DOI:
10.1039/C4MH00066H
(Communication)
Mater. Horiz., 2014,
1, 529-534
Fluorescent quantum dots derived from PEDOT and their applications in optical imaging and sensing†
Received
28th April 2014
, Accepted 16th May 2014
First published on 16th May 2014
Abstract
We herein demonstrate a facile and general approach to synthesize novel polymer quantum dots (QDs) derived from non-fluorescent poly(3,4-ethylenedioxythiophene) (PEDOT). Such a PEDOT-QD is of molecular size and exhibits excellent photostability, two-photon excitation properties, and biocompatibility. Furthermore, we demonstrate the use of this new type of fluorescent reporter for cellular imaging and sensitive and specific optical detection of mercury ions. The synthetic route demonstrated here can be easily modified (e.g., using different polymer precursors, ionic liquids for polymerization, and solvents for exfoliation) to produce different types of polymer QDs with various interesting properties.
Conceptual insights
Due to the intrinsic limitations suffered by the current fluorophores (e.g., fluorescent proteins, organic dyes, and semiconductor quantum dots), seeking better fluorescent reporters which are photostable, biocompatible, and of molecular size is an ongoing and critical effort for the areas of bioimaging and optical sensing. We herein report a facile and general approach to synthesize novel polymer-based quantum dots (QDs). Furthermore, as the proof-of-concept demonstration, such a new type of QD is used for cellular imaging and sensitive optical detection of mercury ions.
|
Introduction
Lighting up the perplexing and dynamic biological processes conventionally relies on organic fluorophores (fluorescent proteins and dyes). But they intrinsically suffer from rapid photo-bleaching which makes long-term real-time imaging difficult. Semiconductor quantum dots (semi-QDs) with high photo-stability have thus been regarded as alternative fluorescent reporters.1–3 These semi-QDs, however, exhibit several innate limitations, including cytotoxicity due to leaching of heavy metal ions, blinking which makes single-molecule tracking challenging and too-large a size which alters the functions/dynamics of the targeted molecules and creates non-physiological clusters while interacting with multiple target molecules. More recently, graphene quantum dots (GQDs) have demonstrated the potential for real-time molecular imaging in live cells owing to their good photostability, small size, and biocompatibility.4,5 Both semi-QDs and GQDs have demonstrated potential for sensitive detection of biomolecules or chemicals.6–9 But these synthetic fluorophores are still not perfectly photo-stable and usually require nontrivial synthesis or purification processes.
It has been recently demonstrated that QDs derived from fluorescent semiconducting polymers (pQDs) show super photo-stability, together with other merits including high brightness, tunable photoluminescence properties, non-blinking properties, and biocompatibility.10–15 Nevertheless, these polymer nanoparticles are sometimes large in size (up to 100 nm). And the synthetic routes are usually tedious. Herein, we report a facile approach to synthesize a new type of pQD derived from non-fluorescent poly(3,4-ethylenedioxythiophene) (PEDOT), which exhibit molecular size, remarkable photo-stability, two-photon excitation properties, and good biocompatibility. The optical properties of PEDOT-QDs are consistent with the theoretical calculations. Furthermore, the applications of this novel PEDOT-QD for cellular imaging and optical sensing of mercury ions (Hg2+) are demonstrated.
Experimental section
Preparation of the fibrous PEDOT film
The 3,4-ethylenedioxythiophene (EDOT) monomer, ionic liquid (1-n-butyl-3-methylimidazolium tetrafluoroborate – BMIMBF4) and N,N-dimethylformamide (DMF) were purchased from Sigma Aldrich. With 0.1 M EDOT monomer in the ionic liquid, the PEDOT film was polymerized on an ITO working electrode held at a constant potential of 1 V for 3 h. An electrochemical workstation (CHI 660D) was used for polymerization, with a three-electrode configuration consisting of a platinum plate counter electrode, a silver wire reference electrode and an ITO working electrode. Thorough washing to eliminate excess ionic liquid and unreacted monomers and overnight drying in a vacuum oven at 27 °C followed polymerization.
Preparation of PEDOT-QDs
The polymer film was then gently scraped off the ITO electrode and suspended in DMF solvent (1 mg mL−1), followed by ultrasonication (Branson 2510; 1.1 A, 230 W) at 27 °C for 4 h. After centrifugation at 10
000 rpm for 30 min, the supernatant was collected. This was repeated 5 times to obtain the dispersion of PEDOT-QDs in DMF, which was subsequently filtered using a WHATMAN 0.2 μm PTFE filter. To re-suspend PEDOT-QDs in distilled water, DMF was completely extracted using rotary evaporation at 80 °C, and distilled water was added to the dried QD aggregates. The solution was then ultra-filtered (molecular weight cut-off of 3 kDa) to remove the aggregates. PEDOT-QD suspensions in both DMF and water are highly stable (lasting for months without apparent aggregation).
Characterization
The samples were examined by field emission scanning electron microscopy (FESEM, JMS-6700F), atomic force microscopy (MFP-3D AFM microscope, Asylum research), Raman spectroscopy (WITec CRM200 using 633 nm laser), high-resolution transmission electron microscopy (HRTEM, JEOL 2010), X-ray photoelectron spectroscopy (ESCALAB MK-II), X-ray diffraction (Bruker D8 Avance diffractometer using Cu Kα radiation) and Fourier transform infrared spectroscopy (Perkin Elmer FTIR Spectrum GX 69233). The UV-vis absorption and photoluminescence performance of QDs was characterized by using a UV-2450 spectrophotometer (Shimadzu), and an LS-55 fluorescence spectrometer (PerkinElmer), respectively.
Cell imaging
The PC12 cells (American Type Culture Collection) were cultured in Dulbecco's modified Eagle's medium (DMEM, Gibco) supplemented with 10% (v/v) fetal bovine serum (Gibco) and 1% penicillin–streptomycin, at 37 °C under a humidified atmosphere containing 5% CO2 and 95% air. Cells were incubated with 76.6 μg mL−1 blue PEDOT-QDs (dispersed in water) or 5.5 μg mL−1 green PEDOT-QDs (dispersed in DMF) for 24 h before imaging with a confocal laser scanning microscope (LSM 510 Meta, Carl Zeiss GMbH, Germany). The blue (or green) QDs were excited at 405 (or 488) nm and detected with an emission filter <480 nm (or >520 nm).
Optical detection of Hg2+ ions
The photoluminescence of PEDOT-QDs (1 mg mL−1 in DMF was diluted 5 times in distilled water) was measured before and after adding various metal salts at defined concentrations, including mercury(II) perchlorate, lead(II) nitrate, zinc nitrate hexahydrate, cadmium chloride, cesium chloride, magnesium sulphate, cobalt nitrate hexahydrate, nickel nitrate hexahydrate, manganese nitrate tetrahydrate, gold(III) chloride trihydrate and potassium chloride.
Results & discussion
Synthesis and morphology of PEDOT-QDs
As schematically illustrated (Scheme 1), EDOT monomers are electro-polymerized in an ionic liquid (BMIMBF4). Field-effect scanning electron microscopy (FESEM) reveals that a film of polymerized EDOTs (PEDOT) consequently forms on the ITO electrode (Fig. 1a). On the film, ultra-long wires and roots of outgrowing wires can be observed (bright dots and lines in Fig. 1a). A closer inspection shows that the film consists of dense interwoven fibers (inset in Fig. 1a). The fibrous PEDOT film is then sonicated in DMF solvent, producing a yellow solution with well-dispersed PEDOT-QDs exfoliated from the film (Scheme 1).
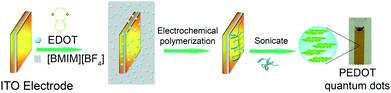 |
| Scheme 1 Schematic illustration for the preparation of PEDOT-QDs. | |
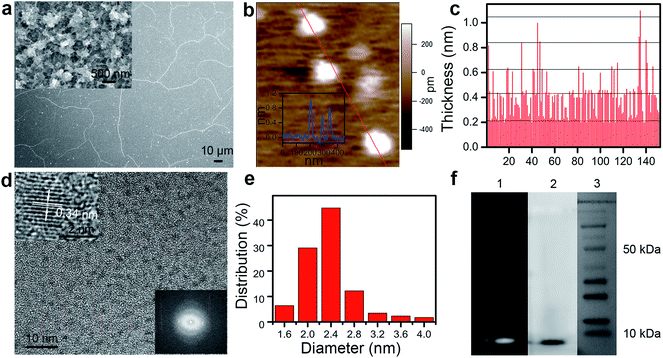 |
| Fig. 1 QDs exfoliated from the fibrous electropolymerized PEDOT film are small and uniform in size. (a) FESEM image of PEDOT. The inset shows the film surface with a higher-resolution. (b) AFM image of PEDOT-QDs. The inset shows the height profile along the indicated line. (c) The height (thickness) of 152 individual QDs. The indicative horizontal lines are 0.21 nm apart. (d) HRTEM image of PEDOT-QDs. The upper inset shows a single QD with the crystal lattice resolved. The lower inset shows the FTT diffraction pattern. (e) Diameter distribution of 172 QDs. (f) The gel electrophoresis of PEDOT-QDs (1 – fluorescent image under UV; 2 – bright field image) and protein markers (3). | |
As observed from atomic force microscopy (AFM), the PEDOT-QDs exhibit quantized thickness (Fig. 1b and c) with an average thickness of ∼0.40 nm (±0.17, n = 152) and a quantal step of ∼0.21 nm (corresponding to the thickness of the single PEDOT chain16). This suggests that PEDOT-QDs range from single to a few layers. High-resolution transmission electron microscopy (HRTEM) shows that PEDOT-QDs are uniform in diameter (∼2.3 ± 0.36 nm, n = 172) (Fig. 1d and e). The diffraction pattern indicates that the QDs possess a certain degree of crystallinity (lower inset in Fig. 1d). Indeed, a well-defined crystal lattice can be resolved from some QDs under high-resolution TEM (upper inset in Fig. 1d). Consistent with a previous study,17 the observed lattice spacing of 3.4 Å corresponds to the characteristic face-to-face distance (010) of the rigid PEDOT polymer, similar to graphite. Therefore, it appears that the disc-like QDs are mechanically chopped by sonication from the crystalline PEDOT polymer chain. Fig. 1f shows the gel-electrophoresis result of the PEDOT-QD sample in comparison with protein markers. The bright field and fluorescence gel images show a narrow band of PEDOT-QDs indicating that they have a uniform size distribution and a molecular weight <10 kDa which is comparable to a single small protein.
Spectroscopic characterization of PEDOT-QDs
X-ray photoelectron spectroscopy (XPS) spectra of both the PEDOT-film and PEDOT-QDs show the characteristic S2s (228 eV), S2p (163 eV), C1s (283 eV) and O1s (531 eV) peaks from PEDOT (Fig. 2a).18,19 The spectrum of the PEDOT-film also exhibits B1s (193 eV), N1s (401 eV), and F1s (685 eV) peaks resulted from the remaining ionic liquid (BMIMBF4) from the polymerization process.20 The B1s and F1s peaks diminish in the XPS spectrum of PEDOT-QDs while the N1s peak is significantly reduced. Deconvolution of the high resolution XPS spectra of C1s (Fig. 2b) and S2p (Fig. 2c) indicates the existence of the (C
C) sp2, (C–C) sp3, C–S, C–O, S2p3/2, and S2p1/2 peaks characteristic of PEDOT, and small C–N and C
N peaks characteristic of [BMIM]+.9,21 The small amount of association of the nitrogen-containing ionic liquid is also confirmed by the high-resolution N1s spectrum (Fig. 2b, inset). In addition, Raman spectra of both the PEDOT film and PEDOT-QDs are similar and in agreement with the characteristic spectrum of PEDOT22 (Fig. 2d). Consistent with XPS characterization, Fourier transform infrared spectra (FTIR) of PEDOT-QDs (Fig. 2e) in both water and DMF show the characteristic peaks from PEDOT at 1638 cm−1 (C
C stretching vibration), 1385 cm−1 (C
C stretching of the thiophene ring), 1124 cm−1 (polythiophene absorption), 1084 cm−1(C–O stretching), and 929 cm−1 (C–S stretching).23 And the peaks from BMIMBF4 are also observed at 1576 cm−1 and 1459 cm−1 (both from imidazole ring adsorption).24 The d020 peak in the X-ray diffraction (XRD) spectrum of the PEDOT film or PEDOT-QDs corresponds to the face-to-face packing between the PEDOT chains with a distance of 0.34 nm
17 (Fig. 2f). This correlates well with the HRTEM observation shown in Fig. 1d.
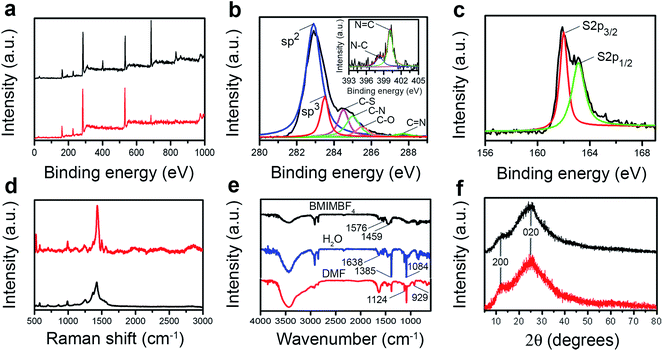 |
| Fig. 2 Spectroscopic characterization. (a) XPS spectra of the PEDOT film (black) and PEDOT-QDs (red). (b) High-resolution C1s peak in the XPS spectrum of PEDOT QDs. The inset shows the N1s peak. (c) High-resolution S2p peak in the XPS spectrum of PEDOT QDs. (d) Raman spectra of the PEDOT film (black) and PEDOT-QDs (red). (e) FTIR spectra of BMIMBF4 (black), PEDOT-QDs (blue – in water; red – in DMF). (f) XRD spectra of the PEDOT film (black) and PEDOT QDs (red). | |
A simple method for synthesis of a new type of polymer QD is demonstrated. In addition to mechanical stress introduced by sonication, it is conceivable that exfoliation of QDs from PEDOT fibers is also facilitated by the intercalation of BMIMBF4. The imidazole ring of BMIM+ may interact with PEDOT via π–π and electrostatic interactions. In support of this, it has been previously reported that ionic liquids can intercalate into PEDOT films and cause swelling.25 Furthermore, it seems that the DMF solvent also plays an important role in exfoliation and stabilizing the exfoliated QDs. In comparison, high-yield exfoliation of QDs does not occur in other solvents (specifically ethanol, water, and acetonitrile).
Optical characterization of PEDOT-QDs
The as-prepared QDs are well dispersed in DMF. They can be extracted using rotary evaporation and re-suspended in water. The absorption spectra of these two suspensions are shown in Fig. 3a. The water suspension of QDs efficiently absorbs UV light while the DMF suspension extends its adsorption to the visible light region. The excitation-dependent emission spectra of both QD suspensions indicate the maximum emission peaks from PEDOT-QDs in DMF and in water at 533 nm (excited at 460 nm) and 450 nm (excited at 360 nm), respectively (Fig. 3b and c). Clearly, the optical properties of PEDOT-QDs depend on their interaction with the solvent. And the excitation dependence suggests the heterogeneity in size and properties of the synthesized QDs. In comparison, EDOT monomers suspended in DMF or water do not show apparent absorption and emission, suggesting that the observed optical properties arise from the confined conjugation of these monomers.
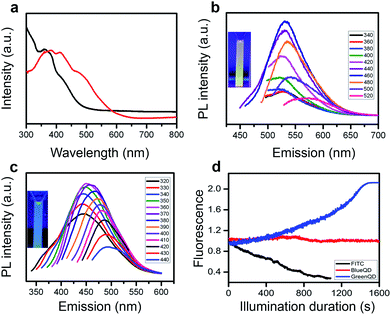 |
| Fig. 3 Optical characteristics of PEDOT-QDs. (a) UV-vis adsorption spectrum of PEDOT QDs in water (black) and DMF (red). (b and c) PL spectra of QDs in DMF and water with different excitation wavelengths. The insets show the images of the QD suspensions under UV. (d) The photobleaching behavior showing normalized fluorescence of the green QDs (in DMF), blue QDs (in water), and FITC under continuous confocal imaging. | |
To reveal the absorption and emission mechanism, density functional theory (DFT) and time-dependent DFT (TDDFT) calculations are performed. The DFT calculations on EDOT trimers (using it as a model compound for PEDOT-QDs) suggest that they can assume three possible configurations (isomers 1–3 as shown in Fig. S1, ESI†). Isomer 3, which has a high dipole moment, is most thermodynamically stable in water by forming multiple hydrogen bonds with water molecules, but not in the aprotic DMF solvent. Therefore, we speculate that the DMF suspension contains isomers 1 and 2 while the water suspension only has isomer 3. Theoretical calculations show that the absorption peaks of isomers 1 and 2 in the dielectric environment of DMF are 411 and 390 nm, respectively (Table 1, ESI†). These values agree well with the experimentally observed peaks at ∼412 nm and ∼384 nm (Fig. 3a). The absorption peak of isomer 3 in water is predicted to be 362 nm which is also close to the experimental observation (∼360 nm as shown in Fig. 3a). Similar to the observed emission peak of PEDOT-QDs in DMF (∼533 nm), the emission peaks of isomers 1 and 2 are calculated to be 515 and 523 nm, respectively (Table 2, ESI†). Isomer 3 in water is predicted to emit at 477 nm, consistent with the observed emission peak of PEDOT-QDs in water (∼450 nm).
PEDOT is natively non-fluorescent due to the small optical band-gap (1.6 eV) which leads to rapid electron–hole recombination.26 As shown by our theoretical studies (Table 1, ESI†), the resulting photoluminescence properties of the nano-sized PEDOT-QDs arise from the band-gap widening due to quantum confinement. The PEDOT-QDs exhibit two excitation peaks (Fig. S2, ESI†) resulting from σ to π* and π to π* transitions (Fig. S3, ESI†).
Organic fluorophores, such as FITC, bleach quickly under confocal imaging (Fig. 3d). Remarkably, no bleaching was observed from both PEDOT-QDs after long-term imaging. Intriguingly, the green QDs (in DMF) significantly increase the fluorescence intensity under illumination. Furthermore, the photo-bleaching studies performed over 2 hours using a spectro-fluorometer indicate a similar behavior except that the PL of blue QDs decreases slightly (Fig. S4, ESI†). It is conceivable that laser annealing further improves the crystalline structure of PEDOT-QDs.27–29 The green QDs in DMF and blue QDs in water have a quantum yield of 13% (using Rhodamine 6G as the reference) and 4% (using quinine sulfate as the reference), respectively.
Furthermore, the two-photon excited photoluminescence (TPPL) of our PEDOT-QDs was examined. TPPL offers unique advantages over the conventional one-photon excitation, including larger penetration depth into the tissue and higher signal-to-noise ratio benefiting from the longer excitation wavelength and nonlinear absorption process.30 The PEDOT-QDs exhibit strong emission under two-photon excitation (800 nm, even at a relatively low intensity of 6.7 GW cm−2) (Fig. S5a, ESI†). The two-photon excitation process is clearly evidenced by the nearly quadratic excitation intensity dependence of the PL signals in the corresponding log–log plot of the PL signals versus excitation intensity31 (Fig. S5b, ESI†). An outstanding photostability under two-photon excitation is demonstrated with a retention of >90% of the initial emission after ∼50 min, when operated at a pumping intensity of 20.1 GW cm−2 (Fig. S5c, ESI†). The molecular size and remarkable photo-stability of PEDOT-QDs under both single and two-photon excitation promise their applications for bio-imaging. Furthermore, a high concentration (150 μg mL−1) of PEDOT-QDs is not able to induce significant cytotoxicity, indicating good biocompatibility of these QDs (Fig. S6, ESI†).
Cellular imaging using PEDOT-QDs
Interestingly, diluting the DMF-suspended QDs in water (95% dilution), their green fluorescence properties preserve suggesting the persistent association between DMF molecules and PEDOT-QDs (Fig. S7, ESI†). As the proof-of-concept demonstration for cellular imaging, we incubated PC12 cells with blue water-suspended QDs (76.6 μg mL−1) and DMF-associated green QDs diluted in cell culture medium (5.5 μg mL−1) for 24 h. As shown in Fig. 4, both types of PEDOT-QDs are taken up into the cells and remain fluorescent. Some QDs are located in the early endosomes which are specifically labeled by the fluorescent early endosome marker (mRFP-Rab5).
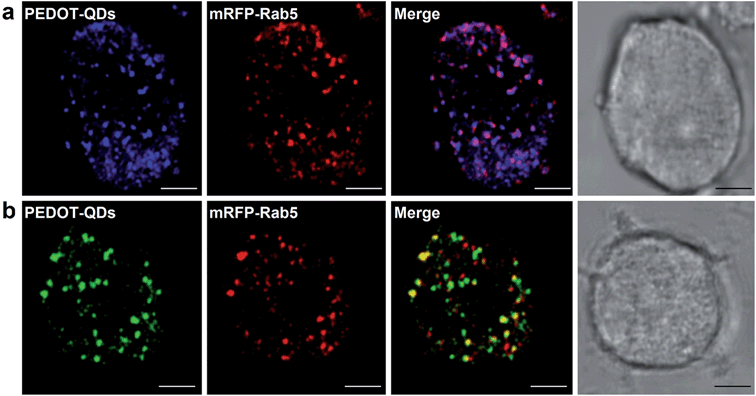 |
| Fig. 4 Confocal imaging of PC12 cells after incubation with blue (a) or green (b) PEDOT-QDs. Confocal images with staining of QDs (column 1) and endosome marker mRFP-Rab5 (column 2). Column 3 is the merged image of columns 1 and 2. Column 4 shows the bright-field image of the cells. Scale bar = 5 μm. | |
Optical detection of mercury ions (Hg2+) using PEDOT-QDs
Similar to other synthetic quantum dots,8,9,32 the small PEDOT-QDs promise wide applications in optical sensing. As the proof-of-concept demonstration, we show here that the photoluminescence (PL) of PEDOT-QDs can be significantly quenched by 57% upon addition of 100 μM Hg2+ ions whereas the PEDOT-QDs are not obviously responsive to other metal ions (Pb2+, Zn2+, Cd2+, Cs+, Mg2+, Co2+, Ni2+, Mn2+, K+ and Au3+) (Fig. 5a). PL quenching by Hg2+ is dose-dependent (Fig. 5b). And as shown in Fig. 5c, the extrapolated lower theoretical detection limit is as low as 0.87 μM (with S/N = 3) and the linear response is up to 10 μM.
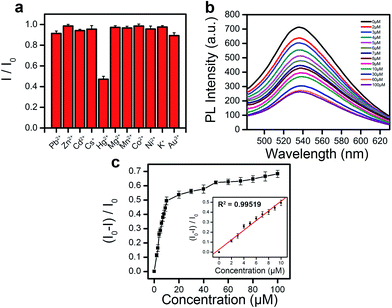 |
| Fig. 5 Sensitive and selective optical detection of Hg2+ ions. (a) PL of PEDOT-QDs (200 μg mL−1 in water) is selectively quenched by Hg2+ (100 μM), but not other metal ions at the concentration. (b) The PL emission spectra of PEDOT-QDs with various concentrations of Hg2+. (c) The relative concentration-dependent fluorescence response of PEDOT-QDs. The inset shows the linear response range. The error bars represent the standard deviation of the measurements from three different samples. | |
It has been previously reported that nitrogen-containing imidazole33 and sulfur-containing thiophene34 can bind with Hg2+ with high affinity. Therefore, Hg2+ ions act as coordinating centers to bridge several PEDOT-QDs together, by interacting with thiophene groups from PEDOT and imidazole groups from BMIM associated with PEDOT-QD via π–π interactions. In turn, fluorescence quenching occurs due to aggregation of QDs. Indeed, Hg2+-induced QD aggregations are observed under AFM (Fig. S8, ESI†).
Conclusions
In conclusion, we have developed a facile and general strategy to synthesize novel fluorescent polymer quantum dots derived from non-fluorescent PEDOT. Such molecularly light, perfectly photo-stable PEDOT-QDs promise a wide range of applications in bio-imaging (e.g., single molecule tracking in live cells) and sensitive optical detection. In principle, the synthetic route demonstrated here can be easily modified (e.g., using different polymer precursors, ionic liquids for polymerization, and solvents) to produce different types of polymer QDs with various interesting properties (preliminary results obtained).
Acknowledgements
This work was supported by the Singapore Ministry of Education under the AcRF Tier 2 grant (MOE2011-T2-2-010), NNSF of China (21275076 and 61328401), Jiangsu Provincial Funds for Distinguished Young Scholars (SK20130046). We would also like to acknowledge the assistance from Arundithi Ananthanarayanan, Dr Parimal Routh, Chew Huai Ping and Xu Qian during sample synthesis, for sample preparation and discussion.
References
- I. L. Medintz, H. T. Uyeda, E. R. Goldman and H. Mattoussi, Nat. Mater., 2005, 4, 435–446 CrossRef CAS PubMed
.
- D. Bera, L. Qian, T. K. Tseng and P. H. Holloway, Materials, 2010, 3, 2260–2345 CrossRef CAS PubMed
.
- A. M. Derfus, W. C. W. Chan and S. N. Bhatia, Nano Lett., 2003, 4, 11–18 CrossRef
.
- X. T. Zheng, A. Than, A. Ananthanaraya, D. H. Kim and P. Chen, ACS Nano, 2013, 7, 6278–6286 CrossRef CAS PubMed
.
- L. Li, G. Wu, G. Yang, J. Peng, J. Zhao and J. J. Zhu, Nanoscale, 2013, 5, 4015–4039 RSC
.
- H. J. Sun, L. Wu, W. L. Wei and X. G. Qu, Mater. Today, 2013, 16, 433–442 CrossRef CAS PubMed
.
- R. Freeman and I. Willner, Chem. Soc. Rev., 2012, 41, 4067–4085 RSC
.
- Y. Lou, Y. Zhao, J. Chen and J.-J. Zhu, J. Mater. Chem. C, 2014, 2, 595 RSC
.
- A. Ananthanarayanan, X. Wang, P. Routh, B. Sana, S. Lim, D.-H. Kim, K.-H. Lim, J. Li and P. Chen, Adv. Funct. Mater., 2014, 24, 3021–3026 CrossRef CAS
.
- X. Zhang, J. Yu, Y. Rong, F. Ye, D. T. Chiu and K. Uvdal, Chem. Sci., 2013, 4, 2143 RSC
.
- C. Wu, C. Szymanski, Z. Cain and J. McNeill, J. Am. Chem. Soc., 2007, 129, 12904–12905 CrossRef CAS PubMed
.
- C. Wu, Y. Jin, T. Schneider, D. R. Burnham, P. B. Smith and D. T. Chiu, Angew. Chem., Int. Ed., 2010, 49, 9436–9440 CrossRef CAS PubMed
.
- C. Wu and D. T. Chiu, Angew. Chem., Int. Ed., 2013, 52, 3086–3109 CrossRef CAS PubMed
.
- Z. Tian, J. Yu, C. Wu, C. Szymanski and J. McNeill, Nanoscale, 2010, 2, 1999–2011 RSC
.
- T. Kietzke, D. Neher, K. Landfester, R. Montenegro, R. Guntner and U. Scherf, Nat. Mater., 2003, 2, 408–412 CrossRef CAS PubMed
.
- Y. Chen, P. P. Gai, L. Jin, D. Zhu, D. B. Tian, E. S. Abdel-Halim, J. R. Zhang and J. J. Zhu, J. Mater. Chem. B, 2013, 1, 3451–3457 RSC
.
- D. C. Martin, J. Wu, C. M. Shaw, Z. King, S. A. Spanninga, S. Richardson-Burns, J. Hendricks and J. Yang, Polym. Rev., 2010, 50, 340–384 CrossRef CAS
.
- S. Bhandari, M. Deepa, S. Singh, G. Gupta and R. Kant, Electrochim. Acta, 2008, 53, 3189–3199 CrossRef CAS PubMed
.
- Y. Yao, N. Liu, M. T. McDowell, M. Pasta and Y. Cui, Energy Environ. Sci., 2012, 5, 7927 CAS
.
- K. Kinoshita, N. Matsunaga, M. Hiraoka, H. Yanagimoto and H. Minami, RSC Adv., 2014, 4, 8605 RSC
.
- H. J. Kim, I. S. Bae, S. J. Cho, J. H. Boo, B. C. Lee, J. Heo, I. Chung and B. Hong, Nanoscale Res. Lett., 2012, 7, 30 CrossRef PubMed
.
- X. Zhang, D. Chang, J. Liu and Y. Luo, J. Mater. Chem., 2010, 20, 5080 RSC
.
- D. Sun, L. Jin, Y. Chen, J. R. Zhang and J. J. Zhu, ChemPhysChem, 2013, 78, 227–234 CAS
.
- N. Xie and W. Luan, Nanotechnology, 2011, 22, 265609 CrossRef PubMed
.
- V. Armel, J. Rivnay, G. Malliaras and B. Winther-Jensen, J. Am. Chem. Soc., 2013, 135, 11309–11313 CrossRef CAS PubMed
.
-
A. Elschner, S. Kirchmeyer, W. Lovenich, U. Merker and K. Reuter, PEDOT: principles and applications of an intrinsically conductive polymer, CRC Press, Boca Raton, FL, 2011 Search PubMed
.
- J. J. Dubowski, C. N. Allen and S. Fafard, Appl. Phys. Lett., 2000, 77, 3583 CrossRef CAS PubMed
.
- S. Chakrabarti, S. Fathpour, K. Moazzami, J. Phillips, Y. Lei, N. Browning and P. Bhattacharya, J. Electron. Mater., 2004, 33, L5–L8 CrossRef CAS PubMed
.
- X. Li, H. Wang, Y. Shimizu, A. Pyatenko, K. Kawaguchi and N. Koshizaki, Chem. Commun., 2011, 47, 932–934 RSC
.
- G. S. He, L. S. Tan, Q. Zheng and P. N. Prasad, Chem. Rev., 2008, 108, 1245–1330 CrossRef CAS PubMed
.
- Y. Wang, X. Yang, T. C. He, Y. Gao, H. V. Demir, X. W. Sun and H. D. Sun, Appl. Phys. Lett., 2013, 102, 021917 CrossRef PubMed
.
- X. Ran, H. Sun, F. Pu, J. Ren and X. Qu, Chem. Commun., 2013, 49, 1079–1081 RSC
.
- S. Madhu, D. K. Sharma, S. K. Basu, S. Jadhav, A. Chowdhury and M. Ravikanth, Inorg. Chem., 2013, 52, 11136–11145 CrossRef CAS PubMed
.
- A. Pal and B. Bag, J. Photochem. Photobiol., A, 2012, 240, 42–49 CrossRef CAS PubMed
.
Footnote |
† Electronic supplementary information (ESI) available. See DOI: 10.1039/c4mh00066h |
|
This journal is © The Royal Society of Chemistry 2014 |
Click here to see how this site uses Cookies. View our privacy policy here.