a control element of the catalyst's topology. Several widely used classes of chiral organocatalysts have benefited from the influence of fluorine, in particular owing to the in-depth investigation of Prof. Ryan Gilmour.24 In this section, we showcase examples of monofluorinated or lightly fluorinated catalysts but we do not deal with highly fluorinated catalysts that fall into the category of fluorous catalysts.27
Fluorinated N-heterocyclic carbenes in Stetter reaction: a stereoelectronic effect
The asymmetric intermolecular Stetter reaction of heterocyclic aldehydes with nitroalkenes was studied by Rovis et al. with the aid of bicyclic triazolium salts as precursors of N-heterocyclic carbenes to generate Breslow intermediates. Fluorinated triazolium 21 demonstrated enhanced reactivity and enantioselectivity as compared to the non-fluorinated analogue 20 (Scheme 13). In this example, the substitution of one hydrogen atom by a fluorine had minimal steric influence but was responsible for a strong stereoelectronic effect imposing a privileged conformation. X-ray analysis of 21 ascertained a preference for the Cγ-exo conformer featuring the fluorine atom and the i-propyl group in pseudoaxial positions with unfavorable steric 1,3-diaxial interactions. To overcome the inherent steric bias for this conformation, a combination of stereoelectronic effects could explain the Cγ-exo conformational preference (Scheme 13). The X-ray structure of 21 clearly showed orbital alignment of the σ*C–F with an adjacent σC–H bonding orbital and an additional hyperconjugative gauche interaction of one of the triazolium nitrogens with the C–F bond (Scheme 13). Hence, the improved enantioselectivity with the fluorinated triazolium salt 21 was attributable to the conformational change in the bicyclic ring system that allowed a more stereoselective orientation of the incoming nitroalkene.28
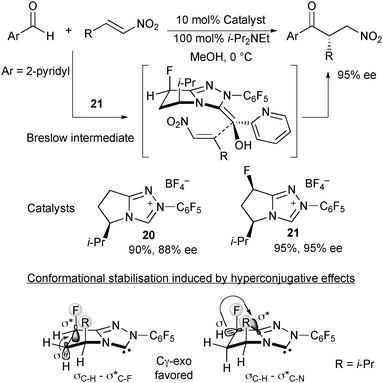 |
| Scheme 13 Conformational effect of fluorine on triazolium catalyst in the Stetter reaction. | |
Fluorinated cinchona alkaloids in enantioselective fluorination: a stereoelectronic effect
Cinchona alkaloids are privileged structures in asymmetric synthesis as ligands and catalysts owing to their natural abundance and easy supply. A recurring problem associated with these molecules is the high conformational lability that causes the multiplication of transition-state assemblies. Gilmour et al. have designed fluorinated cinchona alkaloid derivatives in which the C9 hydroxyl group is replaced by a fluorine atom with the aim of freezing preferential configurations thanks to stereoelectronic and electrostatic effects. For example, in the N-benzyl-C9-fluorocinchonine 22a, the gauche effect of the β-fluoroammonium motif restricts internal rotation around the C8–C9 bond and constrains the structure to adopt an open conformation (Scheme 14, top).29 Catalyst 22a was evaluated as a phase transfer agent in the enantioselective electrophilic fluorination of cyclic β-keto esters and compared with four other N-benzylated cinchonine derivatives 22b–e (Scheme 14, bottom). (9S)-Fluorocinchonine 22a gave the best result with 96% yield and 78% ee. A similar reactivity was observed with 9-deoxycinchonine 22b (94% yield) and cinchonine 22c (90% yield); however, the enantioselectivities were much lower (10 and 8% ee, respectively). Increasing the bulkiness of the hydroxyl group of cinchonine by methylation (22d) or by silylation (22e) showed similar reactivities (86% yield) but led to a noteworthy improvement of the enantioselection, 64 and 57% ee, respectively, but still lower as compared to the use of 22a. Different catalyst conformations in the enantiodiscriminating step are likely to be involved within this series of catalysts. Nevertheless, the introduction of a fluorine atom allows one to restrict internal rotation around the C8–C9 bond of cinchona alkaloids in a predictable way. An additional strategy to control C9–C4′ rotation as well would be desirable to fully constrain the structure, hopefully in a favorable way.
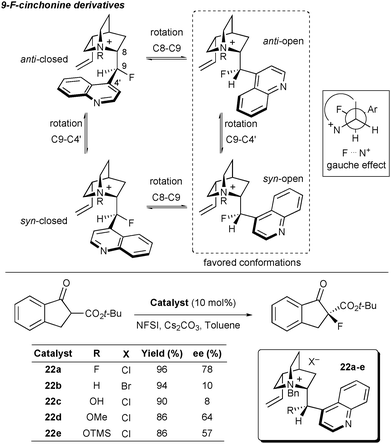 |
| Scheme 14 Restricted internal rotation in C9-fluoro cinchona alkaloids: impact on the phase-transfer catalysed electrophilic fluorination. | |
4-Fluoroproline in asymmetric transannular aldolisation: a stereoelectronic effect
Chandler and List described the transannular aldolisation of cyclic diketones catalysed by 4-substituted prolines including cis- and trans-4-fluoro prolines (Scheme 15).30 Substitution of proline at the 4-position by a trans-hydroxy or a trans-tert-butoxy group led to 10% improvement of the enantioselectivity compared to proline itself, whereas the trans-tert-butyldimethylsilyl group gave only 22% ee. trans-4-Fluoroproline was identified as the most efficient catalyst and led to the bicyclic compound in 75% yield with 80% ee. In contrast, the cis-4-fluoroproline gave poor results with only 50% yield and 58% ee. The relative and absolute stereocontrol was rationalised by the authors through a transition-state model implying a hydrogen-bonding interaction (Scheme 15, bottom left).31 Nonetheless, because of the greater distance between the 4-substituent and the site of ring annulation, Gilmour proposed that the observed beneficial effect of fluorine could be due to a preferred conformational organisation dictated by the fluorine–iminium ion gauche effect resulting from combined stereoelectronic effects (σ*C–F → σC–H hyperconjugation) and a charge–dipole interaction (N+⋯Fδ−) (Scheme 15, bottom right).24 Hence, the role of hydrogen bonding interactions that are commonly proposed for proline catalysis is minimal in this interpretation. Switching from trans-4-fluoroproline to its cis-stereoisomer dramatically lowered both reactivity and stereoselectivity, clearly indicating that a subtle change of the catalyst structure has a strong impact on the global outcome of the reaction. This study has already contributed to the design of novel, efficient, fluoroproline derivatives as part of the “tool box” of catalysts in asymmetric synthesis.32
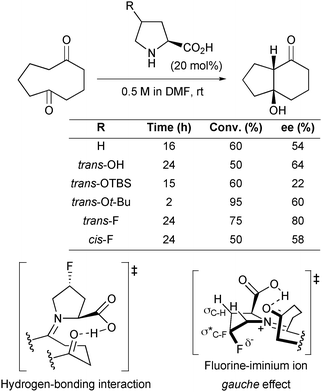 |
| Scheme 15 Transannular aldolisation: proline substitution effect. | |
2-(Fluorodiphenylmethyl)pyrrolidine in asymmetric epoxidation: a stereoelectronic effect
(S)-2-(Fluorodiphenylmethyl)pyrrolidine 2333 is an organocatalyst derived from proline that has been used in the asymmetric epoxidation of α,β-unsaturated aldehydes by Gilmour et al. (Scheme 16).34 Catalyst 23 was condensed with an aldehyde to generate a positively charged iminium species whose syn-clinal endo conformation is governed by the dynamic electrostatic gauche effect together with stabilising hyperconjugative interactions between the low-lying σ*C–F orbital and the adjacent σC–H bond (in the solid state, the angle ϕNCCF was measured at 56.6°). Calculations confirmed the preference for the gauche over the anti conformation for the fluorinated E iminium ion (+16.5 kJ mol−1), whereas in the nonfluorinated analogue the gauche conformer was favored by only 5–6 kJ mol−1. This mode of activation through iminium ion facilitates the addition of hydrogen peroxide to the less encumbered Si face of the E-iminium system. Indeed, the Re face is shielded by an aromatic ring due to the conformation imposed by the fluorine gauche effect. Next the intermediate enamine is intercepted to form the trans epoxide as the main diastereoisomer (Scheme 16).35
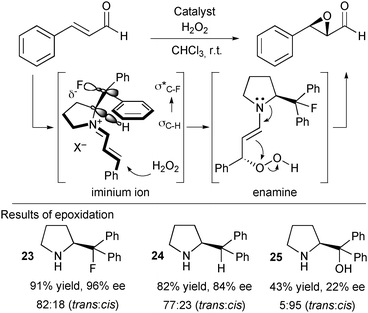 |
| Scheme 16 The fluorine–iminium ion gauche effect on the stereoselective epoxidation of α,β-unsaturated aldehydes. | |
In the epoxidation reaction of trans-cinnamaldehyde, catalyst 23 provided the corresponding epoxide in 96% ee and a 82
:
18 (trans
:
cis) diastereoisomeric ratio.35 In a comparative experiment, epoxidation with non-fluorinated catalyst 24 led to a lower enantioselectivity (85% ee). This epoxidation reaction was previously described with the hydroxylated catalyst 25, but a much lower enantioselectivity was obtained (only 22% ee for an incomplete conversion) but with the opposite trans
:
cis selectivity (5
:
95) compared to catalyst 23.36 Once again, it has been demonstrated that the design of fluorinated catalysts allows preorganisation of the reaction intermediates in a favorable way to induce superior enantioselectivity.
Fluorinated imidazolidinones in Friedel–Crafts alkylation: a stereoelectronic effect
MacMillan's imidazolidinone-based organocatalysts are complementary to proline derivatives to serve as efficient catalysts for a wide variety of asymmetric reactions that proceed through iminium-ion activation. How stereoinduction is conferred for this covalent organocatalysis is still up to debate. So it is not surprising that researchers have investigated the dominant conformations and configurations of iminium-ion intermediates responsible for high enantioinduction.37 Gilmour et al. have reported mechanistic studies involving fluorinated imidazolidinones 28a and 28b in Friedel–Crafts-type alkylation of trans-cinnamaldehyde with N-methyl pyrrole (Scheme 17). It was envisaged that the fluorine–iminium ion gauche effect could be exploited to design conformational probes for organocatalysis. To perform this study, the syntheses of diastereomeric fluorinated iminium salts 28a and 28b were realised. X-ray analyses and 1H NMR studies confirmed a syn-clinal endo topology for 28a (conformer with the fluorine atom over the heterocyclic ring) and a syn-clinal exo one for 28b resulting from a gauche fluorine effect in both cases. These structures have the thermodynamically more stable E-configured +N
C bonds. The E/Z ratio for 28a was clearly lower compared to that for 27 or 28b; however, iminium 27 and 28a led to 78 and 28% ee, respectively, in the conjugate addition of N-methylpyrrole, whereas 28b gave a racemic mixture (Scheme 17). These results indicated that the benzyl shielding group in the exo-Ph conformer 28a was responsible for the better enantioselectivity as compared with 28b (this is in support of the MacMillan's intuitive hypothesis for enantioinduction) and the endo-Ph conformer 28b secured a higher level of geometric control.38
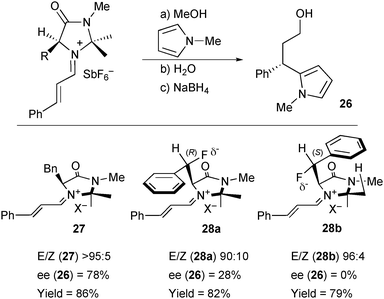 |
| Scheme 17 Use of the fluorine–iminium gauche effect to create conformer equivalents. | |
Fluorinated ketone in asymmetric epoxidation of alkenes: a stereoelectronic effect
The asymmetric epoxidation of alkenes by means of ketone-derived dioxiranes generated in situ from oxone and a ketone has been the subject of intense design of chiral α-fluoroketones that proved to be suitable owing to activating effects both for reactivity and for stereoinduction. For example, stoichiometric use of non-fluorinated ketone 29 showed very low reactivity with only 6% conversion for β-methylstyrene after 8 h. Monofluoroketone 30 led to epoxide in 33% conversion with 79% ee after 11 h reaction. α,α′-Difluoroketone 31 gave the highest ee value whereas gem-difluoroketone 32 was the most effective promoter of the reaction and gave similar reactivity and enantioselectivity (Scheme 18).39 A catalytic version of this reaction was performed with 30 mol% of ketone 31 giving the corresponding epoxide in 80% yield and 88% ee. The similar enantioselectivities obtained with catalysts 31 and 32 seem to indicate that fluorine atoms in the α-position of ketone have little impact on the enantioselectivity, but were necessary to enhance the electrophilicity of the carbonyl function and formation of the dioxirane. However, fluorine substituents in equatorial or axial positions imparted a noticeable stereoelectronic control that caused a dramatic conformational preference in the transition states of the epoxidation reaction.40 Moreover, the fluorine atom has a significant impact on competing processes such as hydration and Baeyer–Villiger decomposition of the catalyst that have to be taken into account for the overall efficiency of chiral α-fluoroketones.
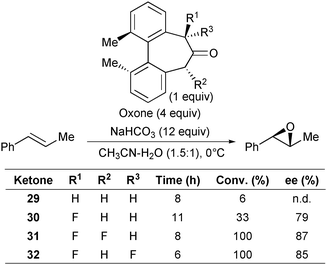 |
| Scheme 18 Fluorinated ketones as catalysts in asymmetric epoxidation. | |
Fluorinated oxazoline amines in enantioselective hetero-Diels–Alder reactions: hydrogen-bonding effect
Hydrogen-bond-donating catalysts are effective in a variety of catalytic reactions including the hetero-Diels–Alder reaction in which some oxazoline-based catalysts featuring a pendant amide group such as 33 (Scheme 19) have been investigated. The acidity of the N–H proton was systematically changed by modifying the R group in 33. An increasing electron-withdrawing capacity of the R group led to an improved enantioselection in the asymmetric hetero-Diels–Alder reaction between Rawal's diene and benzaldehyde. The pKa values of the corresponding acid RCO2H were found to correlate with the observed reaction rate and enantioselectivity (Scheme 19). Product 34 was obtained with the highest enantiomeric excess measured for the most acidic catalyst featuring a CF3 group. A mechanism was proposed in which benzaldehyde binds reversibly to 33 to form an activated complex, which reacts irreversibly with Rawal's diene to give the adduct. The increasing amide acidity in catalyst 33 generates a more tightly bound substrate, which thereby increases the rigidity in the transition state and the enantiomeric ratio.41
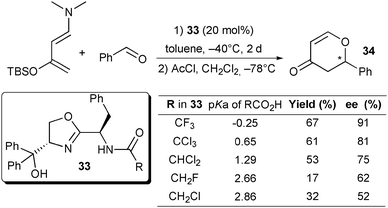 |
| Scheme 19 Evaluation of hydrogen-bond catalysts in (hetero)-Diels–Alder reactions. | |
Fluorinated phosphoric acids in enantioselective (hetero)-Diels–Alder reactions: favorable π–π stacking interactions
BINOL-derived phosphoric acids belong to the well established class of chiral Brønsted acid catalysts that have been successfully used in a number of asymmetric transformations. 3,3′-Substitution of the BINOL moiety is key to high stereoinduction. Fluorinated derivatives are frequent and the role of fluorine atom(s) is often to modulate the pKa of the acid and to bring steric bulk in the case of CF3 group. The remote effect of fluorine substitution for 3,3′-phenyls (from mono- to pentafluoroaryls) was investigated in hetero-Diels–Alder reactions with a combination of chiral phosphoric acid 35 and InBr3. Cyclopentadiene was reacted with (E)-methyl 2-oxo-4-phenylbut-3-enoate to afford the hetero-Diels–Alder adduct 36 as a major product along with the Diels–Alder adduct 37 (Scheme 20). Substitution of 3,3′-aryl groups with one fluorine atom in the para, meta or ortho position led to a gradual increase of enantioselectivity as compared to the non-fluorinated phenyl group, from 80 to 98% ee for 36 and from 80 to 96% ee for 37. However, there was no significant change in the 36/37 ratio. With a pentafluorophenyl group, the enantioselectivity reached 99% ee for the two adducts together with improved 36/37 ratio in favor of 36 and diastereoisomeric ratio of 37 (98
:
2). The magnitude of the fluorine effect is highly position sensitive and a significant positive stereoeffect was obtained with ortho-substitution of the aryl. Moreover, a single ortho-fluoro atom sufficed to deliver very high stereocontrol comparable to that observed with the pentafluorophenyl substitution. It has been suggested that the C6F5 groups of the catalyst participate favorably in π–π stacking interactions with the reactants leading to adducts in excellent yields and enantiomeric excesses.42
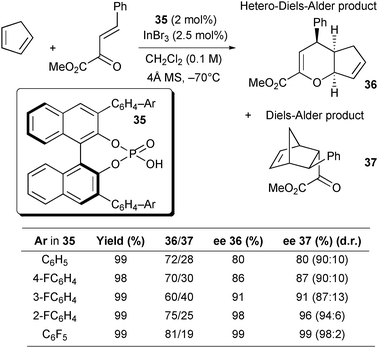 |
| Scheme 20 Remote fluoro-effect on stereocontrol in (hetero)-Diels–Alder reactions. | |
3. Fluorinated solvents, cosolvents and additives
Fluorinated alcohols possess unique physicochemical properties, e.g. low nucleophilicity, high hydrogen bonding donor ability, high ionizing power, and higher acidity than non-fluorinated analogues. The effects of fluorinated alcohols on reactivity, regioselectivity, and stereoselectivity of various reactions have been examined and reviewed.43,44 The most frequently used fluorinated alcohols are trifluoroethanol (TFE) and hexafluoroisopropanol (HFIP) whose physical properties are compared to that of ethanol in Table 1. Rationalisation of the positive effects of fluorinated solvents is not often provided but it is commonly suggested that the weak nucleophilicity, the proton source, and the low coordinative nature of these species account for the superior results.
Table 1 Physical properties of TFE, HFIP and EtOH
|
TFE |
HFIP |
EtOH |
TFE: CF3CH2OH; HFIP: (CF3)2CHOH. |
bp (°C) |
73.8 |
58.6 |
78 |
pKa |
12.4 |
9.3 |
15.9 |
Nucleophilicity (N) |
−2.78 |
−4.23 |
0 |
Dielectric constant (ε) |
26.7 |
16.7 |
24.5 |
Polarity (ENT) |
0.898 |
1.068 |
0.654 |
Ionizing power (Y) |
1.8 |
3.82 |
−1.75 |
Hydrogen bond donor (α) |
1.51 |
1.96 |
0.83 |
Fluorinated alcohols as solvents in asymmetric hydrogenation: acidity and stabilising effects
Amii, Uneyama et al. reported the asymmetric palladium-catalysed hydrogenation of α-trifluoromethylated iminoesters in fluorinated solvents. A dramatic solvent effect was observed in the asymmetric hydrogenation of iminoester 38 as illustrated in Scheme 21. Ordinary solvents (toluene, AcOH, MeOH, EtOH) gave α-aminoester 39 with low to moderate yields and enantioselectivities. Moreover, nucleophilic solvents like methanol and ethanol attacked the imine function to give α-alkoxylated aminoester by-products. Gratifyingly, fluorinated alcohols used as solvents dramatically improved enantioselectivities and gave almost quantitative yields. The best solvent was found to be trifluoroethanol, which led to quantitative yield and 88% ee.45 Considering the high reactivity of fluorinated imines, fluorinated alcohols appeared to be considerably weaker nucleophiles and less coordinative compared to non-fluorinated alcohols. The role of fluorinated alcohols was rationalized as a stabilizer of the active palladium catalyst through weak coordination. The acidity of the fluorinated alcohols might also increase the electrophilicity of the imino group by protonation or hydrogen bonding.
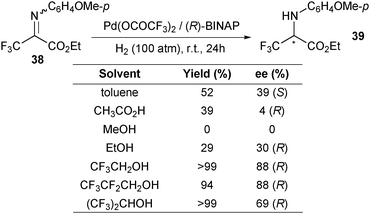 |
| Scheme 21 Effects of solvents on hydrogenation reaction. | |
Variation of the solvent in the catalytic hydrogenation of itaconates 40 mediated by a rhodium complex of phosphine–phosphoramidite ligand (42) provided opposite configurations for the hydrogenated products 41. In trifluoroethanol (TFE), (R)-41 was obtained in up to 99.4% ee whereas the same catalytic system in methyl ethyl ketone (MEK) gave the enantiomer (S)-41 in up to 71.2% ee (Scheme 22). This interesting observation allowed to obtain both enantiomers using the same ligand varying only the solvent, where usual homogeneous catalysis needs the synthesis of the enantiomeric ligand.46 This rare observation alerts us to the fact that different mechanisms may operate as a function of the reaction solvent.
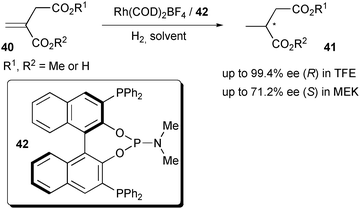 |
| Scheme 22 Solvent-driven divergent enantioinduction. | |
Fluorinated alcohols as additives in asymmetric electrophilic fluorination: assistance in catalyst turnover
Several examples of catalytic systems have demonstrated higher efficiency in the presence of a fluorinated alcohol as additive.44 For instance, the electrophilic fluorination of β-ketoesters with N-fluorobenzenesulfonimide (NFSI) catalyzed by a copper(II)–bis(oxazoline) combination showed a real improvement of the enantioselectivity on addition of an achiral fluorinated alcohol such as HFIP (1 equivalent). Without the additive the α-fluoro-β-ketoester was obtained in 96% yield with 73% ee. The use of bases or molecular sieves did not improve the enantioselectivity and required longer reaction times. However, the addition of one equivalent of HFIP increased the ee value significantly to 85% (Scheme 23). The role of HFIP is to assist the catalyst turnover via the release of the fluorinated substrate from the catalyst.47
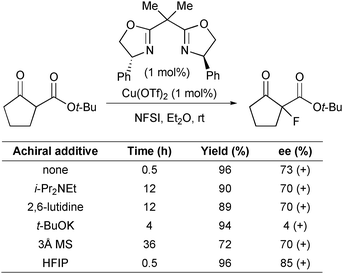 |
| Scheme 23 Effects of a fluorinated additive on enantioselectivity. | |
4. Conclusion and perspectives
Fluorine is a multifaceted atom that offers interesting opportunities with a view to modulating stereoselectivity. The many fluorine effects illustrated in this tutorial review have provided a better understanding of how this element controls reactivity and stereochemistry. We can say with certainty that judicious installation of fluorine atom(s) is a powerful tool to orient the reactivity of substrates as well as to develop novel highly stereodiscriminating catalysts. In addition, the unique properties of fluorinated alcohols as solvents or additives render them worth investigating in any asymmetric transformation. Asymmetric organofluorine chemistry has a bright future thanks to a more accurate understanding of the factors governing the stereoselectivity. Further explorations of the strategic incorporation of fluorine into reaction components are actively pursued and will hence prove valuable in many areas in general organic chemistry.
Notes and references
- D. Seebach, Angew. Chem., Int. Ed. Engl., 1990, 29, 1320–1367 CrossRef.
- M. Schlosser, Angew. Chem., Int. Ed., 1998, 37, 1496–1513 CrossRef CAS.
- D. O'Hagan, Chem. Soc. Rev., 2008, 37, 308–319 RSC.
- W. K. Hagmann, J. Med. Chem., 2008, 51, 4359–4369 CrossRef CAS PubMed.
- S. Purser, P. R. Moore, S. Swallow and V. Gouverneur, Chem. Soc. Rev., 2008, 37, 320–330 RSC.
- D. Cahard, X. Xu, S. Couve-Bonnaire and X. Pannecoucke, Chem. Soc. Rev., 2010, 39, 558–568 RSC.
- M. Essers, T. Ernet and G. Haufe, J. Fluorine Chem., 2003, 121, 163–170 CrossRef CAS.
- K. Shibatomi, K. Futatsugi, F. Kobayashi, S. Iwasa and H. Yamamoto, J. Am. Chem. Soc., 2010, 132, 5625–5627 CrossRef CAS PubMed.
- M. Essers, C. Mück-Lichtenfeld and G. Haufe, J. Org. Chem., 2002, 67, 4715–4721 CrossRef CAS PubMed.
- M. Saburi, L. Shao, T. Sakurai and Y. Uchida, Tetrahedron Lett., 1992, 33, 7877–7880 CrossRef CAS.
- T. Ohta, H. Takaya, M. Kitamura, K. Nagai and R. Noyori, J. Org. Chem., 1987, 52, 3174–3176 CrossRef CAS.
- P. V. Ramachandran and H. C. Brown, ACS Symp. Ser., 2000, 746, 22–37 CrossRef CAS PubMed.
- P. V. Ramachandran, B. Gong and A. V. Teodorović, J. Fluorine Chem., 2007, 128, 844–850 CrossRef CAS PubMed.
- E. J. Corey, X.-M. Cheng, K. A. Cimprich and S. Sarshar, Tetrahedron Lett., 1991, 32, 6835–6838 CrossRef CAS.
- Y. Kuroki, Y. Sakamaki and K. Iseki, Org. Lett., 2001, 3, 457–459 CrossRef CAS.
- T. Ohkuma, M. Koizumi, H. Doucet, T. Pham, M. Kozawa, K. Murata, E. Katayama, T. Yokozawa, T. Ikariya and R. Noyori, J. Am. Chem. Soc., 1998, 120, 13529–13530 CrossRef CAS.
- S. Hashiguchi, A. Fujii, J. Takehara, T. Ikariya and R. Noyori, J. Am. Chem. Soc., 1995, 117, 7562–7563 CrossRef CAS.
- D. Sterk, M. Stephan and B. Mohar, Org. Lett., 2006, 8, 5935–5938 CrossRef CAS PubMed.
- S. V. Slungard, T.-A. Krakeli, T. H. K. Thvedt, E. Fuglseth, E. Sundby and B. H. Hoff, Tetrahedron, 2011, 67, 5642–5650 CrossRef CAS PubMed.
- Y.-L. Liu, T.-D. Shi, F. Zhou, X.-L. Zhao, X. Wang and J. Zhou, Org. Lett., 2011, 13, 3826–3829 CrossRef CAS PubMed.
- M. Ito, S. Kitahara and T. Ikariya, J. Am. Chem. Soc., 2005, 127, 6172–6173 CrossRef CAS PubMed.
- V. Bizet, X. Pannecoucke, J.-L. Renaud and D. Cahard, Angew. Chem., Int. Ed., 2012, 51, 6467–6470 CrossRef CAS PubMed.
- R. Filosa, C. Holder and Y. P. Auberson, Tetrahedron Lett., 2006, 47, 8929–8932 CrossRef CAS PubMed.
- L. E. Zimmer, C. Sparr and R. Gilmour, Angew. Chem., Int. Ed., 2011, 50, 11860–11871 CrossRef CAS PubMed.
- T. Yamazaki, M. Ando, T. Kitazume, T. Kubota and M. Omura, Org. Lett., 1999, 1, 905–908 CrossRef CAS.
- T. Yamazaki, S. Kawashita, T. Kitazume and T. Kubota, Chem.–Eur. J., 2009, 15, 11461–11464 CrossRef CAS PubMed.
-
G. Pozzi, Enantioselective Homogeneous Supported Catalysis, The Royal Society of Chemistry, 2012, pp. 159–205 Search PubMed.
- D. A. DiRocco, K. M. Oberg, D. M. Dalton and T. Rovis, J. Am. Chem. Soc., 2009, 131, 10872–10874 CrossRef CAS PubMed.
- E.-M. Tanzer, W. B. Schweizer, M.-O. Ebert and R. Gilmour, Chem.–Eur. J., 2012, 18, 2006–2013 CrossRef CAS PubMed.
- C. L. Chandler and B. List, J. Am. Chem. Soc., 2008, 130, 6737–6739 CrossRef CAS PubMed.
- S. Bahmanyar, K. N. Houk, H. J. Martin and B. List, J. Am. Chem. Soc., 2003, 125, 2475–2479 CrossRef CAS PubMed.
- A. Quintard, J.-B. Langlois, D. Emery, J. Mareda, L. Guénée and A. Alexakis, Chem.–Eur. J., 2011, 17, 13433–13437 CrossRef CAS PubMed.
- D. O'Hagan, F. Royer and M. Tavasli, Tetrahedron: Asymmetry, 2000, 11, 2033–2036 CrossRef CAS.
- C. Sparr, W. B. Schweizer, H. M. Senn and R. Gilmour, Angew. Chem., Int. Ed., 2009, 48, 3065–3068 CrossRef CAS PubMed.
- E.-M. Tanzer, L. E. Zimmer, W. B. Schweizer and R. Gilmour, Chem.–Eur. J., 2012, 18, 11334–11342 CrossRef CAS PubMed.
- G.-L. Zhao, I. Ibrahem, H. Sundén and A. Córdova, Adv. Synth. Catal., 2007, 349, 1210–1224 CrossRef CAS.
- D. Seebach, R. Gilmour, U. Grošelj, G. Deniau, C. Sparr, M.-O. Ebert, A. K. Beck, L. B. McCusker, D. Šišak and T. Uchimaru, Helv. Chim. Acta, 2010, 93, 603–634 CrossRef CAS.
- C. Sparr and R. Gilmour, Angew. Chem., Int. Ed., 2010, 49, 6520–6523 CrossRef CAS PubMed.
- S. E. Denmark and H. Matsuhashi, J. Org. Chem., 2002, 67, 3479–3486 CrossRef CAS PubMed.
- A. Armstrong, I. Washington and K. N. Houk, J. Am. Chem. Soc., 2000, 122, 6297–6298 CrossRef CAS.
- K. H. Jensen and M. S. Sigman, Angew. Chem., Int. Ed., 2007, 46, 4748–4750 CrossRef CAS PubMed.
- J. Lv, L. Zhang, S. Hu, J.-P. Cheng and S. Luo, Chem.–Eur. J., 2012, 18, 799–803 CrossRef CAS PubMed.
- J. P. Bégué, D. Bonnet-Delpon and B. Crousse, Synlett, 2004, 18–29 Search PubMed.
- I. Shuklov, N. V. Dubrovina and A. Börner, Synthesis, 2007, 2925–2943 CrossRef CAS.
- H. Abe, H. Amii and K. Uneyama, Org. Lett., 2001, 3, 313–315 CrossRef CAS.
- W. Zhang and X. Zhang, J. Org. Chem., 2007, 72, 1020–1023 CrossRef CAS PubMed.
- J.-A. Ma and D. Cahard, Tetrahedron: Asymmetry, 2004, 15, 1007–1011 CrossRef CAS PubMed.
|
This journal is © The Royal Society of Chemistry 2014 |
Click here to see how this site uses Cookies. View our privacy policy here.