DOI:
10.1039/C3NR05444F
(Review Article)
Nanoscale, 2014,
6, 65-75
Polymeric nanotherapeutics: clinical development and advances in stealth functionalization strategies
Received
14th October 2013
, Accepted 29th October 2013
First published on 4th November 2013
Abstract
Long-circulating polymeric nanotherapeutics have garnered increasing interest in research and in the clinic owing to their ability to improve the solubility and pharmacokinetics of therapeutic cargoes. Modulation of carrier properties promises more effective drug localization at the disease sites and can lead to enhanced drug safety and efficacy. In the present review, we highlight the current development of polymeric nanotherapeutics in the clinic. In light of the importance of stealth properties in therapeutic nanoparticles, we also review the advances in stealth functionalization strategies and examine the performance of different stealth polymers in the literature. In addition, we discuss the recent development of biologically inspired “self” nanoparticles, which present a differing stealth concept from conventional approaches.
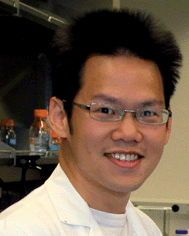 Che-Ming J. Hu | Dr. Che-Ming J. Hu is a postdoctoral researcher in the Department of NanoEngineering at the University of California, San Diego. He received his Ph.D. in Bioengineering at the University of California, San Diego. His research interest is in developing functionalized and biomimetic nanoparticles for cancer and antibacterial treatments. |
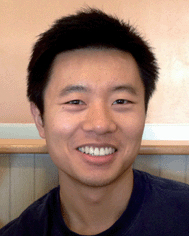 Ronnie H. Fang | Ronnie H. Fang is a Ph.D. student in the Department of NanoEngineering at the University of California, San Diego. He received his Bachelor's degrees in both Engineering Physics and Biochemistry & Cell Biology at the University of California, San Diego. His current research interests include the synthesis and applications of biomimetic nanoparticles. |
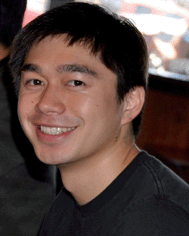 Brian T. Luk | Brian T. Luk is a Ph.D. student in the Department of Bioengineering at the University of California, San Diego. He received his Bachelor's degree in Materials Science & Engineering from Stanford University in 2011. His research interests include applications of biomimetic nanoparticles for cancer treatment. |
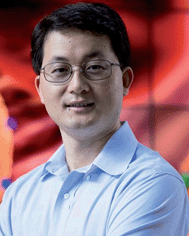 Liangfang Zhang | Dr. Liangfang Zhang is an Associate Professor in the Department of NanoEngineering and Moores Cancer Center at the University of California, San Diego. He received his Ph.D. in Chemical Engineering at the University of Illinois at Urbana-Champaign. His research interests focus on the design, synthesis, characterization and evaluation of nanostructured biomaterials for drug delivery to improve or enable treatments of human diseases, with particular interests in cancers and bacterial infections. |
1. Introduction
The advent of nanoparticle-based drug delivery systems has made a significant impact in the clinic.1 In the last two decades, a plethora of nanoparticle-based therapeutic and diagnostic agents have been developed for the treatment of cancer, diabetes, pain, bacterial infections, asthma, etc.2,3 Among the different nanotherapeutics, polymeric nanoparticles represent a unique class of nanocarriers that promise increased efficacy through controlled drug delivery to diseased sites. Well recognized advantages and features of polymeric nanoparticles include sustained drug release, delivery of poorly soluble drugs, multi-drug co-delivery, and the ability to be functionalized with targeting ligands for targeted delivery.4–9 In addition, many biocompatible and biodegradable polymers, such as poly(D,L-lactide-co-glycolide) (PLGA), poly(lactic acid) (PLA), poly(glutamic acid) (PGA), poly(caprolactone) (PCL), N-(2-hydroxypropyl)-methacrylate copolymers (HPMA), and poly(amino acids),10,11 provide safe and non-toxic nanoparticle building blocks primed for in vivo administration. Extensive research efforts in nanoparticle design have enabled advanced functionalities including disease targeting,2,12–15 stimuli-responsive triggers,16–18 combinatorial drug encapsulation,19–21 and temporally controlled drug release.22,23 Aided by recent developments in polymer engineering and preparation methods, polymeric nanoparticles can now be reliably manufactured and fine-tuned toward optimal performance.24–27 These improved manufacturing techniques significantly benefit the bench-to-bedside translation of polymeric nanotherapeutics, giving rise to a growing number of nanoformulations in clinical use or test. The increasing number of nanoparticles in clinical and preclinical studies also helps to fuel continuing research interest in the various interactions between biological components and synthetic nanomaterials; much effort is devoted to developing novel immune-evasive platforms that promise enhanced pharmacokinetic profiles and therapeutic efficacy. In this article, we highlight recent clinical development regarding polymeric nanotherapeutics and review emerging stealth technologies aimed at passivating nanoparticles against the multi-faceted nature of immune clearance.
2. Clinical development of polymeric nanotherapeutics
The advent of “stealth” nanoparticles has made great impact on nanoparticle drug delivery, particularly toward cancer treatment. First introduced in 1994 in a landmark paper by Langer and his colleagues,28 polymeric nanoparticles grafted with polyethylene glycol (PEG) can circulate in blood for an extended period of time owing to the passivation effect of PEG. By providing a hydration layer and steric barrier surrounding the polymeric core, PEG grafting can reduce non-specific binding of serum proteins to the particles, thereby reducing their clearance by cells of the mononuclear phagocytic system (MPS).29,30 These long-circulating nanoparticles have been shown to benefit drug delivery to the tumor microenvironment owing to the enhanced permeation and retention (EPR) phenomenon.31–33 A number of formulations based on PEGylated polymeric nanoparticles are currently undergoing clinical trials (Table 1). In general, these drug-loaded nanoparticles exhibit prolonged systemic circulation lifetime, sustained drug release kinetics, and better tumor accumulation as compared to small-molecule drugs.34–37 Several notable polymeric nanoformulations in clinical trials are reviewed herein.
Table 1 PEGylated polymeric NPs in clinical trials
Agent |
Formulation |
Company |
Indication |
Status |
Reference |
SP1049C |
PEGylated glycoprotein micelle of doxorubicin |
Supratek Pharma Inc. |
Various cancers |
Phase II |
51
|
CRLX101 |
PEG–cyclodextrin + camptothecin |
Cerulean Pharma |
Various cancers |
Phase II |
47 and 52
|
NC-6004 |
PEG–poly(amino acid) + cisplatin |
NanoCarrier Co. |
Various cancers |
Phase II |
53
|
NK105 |
PEG–poly(aspartate) + paclitaxel |
Nippon Kayaku Co., Ltd |
Various cancers |
Phase III |
54 and 55
|
NK911 |
PEG–poly(aspartate) + doxorubicin |
Nippon Kayaku Co., Ltd |
Various cancers |
Phase I |
56
|
NK012 |
Polymeric micelle SN-38 |
Nippon Kayaku Co., Ltd |
Various cancers |
Phase II |
57
|
BIND-014 |
PEG–PLGA + docetaxel |
BIND Therapeutics |
Various cancers |
Phase II |
27
|
CALAA-01 |
Transferrin-targeted PEG–cyclodextrin + siRNA |
Calando Pharmaceuticals |
Solid tumors |
Phase I |
50
|
Genexol-PM (Cynviloq™, IG-001) |
PEG–PLA + paclitaxel |
Sorrento Therapeutics |
Various cancers |
Phase II, approved in S. Korea |
41, 42 and 58
|
Genexol-PM was the first commercialized polymeric nanotherapeutic and it is currently approved in South Korea for the treatment of metastatic cancer, non-small cell lung cancer, and ovarian cancer. Comprising of paclitaxel and monomethoxy poly(ethylene glycol)-block-poly(D,L-lactide) (mPEG-PLA),38 the ∼25 nm micellar formulation highlights the advantage of polymeric nanocarriers in delivering poorly soluble chemotherapy drugs. Compared to Taxol®, a paclitaxel formulation solubilized in castor oil Cremophor EL, Genexol-PM has also demonstrated stronger effectiveness as a radiosensitizer for the treatment of non-small cell lung cancer while decreasing the exposure of paclitaxel in normal tissues.39 These benefits can be attributed to the physicochemical properties of the nanocarriers, which allow them to exploit the EPR phenomenon in tumors. In addition, Genexol-PM allows for a higher maximal tolerated dose (MTD) of paclitaxel as compared to Taxol,40 which contains a solvent that may cause allergic reactions. The formulation has undergone Phase II clinical trials in the United States and is undergoing several other trials involving combinations with other chemotherapeutics.41,42
CRLX101 is another example of an anti-cancer drug-loaded polymeric nanoparticle currently undergoing human clinical trials. The formulation is comprised of a cyclodextrin–poly(ethylene glycol) copolymer chemically conjugated to camptothecin (CPT), a potent anticancer drug that inhibits topoisomerase 1. The cyclodextrin on CRLX101 is capable of forming inclusion complexes with hydrophobic small molecules, which helps to address the poor water solubility issue of CPT. CRLX101 containing ∼10 wt% CPT is prepared from the self-assembly of CPT-conjugated copolymers, which form nanoparticles of 20 to 60 nm in size.43 The morphological and stealth features of the nanoparticles significantly prolong their residence time in the bloodstream, with a circulation half-life of approximately 1 day in rodents.44 Tail vein injection of CRLX101 to mice bearing tumor xenografts has revealed that the nanoparticle can localize at the tumoral sites. The formulation has also shown increased anti-tumor activity in murine models compared to FDA-approved CPT analogues.45,46 CRLX101 is currently in a Phase II clinical trial and recent publications on the platform show strong evidence that results from animal studies are translatable to humans.47
Also comprising of a cyclodextrin-containing polymer is CALAA-01, which is a nanoformulation carrying siRNA for targeted RNA interference (RNAi) therapy. These nanoparticles are prepared via the self-assembly of cyclodextrin-containing polymers in the presence of nucleic acids, which yields colloidal particles approximately 70 nm in diameter.48 The particles are also stabilized with PEG and functionalized with a targeting ligand, transferrin, through the host–guest inclusion of adamantane tethers and cyclodextrins. The platform highlights the application of nanoengineering design to overcome the many physiological delivery barriers and facilitate effective delivery of fragile therapeutic cargoes. By providing a protective barrier that precludes siRNA from plasma degradation and renal clearance, CALAA-01 enables the nucleic acid-based therapeutic to be systemically administered. In addition, the targeting ligands are incorporated to promote the intracellular uptake of CALAA-01,49 and the nanocarriers readily undergo particle disassembly in acidic environments to promote endosomal escape. These design rationales serve to address the many challenges of siRNA therapeutics, including poor stability and inadequate transfection efficiency. CALAA-01 is currently undergoing a Phase Ib clinical trial against melanoma; it has demonstrated evidence of successful RNAi in humans.50
BIND-014 is another polymeric nanoformulation consisting of PEGylated PLA co-polymer loaded with docetaxel, an anti-mitotic chemotherapeutic. Particularly notable in BIND-014 is the incorporation of targeting ligands specific for prostate-specific membrane antigen (PSMA), a surface marker highly expressed in prostate cancer cells and the neovasculature of other solid malignant tumors. The development of BIND-014 involved the screening of a combinatorial library of hundreds of nanoparticle formulations, and an optimal formulation was identified among particles with varying sizes, surface hydrophilicity, drug loading, and drug release kinetics. This rigorous screening process resulted in a robust formulation that balances the stealth properties of the PEG coating and the targeting capabilities of surface ligands. As a result, BIND-014 was shown to increase the concentration of docetaxel in mouse xenografts by up to an order of magnitude compared to conventional docetaxel formulations, resulting in a significant improvement in the drug's anti-tumor activity without increasing its toxicity. Thorough testing of the BIND-014 formulation was carried out in other animals as well, such as rats and nonhuman primates, prior to clinical translation. BIND-014 demonstrated differentiated efficacy in preclinical models of both prostate and non-prostate solid tumors. In Phase I clinical studies, the formulation was well-tolerated and demonstrated predictable and manageable toxicities.27 There are currently two Phase II clinical trials to evaluate the therapeutic activity of BIND-014 in non-small cell lung carcinoma and metastatic, castration-resistant prostate cancer. Other examples of polymeric nanotherapeutics can be found in Table 1.
3. Development of stealth functionalization strategies
A common feature behind the many polymeric nanotherapeutics in clinical development is their stealth functionality, which enables prolonged pharmacokinetics and improved biodistribution of the particles. Currently, PEG remains the gold standard for stealth functionalization in clinics, and optimization of PEG coating on polymeric nanoparticles has been extensively studied.59 Stealth functionality imparted by PEG to nanoparticles is found to be strongly influenced by its length and surface density on nanoparticle surfaces.60–64 PEG can adopt either a mushroom or a brush-like conformation depending on its length and density; scarcely distributed long PEG favors mushroom conformation and densely packed short PEG favors brush-like conformation.60,65 Generally speaking, higher surface coverage of PEG increases the circulation time of nanoparticles, and PEG with a molecular weight of 5000 Da provides the ideal surface coating for stealth functionalization.23,60
While PEGylation remains the most widely accepted standard for stealth functionalization, recent observations of anti-PEG immunological response have prompted much scrutiny over the immunological implications of PEG.66 There has been conflicting evidence in the literature in regards to the induction of PEG-specific antibodies,67–69 and it has been proposed that repeated administration of PEGylated nanoparticles leads to the production of anti-PEG antibodies, which subsequently increases phagocytosis by the reticuloendothelial system (RES) and results in an accelerated blood clearance phenomenon.70–72 In addition, complement activation through both the classical and alternative pathways by PEG has been reported.73–75 While fine-tuning of PEG length and density has shown much promise in attenuating PEG-associated immune responses,79,80 researchers have also begun to explore alternative long-circulation strategies with the aim of developing nanoparticles with better compatibility and improved performance. Alternative polymer-based stealth functionalization strategies for emerging polymeric nanotherapeutics are summarized in Fig. 1 and described in the following sections.
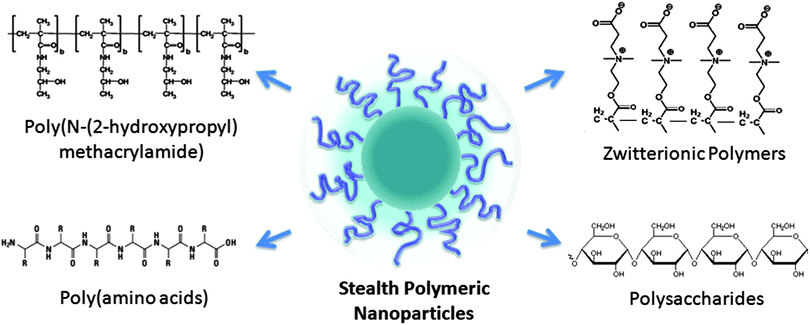 |
| Fig. 1 Examples of alternative hydrophilic polymers for nanoparticle stealth functionalization. | |
3.1 Synthetic polymers for stealth functionalization
Poly[N-(2-hydroxypropyl)methacrylamide] (HPMA) is a hydrophilic polymer with multiple functionalization sites. Upon conjugation with hydrophobic drug molecules, unimolecular micelles can form via a self-assembly process. Such HPMA–drug conjugates have been extensively studied for drug delivery applications.76–80 In contrast to PEG, the multiple functionalization sites on HPMA allow for multiple therapeutic compounds to be covalently attached. Drug-loaded unimolecular HPMA has shown significantly improved pharmacokinetic profiles as compared to free drugs80,81 and has entered various stages of clinical trials.82–84 Owing to HPMA's hydrophilicity, biocompatibility, and lack of immunogenicity,78,85 the polymer has also drawn many research interests as a stealth functionalization candidate in the development of polymeric nanocarriers. HPMA has been applied in place of PEG to stabilize polycation–DNA micellar complexes.86,87 Neutral hydrophilic HPMA shells have been grafted onto gene delivery vesicles with the aim to minimize plasma protein interactions and prolong the circulation time.88 Core–shell structured nanoparticles with a biodegradable polymeric core and a hydrophilic HPMA corona have also been prepared through the self-assembly of block copolymers. Nanoparticles consisting of HPMA–poly(caprolactone) (PCL) and of HPMA–poly(D,L-lactide) (PLA) have been prepared using both A–B–A triblock copolymers and star-shaped block copolymers.89–91 For both HPMA–PCL and HPMA–PLA copolymers, particles approximately 100–150 nm in size have been prepared. Encapsulation of hydrophobic therapeutic cargoes has also been demonstrated in these HPMA grafted nanocarriers using indomethacin and paclitaxel as model drugs.
Another type of synthetic polymer that has emerged as a compelling alternative to PEG is betaine-based polymers, which are zwitterionic materials with ultralow-fouling properties. Similar to many hydrophilic polymers, the zwitterionic material achieves its low fouling property through its hydration effect. However, in contrast to most hydrophilic polymers that bind water through hydrogen bonding, the zwitterionic material possesses a stronger hydration effect attributable to electrostatic interactions.92,93 Polybetaine functionalized surfaces have shown significantly reduced non-specific protein adsorption94–97 and biofilm formation.98–100 Therapeutic materials coated in polybetaines also display unique advantages over those decorated in other hydrophilic polymers. Notably, therapeutic proteins stabilized with poly(carboxybetaine) (PCB) display a higher substrate binding affinity as compared to those conjugated with PEG. Such an enhanced bioactivity is attributed to the superhydrophilic nature of PCB in contrast to the inherent amphiphilicity in PEG.101 Implant materials containing PCB also elicit minimal foreign-body reaction owing to the polymer's ultralow-fouling properties.102 Numerous nanoparticle platforms, including gold,103 iron oxide,104 silica,103 liposomes,105 and hydrogels,105–107 have been successfully functionalized with PCB, which helps to improve the particles' colloidal stability in protein solutions. Regarding polymeric nanoparticles, PCB-grafted PLGA nanoparticles have been prepared using PCB–PLGA block copolymers.108 The sharp polarity contrast between the PCB and PLGA blocks allows for an efficient nanoparticle self-assembly. Uniquely, the nanoparticles retain their stability even under lyophilization conditions in the absence of a cryoprotectant, which can likely be attributed to PCB's strong hydration. A biodistribution study of PCB-grafted and PEG-grafted poly(acrylic acid)-b-poly(lactide) nanoparticles also reveals comparable in vivo survival in the bloodstream,109 affirming PCB's potential as a stealth coating.
Many other synthetic polymers have been explored as alternative stealth coatings for nanocarriers. Examples of these polymers include poly(vinyl alcohol) (PVA),110,111 poly(oxazoline),112 poly(4-acryloylmorpholine) (PAcM),113,114 poly(N,N-dimethylacrylamide) (PDAAm),114,115 and poly(N-vinyl-2-pyrrolidone) (PVP).113–115 In a pharmacokinetic study using a rat model, Ishihara et al. examined the circulation half-lives of polymeric nanoparticles grafted with PVP, PAcM, and PDAAm and compared the results to that of PEGylated nanoparticles.114 All four polymers successfully extended the nanoparticle residence time in the circulation, and PEG yielded the longest half-life among the group. Interestingly, however, upon repeated injections, PEG-grafted nanoparticles exhibited an accelerated blood clearance phenomenon whereas PVP-, PAcM-, and PDAAm-grafted particles did not. The accelerated clearance was found to correlate with the production of IgM antibodies, which was elicited by PEG but not by PVP. The study highlights potential alternative materials for developing stealth nanocarriers with improved performance.
3.2 Biopolymers for stealth functionalization
Poly(amino acid)s (PAAs) are a major class of biopolymers that have been explored as stealth coating for nanocarriers. As compared to synthetic polymers, PAAs are susceptible to degradation by proteases, which helps reduce the risks of in vivo accumulation.116 Deierkauf et al. first examined the anti-phagocytic property of poly(amino acid) by grafting polystyrene latex beads with polyglutamic acid. The amino acid coating proved to be an effective inhibitor of particle uptake, which was ascribed to the electrostatic repulsion between the amino acids and the external surface charge on the polymorphonuclear leukocytes.117 The potential of PAAs in improving nanoparticles' pharmacokinetic profiles was further demonstrated on liposomes by Romberg et al., who showed that liposomes coated with poly(hydroxyethyl-L-asparagine) (PHEA) can outlast PEG-coated liposomes in the circulation.118 Upon intravenous administration in rat at less than 0.25 μmol kg−1, PHEA-coated liposomes showed superior survival in the blood as compared to PEG-coated liposomes. In addition, upon repeated injections, PHEA-coated liposomes exhibited a less pronounced accelerated blood clearance phenomenon. The stealth functionality and the excellent biodegradability of PAAs motivated the development of PAA-grafted polymeric nanoparticles. Block copolymers consisting of biodegradable polymers and PAAs have been prepared with different types of amino acids, including poly(aspartic acid),119,120 poly(glutamic acid),121–123 poly(cysteine),124 and poly(lysine).125 Generally, a hydrophilic peptide sequence is conjugated with a hydrophobic polymer such as PLA for the self-assembly of PAA-grafted nanoparticles.
Polysaccharides are another class of hydrophilic biopolymers that are frequently used to coat nanoparticles. These polymers are a desirable coating material for nanoparticle development owing to their excellent biodegradability and low immunogenicity.126–128 These biopolymers provide a hydrated surface that can be likened to the dense, carbohydrate-rich glycocalyx on cellular surfaces. In examining the effect of polysaccharides on the blood circulation half-life of polymeric nanoparticles, Passirani et al. demonstrated that the brush-like structure of heparin and dextran served to protect the nanoparticles from in vivo clearance. Covalent conjugation of heparin and dextran to a poly(methyl methacrylate)-based nanoparticle significantly prolonged the circulation half-life of the particles from 3 min to several hours. Sheng et al. also showed that functionalization with water-soluble chitosan reduced the phagocytic uptake efficiency and retarded the blood clearance of PLA nanoparticles. An impressive finding was the fact that nanoparticle functionalization with both PEG and chitosan yielded a circulation half-life of 63 h, which was much longer than that of the PEGylated particles reported in the study. The extended circulation time was attributed in part to the charge neutralization between PEG and chitosan and demonstrated the stealth potential of polysaccharides. Among other carbohydrate-based biopolymers, poly(sialic acid) (PSA) has also drawn significant interest as a stealth moiety because the human body has no specific receptors for the compound.129 Adoption of PSA by foreign pathogens and metastatic cancer cells for antigen masking and in vivo survival130–132 also suggests PSA's stealth potential. Conjugation with PSA has successfully prolonged the pharmacokinetic profiles of several protein therapeutics133,134 as well as quantum dot nanoparticles.135 Sialic acid has been applied to PLGA nanoparticles for drug delivery applications and has been shown to prolong particle residence within the brain.136,137
3.3 Biologically inspired stealth strategies
In the engineering of stealth nanocarriers that are capable of evading immune clearance, researchers have also taken design cues from biology by exploiting the surface mechanisms behind blood cells' long circulation. Early work on liposomal carriers demonstrated that incorporation of sialylated glycolipids and glycoproteins, which are major components on erythrocyte surfaces, significantly prolonged the liposomes' in vivo survival. While Surolia et al. first introduced asialogangliosides to liposomes as a biomimetic approach to improve hepatic drug delivery,138 later studies by Allen et al. showed that the incorporation of the ganglioside GM1 greatly prolonged the liposome's residence time in the blood. The stealth effect of GM1 was observed across several different liposomal formulations. For instance, addition of a 0.07 molar ratio of GM1 to a sphingomyelin liposome increased the blood-to-RES ratio from 0.02 to 5.7 2 h post-injection.139 This enhanced blood residence was attributed to GM1's dense surface carbohydrates that mimic cellular membranes. In addition, the role of terminal sialyl groups on these glycolipids was also highlighted as incorporation of asialogangliosides showed a less pronounced stealth effect. Optimization of the biologically inspired stealth liposome by Allen et al. resulted in a liposomal composition that mimicked the outer leaflet of red blood cell (RBC) membranes, consisting of egg phosphatidylcholine/sphingomyelin/cholesterol/ganglioside GM1 at a 1
:
1
:
1
:
0.14 molar ratio.140 Liposomal formulations containing other sialic acid-containing gangliosides,141–143 ganglioside derivatives,144–146 and glycoproteins147 also showed an extended circulation time in the blood. The stealth effect of these glycosylated complexes has been ascribed to their ability to fend off complement activation and macrophage uptake.141,148
Despite the early success of biologically inspired stealthy liposomes, the utilization of biomolecules for stealth functionalization remained relatively unexplored in the development of polymeric nanocarriers. This was in part due to the engineering challenge in functionalizing nanoscale substrates. To bestow nanocarriers with biomimetic functionalities, biomolecules such as proteins and glycans need to be attached to the particles in a non-disruptive and regio-selective manner. With the advances in molecular biology and nanoengineering techniques, biologically inspired stealth polymeric nanoparticles have recently emerged. Possessing active biological components on their surfaces, these nanoparticles are unlike conventional stealth particles that rely on hydration coronas for in vivo survival. Currently, techniques to prepare biologically inspired polymeric nanoparticles can be classified into a bottom-up approach based on protein conjugations and a top-down approach based on cell membrane coating.
3.3.1 Polymeric nanoparticles tagged with molecular self markers.
Among the many surface proteins and molecules present on mammalian cellular membranes, an integrin-associated protein CD47 has gained much research interest as a “marker-of-self” that can protect cells against macrophage uptake through an inhibitory action via SIRPα binding.149 The protein and its analogues have been identified on cancer cells and viruses as immune-evasive masking.150,151 Such anti-phagocytic properties have motivated researchers to apply the protein to mask foreign materials from immune recognition.152,153 Recently, CD47 and its derivative peptide have been attached to polystyrene nanoparticles to enhance their delivery and inhibit phagocytic clearance (Fig. 2). To enable controlled CD47 functionalization on nanoparticles, Rodriguez et al. mixed 160 nm streptavidin-coated polystyrene beads with a recombinant CD47 protein expressing regioselective biotinylation. The streptavidin–biotin coupling approach allowed CD47 to be coated in the proper orientation at a controllable density. In addition to CD47-functionalized beads, the authors also identified a “minimal” self peptide sequence (GNYTCEVTELTREGETIIELK), which was also applied for nanoparticle camouflage. Studies on the CD47- and self peptide-functionalized nanobeads showed that they could inhibit myosin-II recruitment at the phagocytic synapse, thereby suppressing the ensuing phagocytosis. The anti-phagocytic effect of CD47 functionalization was shown to be highly potent, requiring approximately 1 molecule per 45
000 nm2 (equivalent to the lowest CD47 densities reported for human RBCs), suggesting that a nanoparticle 60 nm in radius needs only one CD47 molecule to achieve the active stealth effect.154
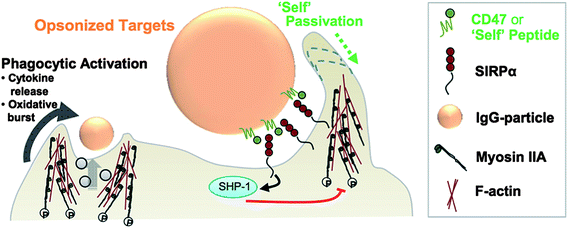 |
| Fig. 2 Schematic of a “self” nanoparticle prepared by immobilizing CD47 or its peptide variant on the nanoparticle surface. Signaling between CD47 or a minimal “self” peptide and their receptor, SIRPα, inhibits phagocytic activation. Reproduced from ref. 154 with permission from the American Association for the Advancement of Science. | |
The difference between the active stealth approach behind the “self” nanoparticles and the passive stealth approach behind conventional PEGylated nanoparticles was tellingly demonstrated in in vivo studies conducted with pre-opsonized nanoparticles. Recognizing the challenge in maintaining stealth functionalization following nanoparticle opsonization, Rodriguez et al. showed that the “self” nanoparticles retained their anti-phagocytic properties in vivo despite controlled opsonization with IgG. In contrast, PEGylated nanoparticles were completely stripped of their stealth properties upon opsonization, exhibiting an equivalent blood residence time to non-functionalized polystyrene nanobeads. The authors also demonstrated enhanced tumoral uptake and increased treatment efficacy using A549 lung adenocarcinoma cells and paclitaxel-loaded polystyrene nanobeads, validating the biologically inspired stealth approach to improve nanoparticle drug delivery.154
3.3.2 Polymeric nanoparticles camouflaged in cellular membranes.
In contrast to the bottom-up approach in attaching “marker-of-self” molecules to nanoparticles, a top-down approach, which exploits cellular membranes in their entirety to coat and camouflage polymeric nanoparticles from immune clearance, was first reported by Hu et al. Using a co-extrusion process, the investigators demonstrated successful coating of sub-100 nm PLGA nanoparticles with natural membranes derived from RBCs.155 The resulting RBC-membrane-coated nanoparticles (RBC–NPs) exhibited a core–shell structure, in which the RBC membrane formed a single bilayer around the polymeric core (Fig. 3). Interestingly, the cell membrane-coating approach adopts the same immune-evasive strategy adopted by many viruses in nature. It was recently discovered that hepatitis A and hepatitis C viruses are capable of “hijacking” cellular membranes, enclosing themselves in these non-immunogenic coatings to escape from neutralizing antibodies. The cell-membrane coating technique presents a unique biomimetic approach in nanoparticle stealth functionalization.156,157
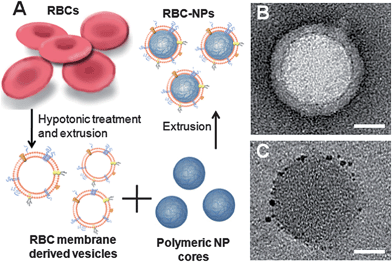 |
| Fig. 3 (A) Schematic of RBC–NP preparation. PLGA nanoparticles are coated in natural RBC membranes. (B) TEM visualization of the RBC–NP shows unilamellar membrane coating over the polymeric core (scale bar = 20 nm). Reproduced from ref. 155 with permission from the National Academy of Sciences. (C) Immunogold staining with anti-CD47 antibodies targeting the protein's extracellular domain shows that CD47 is present on the RBC–NP in a right-side-out orientation (scale bar = 20 nm). Reproduced from ref. 158 with permission from The Royal Society of Chemistry. | |
From a nanoengineering perspective, the membrane coating approach provides unprecedented control in enabling biomimetic surface functionalization on nanoscale polymeric substrates. By translocating cellular membranes in their entirety to nanoparticles, the complex biochemistry on cellular surfaces can be faithfully translocated as well.155 Careful studies of CD47 on the surface of RBC–NPs demonstrated that the particles possess the same density of the biomarker as its RBC source.158 More importantly, the proteins were shown to be oriented almost exclusively in the right-side-out fashion with the extracellular portion displayed on the particle surfaces (Fig. 3). This right-side-out orientation was ascribed in part to the electrostatic repulsion between the negatively charged PLGA nanoparticles and the negatively charged sialyl moieties residing on the exoplasmic side of the membranes. In addition, the stabilization effect by the exoplasmic glycans likely contributed to the observed membrane orientation. As a result of this oriented membrane coating, macrophage uptake of the RBC–NPs was significantly impeded in vitro. RBC–NPs also remained stable in phosphate buffered solution and serum unlike bare PLGA nanoparticles, suggesting that the glycan-rich membranes conferred stability to the polymeric cores. An important finding is that the RBC–NPs possessed longer blood survival as compared to an analogous PEGylated formulation. These results highlight the strength of the biomembrane-coated nanoparticles, whose self-camouflage presents a comprehensive evasion strategy against the multi-faceted nature of immune clearance.159,160
Much development on cellular-membrane coated nanoparticles has been made since RBC–NPs were first reported. Anti-phagocytic properties have been demonstrated with RBC membrane-coated gold nanoparticles161 and white blood cell membrane-coated silica particles,162 demonstrating the broad applicability of the approach. Toward drug delivery, RBC–NPs have been successfully loaded with doxorubicin and showed treatment efficacy against a leukemia cell line.163 The membrane bilayer coating was shown to enable a more sustained drug release profile by serving as a diffusion barrier. Incorporation of targeting ligands, such as folate and aptamers, into RBC–NPs has also been made possible through the insertion of lipid-tethered ligands into the RBC membrane bilayer, which demonstrated the platform's potential for targeted drug delivery.164 Perhaps most exciting is the recent demonstration of the RBC–NPs as a toxin nanosponge against pore-forming toxins. Owing to the particle's structural stability and biomimetic exterior, the RBC–NPs readily interact with the many pore-forming toxins that typically target and damage cellular membranes. Absorbed toxins remain locked-in by the nanoparticles and therefore can be safely metabolized in vivo.165 The toxin nanosponge application highlights the unique strengths of the membrane-camouflage stealth strategy, which allows nanoparticle interaction with other biomolecules as opposed to inhibiting such interactions using non-fouling polymers. This biologically inspired approach provides the venue for novel therapeutic interventions against pathogenic factors that interact with cellular surfaces.
4. Conclusions
Polymeric nanoparticles offer a robust and versatile platform for designing novel and more efficacious therapeutics. The impact of polymeric nanotherapeutics is evidenced by the many nanoformulations currently undergoing clinical trials. Ongoing research efforts continue to explore stealth strategies with the aim of developing nanocarriers that have better immunocompatibility and improved pharmacokinetic profiles. Such efforts are highlighted by a variety of hydrophilic polymers employed for stealth functionalization.
Notably, development of biologically inspired stealth nanoparticles highlights increased understanding of the complex immune clearance mechanisms and of the many biomolecules that contribute to immune evasion. Advancement in nanoengineering techniques has enabled nanoparticle surface functionalization with immunologically relevant components (i.e. CD47 and cellular membranes). These biologically inspired nanoparticles present a new paradigm in nanoparticle functionalization. Rather than hiding foreign particles behind a hydration layer, drug carriers can be camouflaged as “self”, readily evading immune clearance despite direct interaction with biological components in circulation. While the field of “self” nanoparticles remains in its nascent stage, the growing number of cell-derived nanocarriers in the literature provides a glimpse of the drug delivery potential behind biological functionalizations.166–170 Ongoing research in biologically inspired nanoparticles promises unique carrier functionalities that combine the intricacies of natural biomolecules with the flexibility and robustness of synthetic nanomaterials.
Acknowledgements
This work is supported by the National Science Foundation Grant DMR-1216461.
References
- O. C. Farokhzad and R. Langer, Adv. Drug Delivery Rev., 2006, 58, 1456–1459 CrossRef CAS PubMed.
- L. Brannon-Peppas and J. O. Blanchette, Adv. Drug Delivery Rev., 2004, 56, 1649–1659 CrossRef CAS PubMed.
- E. S. Kawasaki and A. Player, Nanomedicine, 2005, 1, 101–109 CrossRef CAS PubMed.
- A. Fassas, R. Buffels, P. Kaloyannidis and A. Anagnostopoulos, Br. J. Haematol., 2003, 122, 161–163 CrossRef.
- J. Kreuter, V. E. Petrov, D. A. Kharkevich and R. N. Alyautdin, J. Controlled Release, 1997, 49, 81–87 CrossRef CAS.
- J. C. Leroux, E. Allemann, F. DeJaeghere, E. Doelker and R. Gurny, J. Controlled Release, 1996, 39, 339–350 CrossRef CAS.
- R. S. Raghuvanshi, Y. K. Katare, K. Lalwani, M. M. Ali, O. Singh and A. K. Panda, Int. J. Pharm., 2002, 245, 109–121 CrossRef CAS.
- T. Safra, F. Muggia, S. Jeffers, D. D. Tsao-Wei, S. Groshen, O. Lyass, R. Henderson, G. Berry and A. Gabizon, Ann. Oncol., 2000, 11, 1029–1033 CrossRef CAS.
- U. Schroeder, P. Sommerfeld, S. Ulrich and B. A. Sabel, J. Pharm. Sci., 1998, 87, 1305–1307 CrossRef CAS PubMed.
- P. A. Gunatillake and R. Adhikari, Eur. Cells Mater., 2003, 5, 1–16 CAS.
- S. Acharya and S. K. Sahoo, Adv. Drug Delivery Rev., 2011, 63, 170–183 CrossRef CAS PubMed.
- D. C. Bibby, J. E. Talmadge, M. K. Dalal, S. G. Kurz, K. M. Chytil, S. E. Barry, D. G. Shand and M. Steiert, Int. J. Pharm., 2005, 293, 281–290 CrossRef CAS PubMed.
- O. C. Farokhzad, J. M. Karp and R. Langer, Expert Opin. Drug Delivery, 2006, 3, 311–324 CrossRef CAS PubMed.
- E. Ruoslahti, Drug Discovery Today, 2002, 7, 1138–1143 CrossRef CAS.
- V. P. Torchilin, Eur. J. Pharm. Sci., 2000, 11, S81–S91 CrossRef CAS.
- N. Rapoport, Prog. Polym. Sci., 2007, 32, 962–990 CrossRef CAS PubMed.
- D. Schmaljohann, Adv. Drug Delivery Rev., 2006, 58, 1655–1670 CrossRef CAS PubMed.
- B. Romberg, W. E. Hennink and G. Storm, Pharm. Res., 2008, 25, 55–71 CrossRef CAS PubMed.
- C. M. Hu and L. Zhang, Biochem. Pharmacol., 2012, 83, 1104–1111 CrossRef CAS PubMed.
- S. Aryal, C. M. Hu and L. Zhang, Mol. Pharm., 2011, 8, 1401–1407 CrossRef CAS PubMed.
- N. Kolishetti, S. Dhar, P. M. Valencia, L. Q. Lin, R. Karnik, S. J. Lippard, R. Langer and O. C. Farokhzad, Proc. Natl. Acad. Sci. U. S. A., 2010, 107, 17939–17944 CrossRef CAS PubMed.
- S. Sengupta, D. Eavarone, I. Capila, G. L. Zhao, N. Watson, T. Kiziltepe and R. Sasisekharan, Nature, 2005, 436, 568–572 CrossRef CAS PubMed.
- Z. Wang and P. C. Ho, Small, 2010, 6, 2576–2583 CrossRef CAS PubMed.
- R. H. Fang, K. N. H. Chen, S. Aryal, C. M. J. Hu, K. Zhang and L. F. Zhang, Langmuir, 2012, 28, 13824–13829 CrossRef CAS PubMed.
- M. Rhee, P. M. Valencia, M. I. Rodriguez, R. Langer, O. C. Farokhzad and R. Karnik, Adv. Mater., 2011, 23, 79–83 CrossRef PubMed.
- Y. T. Kim, B. L. Chung, M. M. Ma, W. J. M. Mulder, Z. A. Fayad, O. C. Farokhzad and R. Langer, Nano Lett., 2012, 12, 3587–3591 CrossRef CAS PubMed.
- J. Hrkach, D. Von Hoff, M. Mukkaram Ali, E. Andrianova, J. Auer, T. Campbell, D. De Witt, M. Figa, M. Figueiredo, A. Horhota, S. Low, K. McDonnell, E. Peeke, B. Retnarajan, A. Sabnis, E. Schnipper, J. J. Song, Y. H. Song, J. Summa, D. Tompsett, G. Troiano, T. Van Geen Hoven, J. Wright, P. LoRusso, P. W. Kantoff, N. H. Bander, C. Sweeney, O. C. Farokhzad, R. Langer and S. Zale, Sci. Transl. Med., 2012, 4, 128ra139 CrossRef PubMed.
- R. Gref, Y. Minamitake, M. T. Peracchia, V. Trubetskoy, V. Torchilin and R. Langer, Science, 1994, 263, 1600–1603 CAS.
- J. Shi, A. R. Votruba, O. C. Farokhzad and R. Langer, Nano Lett., 2010, 10, 3223–3230 CrossRef CAS PubMed.
- F. M. Veronese and G. Pasut, Drug Discovery Today, 2005, 10, 1451–1458 CrossRef CAS.
- M. E. Davis, Z. G. Chen and D. M. Shin, Nat. Rev. Drug Discovery, 2008, 7, 771–782 CrossRef CAS PubMed.
- S. M. Moghimi, A. C. Hunter and J. C. Murray, Pharmacol. Rev., 2001, 53, 283–318 CAS.
- D. Peer, J. M. Karp, S. Hong, O. C. Farokhzad, R. Margalit and R. Langer, Nat. Nanotechnol., 2007, 2, 751–760 CrossRef CAS PubMed.
- C. M. J. Hu, S. Kaushal, H. S. T. Cao, S. Aryal, M. Sartor, S. Esener, M. Bouvet and L. F. Zhang, Mol. Pharm., 2010, 7, 914–920 CrossRef CAS PubMed.
- C. M. J. Hu and L. F. Zhang, Curr. Drug Metab., 2009, 10, 836–841 CrossRef CAS.
- A. Z. Wang, F. Gu, L. F. Zhang, J. M. Chan, A. Radovic-Moreno, M. R. Shaikh and O. C. Farokhzad, Expert Opin. Biol. Ther., 2008, 8, 1063–1070 CrossRef CAS PubMed.
- L. Zhang, F. X. Gu, J. M. Chan, A. Z. Wang, R. S. Langer and O. C. Farokhzad, Clin. Pharm. Ther., 2008, 83, 761–769 CrossRef CAS PubMed.
- S. C. Kim, D. W. Kim, Y. H. Shim, J. S. Bang, H. S. Oh, S. Wan Kim and M. H. Seo, J. Controlled Release, 2001, 72, 191–202 CrossRef CAS.
- M. E. Werner, N. D. Cummings, M. Sethi, E. C. Wang, R. Sukumar, D. T. Moore and A. Z. Wang, Int. J. Radiat. Oncol., Biol., Phys., 2013, 86, 463–468 CrossRef CAS PubMed.
- T. Y. Kim, D. W. Kim, J. Y. Chung, S. G. Shin, S. C. Kim, D. S. Heo, N. K. Kim and Y. J. Bang, Clin. Cancer Res., 2004, 10, 3708–3716 CrossRef CAS PubMed.
- K. S. Lee, H. C. Chung, S. A. Im, Y. H. Park, C. S. Kim, S. B. Kim, S. Y. Rha, M. Y. Lee and J. Ro, Breast Cancer Res. Treat., 2008, 108, 241–250 CrossRef CAS PubMed.
- D. W. Kim, S. Y. Kim, H. K. Kim, S. W. Kim, S. W. Shin, J. S. Kim, K. Park, M. Y. Lee and D. S. Heo, Ann. Oncol., 2007, 18, 2009–2014 CrossRef PubMed.
- J. J. Cheng, K. T. Khin, G. S. Jensen, A. J. Liu and M. E. Davis, Bioconjugate Chem., 2003, 14, 1007–1017 CrossRef CAS PubMed.
- T. Schluep, J. Cheng, K. T. Khin and M. E. Davis, Cancer Chemother. Pharmacol., 2006, 57, 654–662 CrossRef CAS PubMed.
- S. Gaur, L. Chen, T. Yen, Y. Wang, B. Zhou, M. Davis and Y. Yen, Nanomedicine, 2012, 8, 721–730 CrossRef CAS PubMed.
- T. Numbenjapon, J. Wang, D. Colcher, T. Schluep, M. E. Davis, J. Duringer, L. Kretzner, Y. Yen, S. J. Forman and A. Raubitschek, Clin. Cancer Res., 2009, 15, 4365–4373 CrossRef CAS PubMed.
- S. Eliasof, D. Lazarus, C. G. Peters, R. I. Case, R. O. Cole, J. Hwang, T. Schluep, J. Chao, J. Lin, Y. Yen, H. Han, D. T. Wiley, J. E. Zuckerman and M. E. Davis, Proc. Natl. Acad. Sci. U. S. A., 2013, 110, 15127–15132 CrossRef CAS PubMed.
- S. H. Pun and M. E. Davis, Bioconjugate Chem., 2002, 13, 630–639 CrossRef CAS PubMed.
- D. W. Bartlett and M. E. Davis, Bioconjugate Chem., 2007, 18, 456–468 CrossRef CAS PubMed.
- M. E. Davis, J. E. Zuckerman, C. H. Choi, D. Seligson, A. Tolcher, C. A. Alabi, Y. Yen, J. D. Heidel and A. Ribas, Nature, 2010, 464, 1067–1070 CrossRef CAS PubMed.
- J. W. Valle, A. Armstrong, C. Newman, V. Alakhov, G. Pietrzynski, J. Brewer, S. Campbell, P. Corrie, E. K. Rowinsky and M. Ranson, Invest. New Drugs, 2011, 29, 1029–1037 CrossRef CAS PubMed.
- S. Svenson, M. Wolfgang, J. Hwang, J. Ryan and S. Eliasof, J. Controlled Release, 2011, 153, 49–55 CrossRef CAS PubMed.
- R. Plummer, R. H. Wilson, H. Calvert, A. V. Boddy, M. Griffin, J. Sludden, M. J. Tilby, M. Eatock, D. G. Pearson, C. J. Ottley, Y. Matsumura, K. Kataoka and T. Nishiya, Br. J. Cancer, 2011, 104, 593–598 CrossRef CAS PubMed.
- T. Hamaguchi, Y. Matsumura, M. Suzuki, K. Shimizu, R. Goda, I. Nakamura, I. Nakatomi, M. Yokoyama, K. Kataoka and T. Kakizoe, Br. J. Cancer, 2005, 92, 1240–1246 CrossRef CAS PubMed.
- Y. Matsumura and K. Kataoka, Cancer Sci., 2009, 100, 572–579 CrossRef CAS.
- Y. Matsumura, T. Hamaguchi, T. Ura, K. Muro, Y. Yamada, Y. Shimada, K. Shirao, T. Okusaka, H. Ueno, M. Ikeda and N. Watanabe, Br. J. Cancer, 2004, 91, 1775–1781 CrossRef CAS PubMed.
- T. Hamaguchi, T. Doi, T. Eguchi-Nakajima, K. Kato, Y. Yamada, Y. Shimada, N. Fuse, A. Ohtsu, S. Matsumoto, M. Takanashi and Y. Matsumura, Clin. Cancer Res., 2010, 16, 5058–5066 CrossRef CAS PubMed.
- T. Y. Kim, D. W. Kim, J. Y. Chung, S. G. Shin, S. C. Kim, D. S. Heo, N. K. Kim and Y. J. Bang, Clin. Cancer Res., 2004, 10, 3708–3716 CrossRef CAS PubMed.
- S. Stolnik, L. Illum and S. S. Davis, Adv. Drug Delivery Rev., 1995, 16, 195–214 CrossRef CAS.
- J. L. Perry, K. G. Reuter, M. P. Kai, K. P. Herlihy, S. W. Jones, J. C. Luft, M. Napier, J. E. Bear and J. M. DeSimone, Nano Lett., 2012, 12, 5304–5310 CrossRef CAS PubMed.
- R. Gref, M. Luck, P. Quellec, M. Marchand, E. Dellacherie, S. Harnisch, T. Blunk and R. H. Muller, Colloids Surf., B, 2000, 18, 301–313 CrossRef CAS.
- K. Avgoustakis, A. Beletsi, Z. Panagi, P. Klepetsanis, E. Livaniou, G. Evangelatos and D. S. Ithakissios, Int. J. Pharm., 2003, 259, 115–127 CrossRef CAS.
- A. Beletsi, Z. Panagi and K. Avgoustakis, Int. J. Pharm., 2005, 298, 233–241 CrossRef CAS PubMed.
- V. C. F. Mosqueira, P. Legrand, J. L. Morgat, M. Vert, E. Mysiakine, R. Gref, J. P. Devissaguet and G. Barratt, Pharm. Res., 2001, 18, 1411–1419 CrossRef CAS.
- I. Hamad, O. Al-Hanbali, A. C. Hunter, K. J. Rutt, T. L. Andresen and S. M. Moghimi, ACS Nano, 2010, 4, 6629–6638 CrossRef CAS PubMed.
- K. Knop, R. Hoogenboom, D. Fischer and U. S. Schubert, Angew. Chem., Int. Ed., 2010, 49, 6288–6308 CrossRef CAS PubMed.
- H. L. Ma, K. Shiraishi, T. Minowa, K. Kawano, M. Yokoyama, Y. Hattori and Y. Maitani, Pharm. Res., 2010, 27, 296–302 CrossRef CAS PubMed.
- T. Tagami, Y. Uehara, N. Moriyoshi, T. Ishida and H. Kiwada, J. Controlled Release, 2011, 151, 149–154 CrossRef CAS PubMed.
- H. Xu, K. Q. Wang, Y. H. Deng and D. W. Chen, Biomaterials, 2010, 31, 4757–4763 CrossRef CAS PubMed.
- J. K. Armstrong, G. Hempel, S. Koling, L. S. Chan, T. Fisher, H. J. Meiselman and G. Garratty, Cancer, 2007, 110, 103–111 CrossRef PubMed.
- K. Sroda, J. Rydlewski, M. Langner, A. Kozubek, M. Grzybek and A. F. Sikorski, Cell. Mol. Biol. Lett., 2005, 10, 37–47 CAS.
- X. Y. Wang, T. Ishida and H. Kiwada, J. Controlled Release, 2007, 119, 236–244 CrossRef CAS PubMed.
- V. C. F. Mosqueira, P. Legrand, A. Gulik, O. Bourdon, R. Gref, D. Labarre and G. Barratt, Biomaterials, 2001, 22, 2967–2979 CrossRef CAS.
- I. Hamada, A. C. Hunter, J. Szebeni and S. M. Moghimi, Mol. Immunol., 2008, 46, 225–232 CrossRef PubMed.
- J. Szebeni, L. Baranyi, S. Savay, J. Milosevits, R. Bunger, P. Laverman, J. M. Metselaar, G. Storm, A. Chanan-Khan, L. Liebes, F. M. Muggia, R. Cohen, Y. Barenholz and C. R. Alving, J. Liposome Res., 2002, 12, 165–172 CrossRef CAS PubMed.
- F. Greco and M. J. Vicent, Adv. Drug Delivery Rev., 2009, 61, 1203–1213 CrossRef CAS PubMed.
- A. Paul, M. J. Vicent and R. Duncan, Biomacromolecules, 2007, 8, 1573–1579 CrossRef CAS PubMed.
- J. Kopecek, P. Kopeckova, T. Minko and Z. Lu, Eur. J. Pharm. Biopharm., 2000, 50, 61–81 CrossRef CAS.
- P. Chytil, T. Etrych, C. Konak, M. Sirova, T. Mrkvan, B. Rihova and K. Ulbrich, J. Controlled Release, 2006, 115, 26–36 CrossRef CAS PubMed.
- P. Chytil, T. Etrych, C. Konak, M. Sirova, T. Mrkvan, J. Boucek, B. Rihova and K. Ulbrich, J. Controlled Release, 2008, 127, 121–130 CrossRef CAS PubMed.
- P. A. Vasey, S. B. Kaye, R. Morrison, C. Twelves, P. Wilson, R. Duncan, A. H. Thomson, L. S. Murray, T. E. Hilditch, T. Murray, S. Burtles, D. Fraier, E. Frigerio, J. Cassidy and C. R. C. P. I. I. Comm, Clin. Cancer Res., 1999, 5, 83–94 CAS.
- R. Duncan, Adv. Drug Delivery Rev., 2009, 61, 1131–1148 CrossRef CAS PubMed.
- M. J. Vicent, H. Ringsdorf and R. Duncan, Adv. Drug Delivery Rev., 2009, 61, 1117–1120 CrossRef CAS PubMed.
- R. Duncan, Nat. Rev. Cancer, 2006, 6, 688–701 CrossRef CAS PubMed.
- B. Rihova, K. Ulbrich, J. Kopecek and P. Mancal, Folia Microbiol., 1983, 28, 217–227 CrossRef CAS.
- C. Konak, L. Mrkvickova, O. Nazarova, K. Ulbrich and L. W. Seymour, Supramol. Sci., 1998, 5, 67–74 CrossRef CAS.
- D. Oupicky, C. Konak and K. Ulbrich, Mater. Sci. Eng., C, 1999, 7, 59–65 CrossRef.
- C. Konak, V. Subr, L. Kostka, P. Stepanek, K. Ulbrich and H. Schlaad, Langmuir, 2008, 24, 7092–7098 CrossRef CAS PubMed.
- B. S. Lele and J. C. Leroux, Macromolecules, 2002, 35, 6714–6723 CrossRef CAS.
- B. S. Lele and J. C. Leroux, Polymer, 2002, 43, 5595–5606 CrossRef CAS.
- N. Kang and J. C. Leroux, Polymer, 2004, 45, 8967–8980 CrossRef CAS PubMed.
- S. Y. Jiang and Z. Q. Cao, Adv. Mater., 2010, 22, 920–932 CrossRef CAS PubMed.
- Q. Shao, Y. He, A. D. White and S. Y. Jiang, J. Phys. Chem. B, 2010, 114, 16625–16631 CrossRef CAS PubMed.
- Z. Zhang, H. Vaisocherova, G. Cheng, W. Yang, H. Xue and S. Y. Jiang, Biomacromolecules, 2008, 9, 2686–2692 CrossRef CAS PubMed.
- W. Yang, T. Bai, L. R. Carr, A. J. Keefe, J. J. Xu, H. Xue, C. A. Irvin, S. F. Chen, J. Wang and S. Y. Jiang, Biomaterials, 2012, 33, 7945–7951 CrossRef CAS PubMed.
- J. T. Kirk, N. D. Brault, T. Baehr-Jones, M. Hochberg, S. Y. Jiang and D. M. Ratner, Biosens. Bioelectron., 2013, 42, 100–105 CrossRef CAS PubMed.
- Y. T. Li, A. J. Keefe, M. Giarmarco, N. D. Brault and S. Y. Jiang, Langmuir, 2012, 28, 9707–9713 CrossRef CAS PubMed.
- L. Mi and S. Y. Jiang, Biomaterials, 2012, 33, 8928–8933 CrossRef CAS PubMed.
- G. Cheng, G. Z. Li, H. Xue, S. F. Chen, J. D. Bryers and S. Y. Jiang, Biomaterials, 2009, 30, 5234–5240 CrossRef CAS PubMed.
- G. Z. Li, G. Cheng, H. Xue, S. F. Chen, F. B. Zhang and S. Y. Jiang, Biomaterials, 2008, 29, 4592–4597 CrossRef CAS PubMed.
- A. J. Keefe and S. Y. Jiang, Nat. Chem., 2012, 4, 60–64 Search PubMed.
- L. Zhang, Z. Q. Cao, T. Bai, L. Carr, J. R. Ella-Menye, C. Irvin, B. D. Ratner and S. Y. Jiang, Nat. Biotechnol., 2013, 31, 553–556 CrossRef CAS PubMed.
- W. Yang, L. Zhang, S. L. Wang, A. D. White and S. Y. Jiang, Biomaterials, 2009, 30, 5617–5621 CrossRef CAS PubMed.
- L. Zhang, H. Xue, C. L. Gao, L. Carr, J. N. Wang, B. C. Chu and S. Y. Jiang, Biomaterials, 2010, 31, 6582–6588 CrossRef CAS PubMed.
- Z. Q. Cao, L. Zhang and S. Y. Jiang, Langmuir, 2012, 28, 11625–11632 CrossRef CAS PubMed.
- G. Cheng, L. Mi, Z. Q. Cao, H. Xue, Q. M. Yu, L. Carr and S. Y. Jiang, Langmuir, 2010, 26, 6883–6886 CrossRef CAS PubMed.
- L. Zhang, H. Xue, Z. Q. Cao, A. Keefe, J. N. Wang and S. Y. Jiang, Biomaterials, 2011, 32, 4604–4608 CrossRef CAS PubMed.
- Z. Q. Cao, Q. M. Yu, H. Xue, G. Cheng and S. Y. Jiang, Angew. Chem., Int. Ed., 2010, 49, 3771–3776 CrossRef CAS PubMed.
- L. Zhang, Z. Q. Cao, Y. T. Li, J. R. Ella-Menye, T. Bai and S. Y. Jiang, ACS Nano, 2012, 6, 6681–6686 CrossRef CAS PubMed.
- H. Takeuchi, H. Kojima, H. Yamamoto and Y. Kawashima, J. Controlled Release, 2001, 75, 83–91 CrossRef CAS.
- J. U. Menon, S. Kona, A. S. Wadajkar, F. Desai, A. Vadla and K. T. Nguyen, J. Biomed. Mater. Res., Part A, 2012, 100, 1998–2005 CrossRef PubMed.
- M. C. Woodle, C. M. Engbers and S. Zalipsky, Bioconjugate Chem., 1994, 5, 493–496 CrossRef CAS.
- V. P. Torchilin, V. S. Trubetskoy, K. R. Whiteman, P. Caliceti, P. Ferruti and F. M. Veronese, J. Pharm. Sci., 1995, 84, 1049–1053 CrossRef CAS.
- T. Ishihara, T. Maeda, H. Sakamoto, N. Takasaki, M. Shigyo, T. Ishida, H. Kiwada, Y. Mizushima and T. Mizushima, Biomacromolecules, 2010, 11, 2700–2706 CrossRef CAS PubMed.
- Y. Kaneda, Y. Tsutsumi, Y. Yoshioka, H. Kamada, Y. Yamamoto, H. Kodaira, S. Tsunoda, T. Okamoto, Y. Mukai, H. Shibata, S. Nakagawa and T. Mayumi, Biomaterials, 2004, 25, 3259–3266 CrossRef CAS PubMed.
- B. Romberg, J. M. Metselaar, L. Baranyi, C. J. Snel, R. Bunger, W. E. Hennink, J. Szebeni and G. Storm, Int. J. Pharm., 2007, 331, 186–189 CrossRef CAS PubMed.
- F. A. Deierkauf, H. Beukers, M. Deierkauf and J. C. Riemersma, J. Cell. Physiol., 1977, 92, 169–175 CrossRef CAS PubMed.
- B. Romberg, C. Oussoren, C. J. Snel, W. E. Hennink and G. Storm, Pharm. Res., 2007, 24, 2394–2401 CrossRef CAS PubMed.
- H. Arimura, Y. Ohya and T. Ouchi, Macromol. Rapid Commun., 2004, 25, 743–747 CrossRef CAS.
- H. Arimura, Y. Ohya and T. Ouchi, Biomacromolecules, 2005, 6, 720–725 CrossRef CAS PubMed.
- C. Deng, X. S. Chen, J. Sun, T. C. Lu, W. S. Wang and X. B. Jing, J. Polym. Sci., Part A: Polym. Chem., 2007, 45, 3218–3230 CrossRef CAS.
- C. Deng, H. Y. Tian, P. B. Zhang, J. Sun, X. S. Chen and X. B. Jing, Biomacromolecules, 2006, 7, 590–596 CrossRef CAS PubMed.
- H. F. Liang, S. C. Chen, M. C. Chen, P. W. Lee, C. T. Chen and H. W. Sung, Bioconjugate Chem., 2006, 17, 291–299 CrossRef CAS PubMed.
- J. Sun, X. S. Chen, T. C. Lu, S. Liu, H. Y. Tian, Z. P. Guo and X. B. Jing, Langmuir, 2008, 24, 10099–10106 CrossRef CAS PubMed.
- C. Deng, X. S. Chen, H. J. Yu, J. Sun, T. C. Lu and X. B. Jing, Polymer, 2007, 48, 139–149 CrossRef CAS PubMed.
- T. Kean and M. Thanou, Adv. Drug Delivery Rev., 2010, 62, 3–11 CrossRef CAS PubMed.
- H. B. Li, R. Niu, J. L. Yang, J. Nie and D. Z. Yang, Carbohydr. Polym., 2011, 86, 1578–1585 CrossRef CAS PubMed.
- C. Lemarchand, R. Gref and P. Couvreur, Eur. J. Pharm. Biopharm., 2004, 58, 327–341 CrossRef CAS PubMed.
- G. Gregoriadis, S. Jain, I. Papaioannou and P. Laing, Int. J. Pharm., 2005, 300, 125–130 CrossRef CAS PubMed.
- G. Yogeeswaran and P. L. Salk, Science, 1981, 212, 1514–1516 CAS.
- U. Rutishauser, J. Cell. Biochem., 1998, 70, 304–312 CrossRef CAS.
- M. Muhlenhoff, M. Eckhardt and R. Gerardy-Schahn, Curr. Opin. Struct. Biol., 1998, 8, 558–564 CrossRef CAS.
- S. Jain, D. H. Hreczuk-Hirst, B. McCormack, M. Mital, A. Epenetos, P. Laing and G. Gregoriadis, Biochim. Biophys. Acta, Gen. Subj., 2003, 1622, 42–49 CrossRef CAS.
- A. I. Fernandes and G. Gregoriadis, Biochim. Biophys. Acta, Protein Struct. Mol. Enzymol., 1997, 1341, 26–34 CrossRef CAS.
- T. Ohyanagi, N. Nagahori, K. Shimawaki, H. Hinou, T. Yamashita, A. Sasaki, T. Jin, T. Iwanaga, M. Kinjo and S. I. Nishimura, J. Am. Chem. Soc., 2011, 133, 12507–12517 CrossRef CAS PubMed.
- L. Bondioli, L. Costantino, A. Ballestrazzi, D. Lucchesi, D. Boraschi, F. Pellati, S. Benvenuti, G. Tosi and M. A. Vandelli, Biomaterials, 2010, 31, 3395–3403 CrossRef CAS PubMed.
- G. Tosi, A. V. Vergoni, B. Ruozi, L. Bondioli, L. Badiali, F. Rivasi, L. Costantino, F. Forni and M. A. Vandelli, J. Controlled Release, 2010, 145, 49–57 CrossRef CAS PubMed.
- A. Surolia and B. K. Bachhawat, Biochim. Biophys. Acta, 1977, 497, 760–765 CrossRef CAS.
- T. M. Allen and A. Chonn, FEBS Lett., 1987, 223, 42–46 CrossRef CAS.
- T. M. Allen, C. Hansen and J. Rutledge, Biochim. Biophys. Acta, 1989, 981, 27–35 CrossRef CAS.
- A. Gabizon and D. Papahadjopoulos, Proc. Natl. Acad. Sci. U. S. A., 1988, 85, 6949–6953 CrossRef CAS.
- A. Chonn, S. C. Semple and P. R. Cullis, J. Biol. Chem., 1992, 267, 18759–18765 CAS.
- D. Liu, Y. K. Song and F. Liu, Pharm. Res., 1995, 12, 1775–1780 CrossRef CAS.
- Y. S. Park and L. Huang, Biochim. Biophys. Acta, 1993, 1166, 105–114 CrossRef CAS.
- M. C. Woodle, C. M. Engbers and S. Zalipsky, Bioconjugate Chem., 1994, 5, 493–496 CrossRef CAS.
- H. Yamauchi, T. Yano, T. Kato, I. Tanaka, S. Nakabayashi, K. Higashi, S. Miyoshi and H. Yamada, Int. J. Pharm., 1995, 113, 141–148 CrossRef CAS.
- H. Yamauchi, H. Kikuchi, K. Yachi, M. Sawada, M. Tomikawa and S. Hirota, Int. J. Pharm., 1993, 90, 73–79 CrossRef CAS.
- M. T. Michalek, E. G. Bremer and C. Mold, J. Immunol., 1988, 140, 1581–1587 CAS.
- P. A. Oldenborg, A. Zheleznyak, Y. F. Fang, C. F. Lagenaur, H. D. Gresham and F. P. Lindberg, Science, 2000, 288, 2051–2054 CrossRef CAS.
- F. P. Lindberg, D. M. Lublin, M. J. Telen, R. A. Veile, Y. E. Miller, H. Donis-Keller and E. J. Brown, J. Biol. Chem., 1994, 269, 1567–1570 CAS.
- K. Weiskopf, A. M. Ring, C. C. Ho, J. P. Volkmer, A. M. Levin, A. K. Volkmer, E. Ozkan, N. B. Fernhoff, M. van de Rijn, I. L. Weissman and K. C. Garcia, Science, 2013, 341, 88–91 CrossRef CAS PubMed.
- R. K. Tsai, P. L. Rodriguez and D. E. Discher, Blood Cells, Mol., Dis., 2010, 45, 67–74 CrossRef CAS PubMed.
- S. J. Stachelek, M. J. Finley, I. S. Alferiev, F. Wang, R. K. Tsai, E. C. Eckells, N. Tomczyk, J. M. Connolly, D. E. Discher, D. M. Eckmann and R. J. Levy, Biomaterials, 2011, 32, 4317–4326 CrossRef CAS PubMed.
- P. L. Rodriguez, T. Harada, D. A. Christian, D. A. Pantano, R. K. Tsai and D. E. Discher, Science, 2013, 339, 971–975 CrossRef CAS PubMed.
- C. M. Hu, L. Zhang, S. Aryal, C. Cheung, R. H. Fang and L. Zhang, Proc. Natl. Acad. Sci. U. S. A., 2011, 108, 10980–10985 CrossRef CAS PubMed.
- Z. D. Feng, L. Hensley, K. L. McKnight, F. Y. Hu, V. Madden, L. F. Ping, S. H. Jeong, C. Walker, R. E. Lanford and S. M. Lemon, Nature, 2013, 496, 367–371 CrossRef CAS PubMed.
- V. Ramakrishnaiah, C. Thumann, I. Fofana, F. Habersetzer, Q. W. Pan, P. E. de Ruiter, R. Willemsen, J. A. A. Demmers, V. S. Raj, G. Jenster, J. Kwekkeboom, H. W. Tilanus, B. L. Haagmans, T. F. Baumert and L. J. W. van der Laan, Proc. Natl. Acad. Sci. U. S. A., 2013, 110, 13109–13113 CrossRef CAS PubMed.
- C. M. Hu, R. H. Fang, B. T. Luk, K. N. Chen, C. Carpenter, W. Gao, K. Zhang and L. Zhang, Nanoscale, 2013, 5, 2664–2668 RSC.
- D. E. Owens 3rd and N. A. Peppas, Int. J. Pharm., 2006, 307, 93–102 CrossRef PubMed.
- C. M. Hu, R. H. Fang and L. Zhang, Adv. Healthcare Mater., 2012, 1, 537–547 CrossRef CAS PubMed.
- W. Gao, C. M. Hu, R. H. Fang, B. T. Luk, J. Su and L. Zhang, Adv. Mater., 2013, 25, 3549–3553 CrossRef CAS PubMed.
- A. Parodi, N. Quattrocchi, A. L. van de Ven, C. Chiappini, M. Evangelopoulos, J. O. Martinez, B. S. Brown, S. Z. Khaled, I. K. Yazdi, M. V. Enzo, L. Isenhart, M. Ferrari and E. Tasciotti, Nat. Nanotechnol., 2013, 8, 61–68 CrossRef CAS PubMed.
- S. Aryal, C. M. Hu, R. H. Fang, D. Dehaini, C. Carpenter, D. E. Zhang and L. Zhang, Nanomedicine, 2013, 8, 1271–1280 CrossRef CAS PubMed.
- R. H. Fang, C. M. Hu, K. N. Chen, B. T. Luk, C. W. Carpenter, W. Gao, S. Li, D. E. Zhang, W. Lu and L. Zhang, Nanoscale, 2013, 5, 8884–8888 RSC.
- C. M. Hu, R. H. Fang, J. Copp, B. T. Luk and L. Zhang, Nat. Nanotechnol., 2013, 8, 336–340 CrossRef CAS PubMed.
- S. Lakhal and M. J. Wood, BioEssays, 2011, 33, 737–741 CrossRef CAS PubMed.
- A. Kulp and M. J. Kuehn, Annu. Rev. Microbiol., 2010, 64, 163–184 CrossRef CAS PubMed.
- A. Lejeune, M. Moorjani, C. Gicquaud, J. Lacroix, P. Poyet and R. Gaudreault, Anticancer Res., 1994, 14, 915–919 CAS.
- N. E. Toledano Furman, Y. Lupu Haber, T. Bronshtein, L. Kaneti, N. Letko, E. Weinstein, L. Baruch and M. Machluf, Nano Lett., 2013, 13, 3248–3255 CrossRef CAS PubMed.
- K. Tang, Y. Zhang, H. Zhang, P. Xu, J. Liu, J. Ma, M. Lv, D. Li, F. Katirai, G. X. Shen, G. Zhang, Z. H. Feng, D. Ye and B. Huang, Nat. Commun., 2012, 3, 1282 CrossRef PubMed.
|
This journal is © The Royal Society of Chemistry 2014 |
Click here to see how this site uses Cookies. View our privacy policy here.