DOI:
10.1039/C4MH00037D
(Communication)
Mater. Horiz., 2014,
1, 411-418
Ultrasonication-switched formation of dice- and cubic-shaped fullerene crystals and their applications as catalyst supports for methanol oxidation†
Received
28th February 2014
, Accepted 22nd April 2014
First published on 24th April 2014
Abstract
Three-dimensional (3D) nanostructures of an endohedral fullerene, Sc3N@C80 (Ih), including both cubic-shaped and unprecedented dice-shaped crystals, have been successfully prepared for the first time via a modified liquid–liquid interfacial precipitation (LLIP) method integrating ultrasonication. By simply switching ultrasonication on/off upon mixing the good/poor (mesitylene/isopropanol) solvents, the dice- and cubic-shaped Sc3N@C80 crystals have been selectively prepared, and their size can be readily controlled by varying the concentration of the starting Sc3N@C80 solution in mesitylene. The growth mechanism of the cubic- and dice-shaped Sc3N@C80 crystals has been proposed, highlighting the formation of local mesitylene cavities which lead to the nucleation and growth of Sc3N@C80 molecules, while the formation of the dice structure. i.e., a hole in each facet of the cube, was primarily due to the hindered diffusion of Sc3N@C80 solutes by ultrasonication-generated gas microbubbles. The crystal structure of the Sc3N@C80 cubes has been determined as a simple cubic unit cell with a lattice constant of a = 10.8 Å. Finally the dice-shaped Sc3N@C80 crystals were applied as a Pt catalyst support for the methanol oxidation reaction (MOR), revealing the improved MOR activity compared to the cubic-shaped Sc3N@C80 crystals due to its larger surface area resulting from the dice (hole) structure.
1. Introduction
Since the first success in the macroscopic production of fullerenes, along with the facile formation of different forms of C60 crystals,1 the study of fullerene crystals with controllable morphology, size and dimensions, which show potential applications in catalysis, field effect transistors and photoelectric devices etc., has been attracting considerable attention.2–5 So far, vapor-driven and solution-driven methods are the most common for the preparation of fullerene crystals.6–11 In particular, as a facile method affording predetermined morphology and improved uniformity of the crystals, the solution-based liquid–liquid interfacial precipitation (LLIP) method has been widely used for the growth of different types of single-crystalline C60 with tunable morphologies ranging from one-dimensional (1D) rods or tubes to 2D plates.12–16 However, the preparation of 3D fullerene nanostructures, specifically cubic-shaped C60 crystals, has been rarely reported. Instead, single-crystalline C70 cubes have been successfully prepared in two reports. In 2010, Choi et al. used the LLIP method and succeeded in fabricating the first cubic-shaped C70 crystals, which exhibited enhanced photoluminescence over that of C70 powders.17 Recently Wågberg et al. also accomplished the preparation of C70 microcubes by using a fast solvent-assisted evaporation method with aromatic solvents and halogen radicals as a controller.18 Thus, the formation of 3D fullerene crystals, particularly cubes, seems to be dependent on the nature of the fullerene, and an intriguing question to be addressed is whether cubic- or other shaped 3D crystals could be formed for other types of fullerenes, including endohedral fullerenes which represent a special type of fullerenes with atoms, ions or clusters entrapped in carbon cages.19–23
During the process of crystal nucleation and growth, any negligible perturbation may result in a considerable change to the final crystal in terms of morphology and size. In particular, ultrasonication has recently been widely implemented in the crystal growth of different molecules including fullerenes such as C60 because it can readily induce gas microbubbles which function as physical matrices for nanocrystal nucleation and growth, and consequently affect the supersaturation level required for nucleation and the final particle size distribution.4,24–27 However, to our knowledge, to date, ultrasonication perturbation has not been applied in the crystal growth of endohedral fullerenes.28–32
Herein, we report the first preparation of 3D nanostructures of an endohedral fullerene, Sc3N@C80 (Ih), via a modified LLIP method integrating ultrasonication, affording both cubic-shaped and unprecedented dice-shaped crystals, which can be tuned by simply switching ultrasonication on/off upon mixing the binary solvents. The growth mechanism of the morphology-tunable Sc3N@C80 crystals was proposed on the basis of a systematic study on the effects of ultrasonication, good/poor solvents and their mixing ratio on the morphology of the Sc3N@C80 crystals. Finally the dice- and cubic-shaped Sc3N@C80 crystals were applied as Pt catalyst supports for the methanol oxidation reaction (MOR), and the correlation between the morphology and the MOR activity of the Sc3N@C80 crystals was unveiled.
2. Experimental
2.1. Materials
Sc3N@C80 (Ih) was synthesized by a modified Krätschmer–Huffman DC-arc discharging method and isolated with a high purity (>99%), as reported previously.33 Other reagents, including the solvents (mesitylene and isopropanol), were commercially available, and used without further purification.
2.2. Preparation
The preparation procedure of the dice-shaped Sc3N@C80 crystals using a modified LLIP method is shown in Scheme 1 (route (I)). The detailed procedure was as follows: 3 mL isopropanol was quickly added into 1 mL Sc3N@C80 solution in mesitylene (0.1 mg mL−1) with ultrasonication (KQ5200B ultrasonicator, 200 W, 40 KHz, Kunshan Ultrasonic Instruments Co., Ltd, China) switched on in a 5 mL glass vessel, and then ultrasonication was performed for 5 min. A large amount of microbubbles emerged and Sc3N@C80 molecules nucleated and crystallized during the ultrasonication process. After ultrasonication, the mixture solution became light grey. Finally the mixture was kept at room temperature for 12 h for crystal growth.
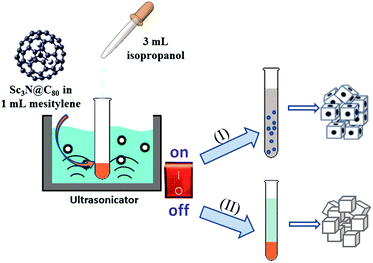 |
| Scheme 1 A schematic illustration of the procedure to prepare the dice- and cubic-shaped Sc3N@C80 crystals via a modified LLIP method integrating ultrasonication. | |
The preparation procedure of cubic-shaped Sc3N@C80 crystals using the traditional LLIP method was as follows: 3 mL isopropanol was slowly added into 1 mL Sc3N@C80 solution in mesitylene (0.1 mg mL−1) in a 5 mL glass vessel by keeping a placid interface between mesitylene and isopropanol (see route (II) of Scheme 1). The sample vessel was then shaken by hand for 1 min, resulting in a homogeneous yellowish mixture. The mixture solution was then ultrasonicated for 5 min and many small microbubbles formed, in which Sc3N@C80 molecules nucleated and crystallized. After ultrasonication, the sample solution became light grey. Finally the mixture was kept at room temperature for 12 h for crystal growth.
2.3. Preparation procedure of Pt/Sc3N@C80 crystals/ITO electrodes
Sc3N@C80 crystals were dispersed in acetone and then transferred to a small cell in which two ITO electrodes were kept at a distance of 5 mm by a Teflon spacer. A DC voltage of 120 V was applied between the two electrodes using a DC power supply. The electrode surface connected to the positive terminal became dark grey after the deposition of Sc3N@C80 crystals, and then the film was dried in air. Two different types of modified ITO electrodes, namely Sc3N@C80 dice/ITO, Sc3N@C80 cubes/ITO as well as blank ITO electrodes, were exposed to an aqueous solution of K2PtCl6 (1 mM) and LiClO4 (1 M) for reduction, and consequently platinum (Pt) nanoparticles were formed and deposited onto the Sc3N@C80 crystal film by applying a potential at 315 mV vs. the Ag/AgCl electrode. The amount of Pt deposited as determined from coulometry was ∼16 μg.
The electrochemical active surface area measurement was carried out in 0.5 M H2SO4 solution. The scan rate was 100 mV s−1. Sc3N@C80 crystals/ITO electrodes were used as the working electrode. A platinum plate and an Ag/AgCl electrode were used as the counter and reference electrode, respectively. The electrochemical active surface area of the catalyst was calculated by measuring the charge for hydrogen desorption, QH, according to formula (1):34
| 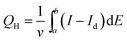 | (1) |
where
v represents the scanning speed,
Id represents the current modifying value of the double layer, and (
a,
b) is the voltage range of hydrogen desorption. Then
QH was used to calculate the active platinum area of the electrodes according to the following formula:
34 |  | (2) |
where
MPt represents the platinum loading in the electrode and
QHref represents the charge required to oxidize a monolayer of H
2 on flat Pt (210 μC cm
−2).
34
2.4. Measurements
SEM imaging was carried out on a JSM-7401F instrument (JEOL, Japan). High resolution transmission electron microscopy (HR-TEM) and selected area electron diffraction (SAED) measurements were made by applying a drop of the sample to a copper grid using a JEOL-2010 TEM instrument (JEOL, Japan). X-ray diffraction (XRD) data were collected with a TTR-III instrument (RIGAKU, Japan) using graphite monochromator filtered Cu Kα radiation. The samples for XRD analysis were prepared by dropping samples onto a silicon substrate in air. Thermal gravimetric analysis (TGA) was carried out under a 100 sccm N2 gas flow on a DTG-60H instrument (Shimadzu, Japan). Methanol oxidation reaction (MOR) measurements were performed in 2.0 M methanol and 1.0 M H2SO4 solution on a 660A electrochemical analyzer (CH instruments Inc., USA) at room temperature. Pt/Sc3N@C80 crystals/ITO electrodes were used as the working electrode. A platinum plate and a Ag/AgCl electrode were used as the counter and reference electrodes, respectively. The scan rate was 20 mV s−1. Chronoamperometric measurements were also performed on the 660A electrochemical analyzer at 0.81 V.
3. Results and discussion
3.1. Preparation of dice- and cubic-shaped Sc3N@C80 (Ih) crystals
Sc3N@C80 (Ih) crystals were prepared by the LLIP method as shown in Scheme 1 with mesitylene and isopropanol used as the “good” solvent for dissolving Sc3N@C80 (Ih) and the “poor” solvent for precipitation, respectively. High-purity (>99%) Sc3N@C80 (Ih, hereafter the label of isomer Ih is omitted for clarity), which was synthesized by a modified Krätschmer–Huffman DC-arc discharging method,33 was dissolved in mesitylene with variable concentrations (0.05, 0.1 mg mL−1), and then 3 mL isopropanol was slowly added into 1 mL Sc3N@C80 solution in mesitylene by two different means. In the first set of the study, the addition of isopropanol into Sc3N@C80 solution in mesitylene was made by keeping a placid interface between these two solvents (see route (II) of Scheme 1). After the complete mixing of isopropanol and mesitylene via shaking by hand followed by ultrasonication treatment, the Sc3N@C80 molecules nucleated and crystallized, and the SEM images of the prepared Sc3N@C80 crystals are shown in Fig. 1A, with the initial concentration of Sc3N@C80 solution in mesitylene set as 0.1 mg mL−1. Clearly uniform cubic-shaped crystals with an average size of ∼0.8 μm were obtained (see Fig. S1A, ESI†), indicating the successful preparation of 3D microcubes of Sc3N@C80.
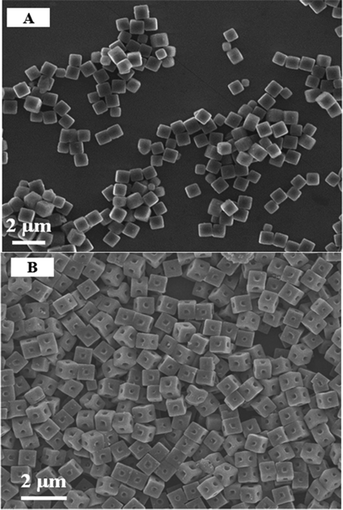 |
| Fig. 1 SEM images of cubic- (A) and dice-shaped (B) Sc3N@C80 crystals prepared by the LLIP method with or without ultrasonication. The concentration of the Sc3N@C80 solution in mesitylene was 0.1 mg mL−1. | |
Interestingly, when isopropanol was added rapidly into the Sc3N@C80 solution in mesitylene (0.1 mg mL−1) and ultrasonication was switched on simultaneously (see route (I) of Scheme 1), while the following procedure was exactly the same as that used for the growth of cubic-shaped crystals, the resultant crystals change to uniform dice shapes according to the SEM image (Fig. 1B), and the average size of the dice is 0.9–1.0 μm (see Fig. S1B, ESI†). Since the dramatic changes of both the morphology (dice vs. cube) and size result from simply switching ultrasonication on/off upon mixing the binary solvents (isopropanol and mesitylene), this result indicates the strong influence of perturbation induced by ultrasonication on the crystal nucleation and growth of the Sc3N@C80 crystals.
For both cases of cubic- and dice-shaped Sc3N@C80 crystals, the size of the final crystals is found to be sensitively dependent on the initial concentration of Sc3N@C80 solution in mesitylene, while when the initial concentration of Sc3N@C80 solution in mesitylene decreases to 0.05 mg mL−1, the average size of the Sc3N@C80 cubes (ultrasonication off) and dice (ultrasonication on) increases to ∼2.0 and 2.1 μm, respectively (see Fig. S2, ESI†). This phenomenon is in accordance with other fullerene nanostructures reported in the literature,8–16 and can be interpreted by the formation of more nucleation sites leading to more crystal seeds and a larger concentration depletion of Sc3N@C80 molecules under a higher concentration of Sc3N@C80 solution. Therefore, the size of the dice- or cubic-shaped Sc3N@C80 crystals can be readily controlled by simply varying the concentration of the starting Sc3N@C80 solution in mesitylene.
3.2. Effects of ultrasonication, good/poor solvents and their mixing ratio on the morphology of Sc3N@C80 crystals
To unveil the role of ultrasonication applied during mixing the good/poor solvents (mesitylene/isopropanol) on the transformation of the cubic-shaped Sc3N@C80 crystals into dice-shaped crystals, we performed a comparison experiment by applying vigorous vibration, which may also induce perturbation on the mixing process. When a vigorous vibration from a mechanical shaker was applied during the rapid addition of isopropanol into the Sc3N@C80 solution in mesitylene, we found that mesitylene and isopropanol mixed only locally but not thoroughly in the vessel despite the vessel being shaken vigorously; as a result, non-uniform cubic-shaped Sc3N@C80 crystals with a much larger size distribution were obtained (see Fig. S3, ESI†). This is understandable because the Sc3N@C80 solutes distribute inhomogeneously around the local mixing of mesitylene and isopropanol. It is notable that, despite the perturbation by vigorous vibration, only Sc3N@C80 cubes were observed and no dice formed under the vigorous vibration conditions. This suggests that a perturbation induced by ultrasonication is necessary and more effective for dice formation because of the more homogeneous mixing of the binary solvents, and more importantly, the generation of gas microbubbles, which provide physical matrices for crystal nucleation and growth.17,27
Given that the role of ultrasonication on the formation of dice-shaped Sc3N@C80 crystals was confirmed, we next carried out a series of control experiments by varying the combination of good/poor solvents and their volume ratio in order to investigate the roles of good/poor solvents (mesitylene/isopropanol) on the formation of dice-shaped Sc3N@C80 crystals under ultrasonication conditions. First we selectively used analogous alkyl-substituted benzenes such as p-xylene and m-xylene as an alternative good solvent to dissolve Sc3N@C80. With the identical preparation procedure to that using mesitylene and ultrasonication, 1D micron-sized rods and micron-sized needle-like rods were obtained for p-xylene and m-xylene respectively, as we reported very recently (see Fig. S4, ESI†).32 Previously, using toluene or CS2 as the good solvent to dissolve Sc3N@C80, Wakahara et al. obtained either 1D Sc3N@C80 nanowhiskers when using toluene, or 1D nanowhiskers/2D tetragonal nanosheets by using CS2 (ultrasonication was not applied in both cases).30 Because the same solvent (isopropanol) was used as the poor solvent for interfacial precipitation in all cases, including our present study, the large diversity of the final Sc3N@C80 crystals must result from the nature of the good solvent. The dependence of the solvent nature (molecular geometry, solubility etc.) on the morphology of the nanostructures of fullerenes, especially C60, has been extensively investigated in the literature.11 It is commonly believed that the good solvent generally provides local cavities for crystal growth, guiding the self-crystallization of fullerene molecules into different morphologies.11 In this sense, the solvent geometry and solubility of fullerenes are two major factors determining the final morphology of fullerene crystals. In our present study, mesitylene has a higher molecular symmetry than p-xylene and m-xylene and Sc3N@C80 has a lower solubility in mesitylene, and presumably this is beneficial for the formation of homogeneous local mesitylene cavities, leading to the cocrystallization of Sc3N@C80 molecules with mesitylene. Thus homogeneous crystal growth in all axial directions is fulfilled and consequently 3D cubic-/dice-shaped Sc3N@C80 crystals form.
The good solvent determines the geometry of the Sc3N@C80 crystals, but does not seem to account for the formation of holes in each facet (i.e., dice-shape) of the Sc3N@C80 cubes, for which ultrasonication plays the determining role as discussed above. The effect of the poor solvent on the morphology of the Sc3N@C80 crystals was then studied further by substituting isopropanol with n-hexane and acetone. When n-hexane with a lower polarity than isopropanol was used as the poor solvent for precipitation, random Sc3N@C80 crystals, including both irregular polyhedra and pseudo-cubes with obscure edges and quite small and shallow holes in each facet, were obtained. On the other hand, when acetone with higher polarity replaced isopropanol, inhomogeneous dice-shaped Sc3N@C80 crystals with much larger holes in each facet were observed (see Fig. S5, ESI†). This phenomenon is probably because, similar to the case of C60,35 the relatively lower solubility of Sc3N@C80 in acetone than in n-hexane led to hindered Sc3N@C80 solute diffusion when using acetone, and thus crystals grew along the preferred direction. These results reveal that the polarity of the poor solvent is responsible for the size of the holes in each facet of the Sc3N@C80 dice.
Since mesitylene and isopropanol was determined to be the most favorable good/poor solvent combination for the formation of dice-shaped Sc3N@C80 crystals, the influence of their mixing ratio was studied next to gain deeper insight into the growth mechanism of the Sc3N@C80 crystals. Under the conditions of mesitylene
:
isopropanol = 1
:
3 (v/v), perfect Sc3N@C80 dice were obtained (see Fig. 1B). When the mixing ratio of mesitylene to isopropanol increased to 1
:
1 (v/v), the relative concentration of Sc3N@C80 in the mesitylene and isopropanol mixed solvents increased, resulting in the formation of more crystal nuclei and a sufficient solute supply, and consequently smaller cubic-shaped crystals instead of dice were obtained. On the other hand, the decrease of the mixing ratio of mesitylene to isopropanol to 1
:
5 (v/v) led to the coexistence of both dice and cubes with sharp edges and comparable size (see Fig. S6, ESI†). Since in this case the relative concentration of Sc3N@C80 in the mesitylene and isopropanol mixed solvents decreases, the size of the dice-shaped Sc3N@C80 crystals is obviously much larger compared to that obtained under the optimum conditions discussed above (mesitylene
:
isopropanol = 1
:
3 (v/v)). Interestingly, for some Sc3N@C80 dice, it was found that the holes were filled or attached with many small particles or cubes, and this can be regarded as an intermediate structure between the perfect dice and cube.
3.3. Growth mechanism and crystal structures of cubic- and dice-shaped Sc3N@C80 crystals
Based on the above systematic studies, a plausible growth mechanism of the cubic- and dice-shaped Sc3N@C80 crystals is proposed in Scheme 2. For Sc3N@C80 cubes, in the first step, local mesitylene cavities form immediately upon the addition of isopropanol, in which Sc3N@C80 molecules nucleate. Then crystal seeds start to grow by the deposition of Sc3N@C80 molecules onto the crystal nucleus via π–π stacking with the guidance of mesitylene solvent molecules, leading to the formation of Sc3N@C80 cubes. A similar mechanism has been reported for C70 cubes by Choi et al.17 When ultrasonication was switched on, upon mixing mesitylene and isopropanol, gas microbubbles are instantaneously generated, which on one hand promote the homogeneous formation of the local mesitylene cavities for Sc3N@C80 nucleation, but on the other hand hinder the diffusion of Sc3N@C80 solutes for crystal growth.4,24–27 Due to the slower diffusion of Sc3N@C80 solutes from mesitylene to isopropanol, Sc3N@C80 molecules would stack along the preferable 〈100〉 and 〈111〉 directions, followed by the principle of crystal growth under a shortage of the solute, leading to hole formation in each facet of the cube, i.e., a dice-shaped structure. It should be noted that, for the preparation of C70 cubes by Choi et al., ultrasonication along with rapidly mixing mesitylene and isopropanol was also applied, and the implementation of ultrasonication was found to be advantageous for improving the size homogeneity of the C70 cubes, but, similar to the case without applying ultrasonication, only cubes were obtained without dice-shaped crystals.17 The dramatic difference between Sc3N@C80 and C70 on the crystallization behavior under ultrasonication conditions suggests that the crystal growth of fullerenes with perturbation is sensitively dependent on the nature of the fullerenes, such as the cage size and charge transfer which takes place within endohedral fullerenes,19–23 and such a statement is to be further verified by other (endohedral) fullerenes.
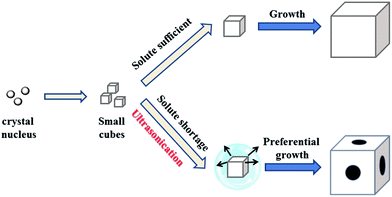 |
| Scheme 2 A schematic illustration of the growth process of cubic- and dice-shaped Sc3N@C80 crystals. Small cubes form after nucleation and then grow by preferential deposition. | |
Given that C60 and C70 crystals prepared by the LLIP method always contain solvent molecules in the crystal lattices,12–17,36 an open question arises: whether the dice-shaped Sc3N@C80 crystals contain the solvent molecules in the crystal lattices or not. Thermal gravimetric analysis (TGA) was then carried out under a 100 sccm N2 gas flow to investigate the decomposition process of the dice-shaped Sc3N@C80 crystals upon heating to over 800 °C, and two weight loss processes were clearly observed. In the first weight loss step (160–340 °C), a ∼15% weight loss was observed, which can be assigned to the removal of mesitylene solvent molecules. Since only one weight loss process was found in this temperature region, the coexistence of isopropanol solvent molecules can be ruled out. In the second weight loss process (>800 °C), the weight loss is attributed to the decomposition of dice-shaped Sc3N@C80 crystals (see Fig. S7, ESI†). The TGA results reveal that the dice-shaped Sc3N@C80 crystals contain mesitylene solvent molecules with the molar ratio of mesitylene to Sc3N@C80 being ∼1.6
:
1. Besides, the existence of mesitylene solvent molecules in both the dice- and cubic-shaped Sc3N@C80 crystals is further confirmed by FTIR measurements (see Fig. S8, ESI†).
The crystal structures of the cubic- and dice-shaped Sc3N@C80 crystals were examined by powder X-ray diffraction (XRD, Fig. 2) in combination with the electron diffraction pattern (Fig. 3). In the XRD pattern of the Sc3N@C80 cubes (Fig. 2(I)), two intense diffraction peaks with d-spacings of 10.8 Å and 5.41 Å respectively can be indexed as the (100) and (200) planes, suggesting that the Sc3N@C80 cubes lie on the Si substrate through {100}-series planes. There are several other weak diffraction peaks with d-spacings of 6.24, 4.85, 4.43, 3.62, 3.42 and 3.27 Å corresponding to the (111), (210), (211), (300), (310) and (311) planes, respectively (see S9, ESI†). Such a diffraction pattern perfectly matches to the simple cubic structure (a = 10.8 Å). A high-resolution transmission electron microscopy (HR-TEM) image of a Sc3N@C80 cube (inset A of Fig. 3) shows a clear lattice image with the distance between two adjacent planes of 10.8 Å, which could be assigned to the d-spacing of the (100) crystalline plane as is clearly observed in the corresponding selected-area electron diffraction (SAED) pattern (inset B of Fig. 3). These data coincide with that obtained from the XRD characterization, confirming the simple cubic structure with a = 10.8 Å for the Sc3N@C80 cubes.
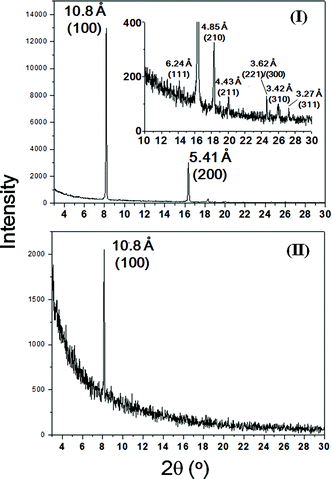 |
| Fig. 2 XRD patterns of cubic- (I) and dice-shaped (II) Sc3N@C80 crystals. Inset: magnified XRD pattern in the region of 2θ = 10–30°. | |
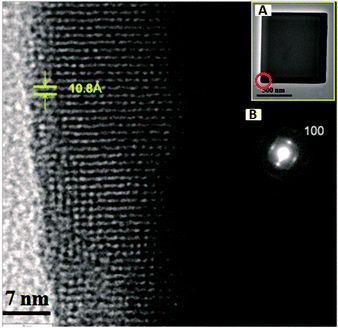 |
| Fig. 3 High-resolution TEM (HR-TEM) image of a cubic-shaped Sc3N@C80 crystal. Insets: (A) TEM image of a single Sc3N@C80 cube. The red ring marks the site of electron diffraction; (B) selected area electron diffraction (SAED) pattern. | |
For the dice-shaped Sc3N@C80 crystals, only one intense diffraction peak with a d-spacing of 10.8 Å was observed in the XRD pattern (Fig. 2(II)), which can be indexed as the (100) plane as well. Similar to the case of the Sc3N@C80 cubes, the dominating appearance of only (100) plane diffraction suggests that the dice-shaped Sc3N@C80 crystals contact with Si substrate through {100}-series planes as well, while the (200) plane diffraction was not detected. This might be ascribed to the weak crystallinity of the dice-shaped Sc3N@C80 crystals. In fact, the sample with weak crystallinity suffers amorphization after high-energy electron beam bombarding, thus an attempt to measure the HR-TEM image and SAED pattern of the dice-shaped Sc3N@C80 crystals failed.
3.4. Application of cubic- and dice-shaped Sc3N@C80 crystals as Pt catalyst supports for the methanol oxidation reaction (MOR)
To our knowledge, the catalytic properties of endohedral fullerenes have never been studied. The unprecedented dice shape of the Sc3N@C80 crystals with the hole structure stimulated us to investigate their application as a Pt catalyst support for the methanol oxidation reaction (MOR),2–4,37,38 and its catalytic activity is compared with that of the cubic-shaped Sc3N@C80 crystals so as to understand the structure–property relationship of the Sc3N@C80 crystals. Sc3N@C80 dice were first deposited onto an indium tin oxide (ITO) film electrode by an electrophoretic deposition method,32 and then ∼16 μg platinum (Pt) was loaded onto the Sc3N@C80 crystal surface (see Experimental details).3,4Fig. 4(I) shows the cyclic voltammogram (CV) of the Pt/Sc3N@C80 dice/ITO electrode in 0.5 M H2SO4 solution, which includes also those of the Pt/Sc3N@C80 cubes/ITO and Pt/ITO electrodes for comparison. All three CV curves show obvious redox peaks associated with hydrogen adsorption/desorption below 0.15 V (vs. Ag/AgCl). The electrochemical active surface area was calculated by measuring the charge (QH) associated with the adsorbed hydrogen monolayer in the potential region of −0.2 to 0.15 V, which is 5.93, 3.56 and 2.43 m2 g−1 for the Pt/Sc3N@C80 dice/ITO, Pt/Sc3N@C80 cubes/ITO and Pt/ITO electrodes, respectively (see Experimental details). It is noteworthy that the electrochemical active surface area of the Pt/Sc3N@C80 dice/ITO electrode is ca. 1.7 and 2.4 times larger than those of the Pt/Sc3N@C80 cubes/ITO and Pt/ITO electrodes, respectively, suggesting that the dispersion of the Pt catalyst nanoparticles is largely improved upon applying Sc3N@C80 crystals as catalyst supports.
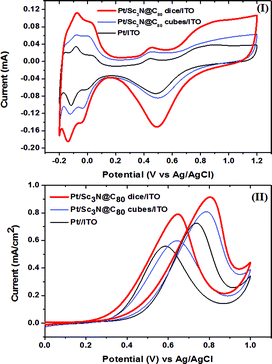 |
| Fig. 4 (I) Cyclic voltammograms of the Pt/Sc3N@C80 dice/ITO, Pt/Sc3N@C80 cubes/ITO and Pt/ITO electrodes recorded in 0.5 M H2SO4 with a scan rate of 100 mV s−1; (II) normalized cyclic voltammograms of the Pt/Sc3N@C80 dice/ITO, Pt/Sc3N@C80 cubes/ITO and Pt/ITO electrodes in 2.0 M methanol + 1.0 M H2SO4 solutions with a scan rate of 20 mV s−1. The curves are normalized to the electrochemical active surface area and Pt loading (∼16 μg). | |
The MOR curves of the three modified ITO electrodes normalized to the electrochemical active surface area and Pt loading, which were measured in 2.0 M methanol and 1.0 M H2SO4 solution at room temperature with a scan rate of 20 mV s−1, are compared in Fig. 4(II). The Pt/Sc3N@C80 dice/ITO and Pt/Sc3N@C80 cubes/ITO electrodes show onset potentials of 0.15 V and 0.17 V, respectively, which exhibit a negative shift of about 0.09 and 0.07 V over that of the Pt/ITO electrode (0.24 V). The oxidation peak currents observed for the Pt/Sc3N@C80 dice/ITO and Pt/Sc3N@C80 cubes/ITO electrodes are both higher than that for the Pt/ITO electrode (see Fig. 4(II)). Besides, chronoamperometric measurements performed at 0.81 V confirmed the highest methanol oxidation current for the Pt/Sc3N@C80 dice/ITO electrode, and that the catalytic activity follows the order of Pt/Sc3N@C80 dice/ITO > Pt/Sc3N@C80 cubes/ITO > Pt/ITO (see Fig. S9, ESI†). A higher electrocatalytic stability of the Pt/Sc3N@C80 dice/ITO electrode over that of the Pt/Sc3N@C80 cubes/ITO electrode is observed despite the fast current decay due to the poisoning of the catalyst by the intermediate species formed during the methanol oxidation reaction.38 The improved MOR activity of Pt/Sc3N@C80 dice/ITO compared to Pt/Sc3N@C80 cubes/ITO is presumably due to the larger surface area of the Sc3N@C80 dice resulting from the unique dice (hole) structure.4
4. Conclusions
In summary, for the first time, 3D nanostructures of Sc3N@C80, including both cubic-shaped and unprecedented dice-shaped crystals, have been successfully prepared via a modified LLIP method integrating ultrasonication. By simply switching ultrasonication on/off upon mixing the good/poor solvents (mesitylene/isopropanol), the dice- and cubic-shaped Sc3N@C80 crystals were selectively prepared, and their size can be readily controlled by varying the concentration of the starting Sc3N@C80 solution in mesitylene. Based on a systematic study on the effects of ultrasonication, good/poor solvents and their mixing ratio on the morphology of the Sc3N@C80 crystals, a plausible growth mechanism of the cubic- and dice-shaped Sc3N@C80 crystals was proposed, highlighting the formation of local mesitylene cavities which lead to the nucleation and growth of Sc3N@C80 molecules, while the formation of the dice structure. i.e., a hole in each facet of the cube, was primarily due to the hindered diffusion of Sc3N@C80 solutes by ultrasonication-generated gas microbubbles. The crystal structure of the Sc3N@C80 cubes was determined to feature a simple cubic unit cell with a lattice constant of a = 10.8 Å. Finally, the dice-shaped Sc3N@C80 crystals were applied as a Pt catalyst support for the MOR, revealing the improved MOR activity compared to cubic-shaped Sc3N@C80 crystals due to their larger surface area resulting from the dice (hole) structure. As the first success of the facile preparation of 3D nanostructures of endohedral fullerenes, our study provides new insight into the crystallization behavior of fullerenes as well as their potential application in direct methanol fuel cells.
Acknowledgements
We are grateful to valuable discussions with Professor Suhua Wang (Institute of Intelligent Machines, Chinese Academy of Sciences). This work was partially supported by the National Basic Research Program of China (2010CB923300, 2011CB921400) and the National Natural Science Foundation of China (no. 21132007, 21371164).
Notes and references
- W. Krätschmer, L. D. Lamb, K. Fostiropoulos and D. R. Huffman, Nature, 1990, 347, 354 CrossRef
.
- B. S. Sherigara, W. Kutner and F. D'Souza, Electroanalysis, 2003, 15, 753 CrossRef CAS
.
- K. Vinodgopal, M. Haria, D. Meisel and P. Kamat, Nano Lett., 2004, 4, 415 CrossRef CAS
.
- Y. Zhang, L. Jiang, H. Li, L. Z. Fan, W. P. Hu, C. R. Wang, Y. F. Li and S. H. Yang, Chem. - Eur. J., 2011, 17, 4921 CrossRef CAS PubMed
.
- Y. Zhang, W. Liu, L. Jiang, L. Z. Fan, C. Wang, W. P. Hu, H. Zhong, Y. F. Li and S. H. Yang, J. Mater. Chem., 2010, 20, 953 RSC
.
- L. Wang, B. B. Liu, D. Liu, M. G. Yao, Y. Y. Hou, S. D. Yu, T. Cui, D. M. Li, G. T. Zou, A. Iwasiewicz and B. Sundqvist, Adv. Mater., 2006, 18, 1883 CrossRef CAS
.
- X. D. Shi, A. R. Kortan, J. M. Williams, A. M. Kini, B. M. Savall and P. M. Chaikin, Phys. Rev. Lett., 1992, 68, 827 CrossRef CAS
.
- J. F. Geng, I. A. Solov'yov, W. Z. Zhou, A. V. Solov'yov and B. F. G. Johnson, J. Phys. Chem. C, 2009, 113, 6390 CAS
.
- M. G. Yao, B. M. Andersson, P. Stenmark, B. Sundqvist, B. B. Liu and T. Wågberg, Carbon, 2009, 47, 1181 CrossRef CAS PubMed
.
- L. Wang, B. B. Liu, D. D. Liu, M. G. Yao, S. D. Yu, Y. Y. Hou, B. Zou, T. Cui, G. T. Zou, B. Sundqvist, Z. J. Luo, H. Li, Y. C. Li, J. Liu, S. J. Chen, G. R. Wang and Y. C. Liu, Appl. Phys. Lett., 2007, 91, 103 Search PubMed
.
- C. Park, H. J. Song and H. C. Choi, Chem. Commun., 2009, 4803 RSC
.
- K. Miyazawa, Y. Kuwasaki, A. Obayashi and M. Kuwabara, J. Mater. Res., 2002, 17, 83 CrossRef CAS
.
- Y. Jin, R. J. Curry, J. Sloan, R. A. Hatton, L. C. Chong, N. Blanchard, S. V. Tolojan, W. H. Kroto and S. R. P. Silva, J. Mater. Chem., 2006, 16, 3715 RSC
.
- K. Miyazawa, J. Minato, T. Yoshii, M. Fujino and T. Suga, J. Mater. Res., 2005, 20, 688 CrossRef CAS
.
- M. Sathish and K. Miyazawa, J. Am. Chem. Soc., 2007, 129, 13816 CrossRef CAS PubMed
.
- M. Sathish, K. Miyazawa, J. P. Hill and K. Ariga, J. Am. Chem. Soc., 2009, 131, 6372 CrossRef CAS PubMed
.
- C. Park, E. Yoon, M. Kawano, T. Joo and H. C. Choi, Angew. Chem., Int. Ed., 2010, 49, 9670 CrossRef CAS PubMed
.
- M. G. Yao, X. H. Fan, D. D. Liu, B. B. Liu and T. Wågberg, Carbon, 2012, 50, 209 CrossRef CAS PubMed
.
- A. A. Popov, S. F. Yang and L. Dunsch, Chem. Rev., 2013, 113, 5989 CrossRef CAS PubMed
.
- T. S. Wang and C. R. Wang, Acc. Chem. Res., 2014, 47, 450 CrossRef CAS PubMed
.
- X. Lu, L. Feng, T. Akasaka and S. Nagase, Chem. Soc. Rev., 2012, 41, 7723 RSC
.
- S. F. Yang, Curr. Org. Chem., 2012, 16, 1079 CrossRef CAS
.
- S. F. Yang, F. P. Liu, C. B. Chen, M. Z. Jiao and T. Wei, Chem. Commun., 2011, 47, 11822 RSC
.
- N. Lyczko, F. Espitalier, O. Louisnard and J. Schwartzentruber, Chem. Eng. J., 2002, 86, 233 CrossRef CAS
.
- H. Li, J. K. Wang, Y. Bao, Z. C. Guo and M. Y. Zhang, J. Cryst. Growth, 2003, 247, 192 CrossRef CAS
.
- Z. Guo, M. Zhang, H. Li, J. Wang and E. Kougoulos, J. Cryst. Growth, 2005, 273, 555 CrossRef CAS PubMed
.
- B. W. Zeiger and K. S. Suslick, J. Am. Chem. Soc., 2011, 133, 14530 CrossRef CAS PubMed
.
- C. J. Li, Y. G. Guo, B. S. Li, C. R. Wang, L. J. Wan and C. L. Bai, Adv. Mater., 2005, 17, 71 CrossRef CAS
.
- T. Tsuchiya, R. Kumashiro, K. Tanigaki, Y. Matsunaga, M. O. Ishitsuka, T. Wakahara, Y. Maeda, Y. Takano, M. Aoyagi, T. Akasaka, M. T. Liu, T. Kato, K. Suenaga, J. S. Jeong, S. Iijima, F. Kimura, T. Kimura and S. Nagase, J. Am. Chem. Soc., 2008, 130, 450 CrossRef CAS PubMed
.
- T. Wakahara, Y. Nemoto, M. Xu, K. Miyazawa and D. Fujita, Carbon, 2010, 48, 3359 CrossRef CAS PubMed
.
- Y. Xu, C. C. He, F. P. Liu, M. Z. Jiao and S. F. Yang, J. Mater. Chem., 2011, 21, 13538 RSC
.
- Y. Xu, J. H. Guo, T. Wei, X. Chen, Q. Yang and S. F. Yang, Nanoscale, 2013, 5, 1993 RSC
.
- S. F. Yang, C. B. Chen, A. A. Popov, W. F. Zhang, F. P. Liu and L. Dunsch, Chem. Commun., 2009, 6391 RSC
.
- A. Pozio, M. D. Francesco, A. Cemmi, F. Cardellini and L. Giorgi, J. Power Sources, 2002, 105, 13 CrossRef CAS
.
- R. S. Ruoff, D. S. Tse, R. Malhotra and D. C. Lorents, J. Phys. Chem., 1993, 97, 3379 CrossRef CAS
.
- J. F. Geng, W. Z. Zhou, P. Skelton, W. B. Yue, I. A. Kinloch, A. H. Windle and B. F. G. Johnson, J. Am. Chem. Soc., 2008, 130, 2527 CrossRef CAS PubMed
.
- H. R. Barzegar, G. Hu, C. Larsen, X. Jia, L. Edman and T. Wågberg, Carbon, 2014, 73, 34 CrossRef CAS PubMed
.
- L. Gao, L. Ding and L. Fan, Electrochim. Acta, 2013, 106, 159 CrossRef CAS PubMed
.
Footnotes |
† Electronic supplementary information (ESI) available: Size distributions of cubic- and dice-shaped Sc3N@C80 crystals, SEM images of Sc3N@C80 crystals obtained under other conditions, TGA curve of Sc3N@C80 dice, FTIR spectra of Sc3N@C80 dice and cubes, detailed indexing data of Sc3N@C80 cubes, chronoamperometric curves, etc. See DOI: 10.1039/c4mh00037d |
‡ These authors contributed equally to this work. |
|
This journal is © The Royal Society of Chemistry 2014 |
Click here to see how this site uses Cookies. View our privacy policy here.