Tuning the magnetic behaviors in [FeIII12LnIII4] clusters with aromatic carboxylate ligands†
Received
9th November 2013
, Accepted 9th January 2014
First published on 4th February 2014
Abstract
A family of Fe12Ln4 clusters based on thiophene-3-carboxylic acid (3-TCA) or 3-methoxybenzoic acid (m-MOBA) were synthesized with a step-by-step strategy, namely [Fe12Ln4(μ4-O)6(μ3-O)4(μ3-OH)4(3-TCA)24] (Ln = La (1), Gd (3) and Dy (4)), {[Fe24Sm8(μ4-O)12(μ3-O)8(μ3-OH)8(3-TCA)46(NO3)2]·4CH3CN} (2), [Fe12La4(μ4-O)6(μ3-O)4(μ3-OH)4(m-MOBA)24] (5) and {[Fe12Sm4(μ4-O)6(μ3-O)4(μ3-OH)4(m-MOBA)24]·4CH3CN} (6). For each of the six complexes, two Fe4O2(OH)2 cubane units “sandwich” four FeIII centers to form Fe12O10(OH)4, and it is further connected to four LnIII ions through six μ4-O2− bridges to obtain a Fe12Ln4 core. Magnetic analyses indicate that 1–6 show different magnetic properties, and 2 and 6 show SMM-like behaviors, due to the differences in lanthanide ions and aromatic monocarboxylate ligands. Note that SmIII-containing 3d–4f clusters exhibiting SMM-like behavior are still rare in the documented cases.
Introduction
In recent years, cluster complexes and coordination polymers based on polynuclear clusters have drawn much attention from researchers, focusing on designing new functional molecular magnetic materials and metal–organic frameworks.1 Especially, considerable interest has been focused on employing a heterometallic approach to construct clusters and chain based compounds with fascinating magnetic phenomena,2 such as single-chain magnets (SCMs) behavior3 and single-molecule magnets (SMMs) behavior,4 which might be promising candidates for novel functional molecular magnetic materials.5 The synthesis of SMMs with larger energy barriers and higher blocking temperature has become an area of intensive research activity since the first discovery of SMMs in the 1990s.6 Usually, SMM behavior results from the favorable arrangement of metal ions with single ion anisotropy in a high-ground-spin state molecule.7 In the search for enhanced SMMs, the combination of 4f and 3d metal ions has been gradually receiving more and more attention.8 Additionally, the potential of iron-based systems has long been recognized by chemical researchers for the synthesis of magnetically interesting systems and SMMs.9 More recently, interesting and unusual magnetic effects have been observed in lanthanide complexes, especially those involving Tb and Dy because of their large anisotropy.2f,10 Thus, several FeIII–LnIII systems have been documented in order to incorporate favorable properties of both FeIII and LnIII ions (many examples for Ln = Tb/Dy).11
Bearing various bridging modes and magnetic exchange pathways between metal centers, carboxylate ligands have been widely used in the construction of 3d complexes with novel structures and interesting magnetic properties.12 However, pure carboxylate ligands are rarely used for building FeIII–LnIII clusters, due to the great challenge of the synthetic approach.4a,13 Inspired by a step-by-step strategy,13,14 classic iron-based precursors (Fe3O clusters) could be firstly synthesized; then Fe3O precursors as primary reactants react with lanthanide ions to facilitate the cluster formation. By this approach, we have obtained some new bridging networks affording interesting magneto-structural information. The Fe12Sm4 cluster constructed from benzoic acid displaying SMM behavior, which originates from the anisotropy of SmIII ions and is still rare in the documented cases, has been reported by our group.15 In this work, we chose thiophene-3-carboxylic acid (3-TCA) and 3-methoxybenzoic acid (m-MOBA) (Scheme 1) as primary ligands, because not only are they rigid ligands with carboxylate groups to construct high-nuclear clusters but also they are similar to benzoic acid in order to better understand the variations of magnetic behavior of the complexes.
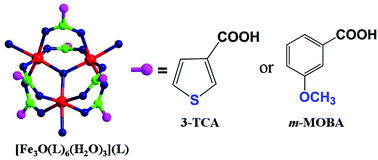 |
| Scheme 1 The structure core of Fe3O precursor constructed from 3-TCA or m-MOBA. | |
As part of our continuing studies on the synthesis and magnetic properties of Fe–Ln clusters,15 we report herein a family of Fe12Ln4 clusters from the reaction of 3-TCA/m-MOBA and metal ions of iron and lanthanide, namely [Fe12Ln4(μ4-O)6(μ3-O)4(μ3-OH)4(3-TCA)24] (Ln = La (1), Gd (3) and Dy (4)), {[Fe24Sm8(μ4-O)12(μ3-O)8(μ3-OH)8(3-TCA)46(NO3)2]·4CH3CN} (2), [Fe12La4(μ4-O)6(μ3-O)4(μ3-OH)4(m-MOBA)24] (5) and {[Fe12Sm4(μ4-O)6(μ3-O)4(μ3-OH)4(m-MOBA)24]·4CH3CN} (6). It is revealed that 2 and 6 show SMM-like behaviors. Interestingly, no SMM-like behaviour for 4 was observed despite the strong anisotropy of DyIII ions. To the best of our knowledge, SmIII-containing 3d–4f clusters exhibiting SMM-like behavior have been reported rarely so far.
Experimental
Materials and measurements
All the starting materials for synthesis were commercially available and used as received. [Fe3O(3-TCA)6(H2O)3](3-TCA) and [Fe3O(m-MOBA)6(H2O)3](m-MOBA) were prepared according to the procedures reported previously with small modifications.16 Elemental analyses (C, H and N) were performed on a Perkin-Elmer 240C analyzer (Perkin-Elmer, USA). IR spectra were measured on a TENSOR 27 OPUS FT-IR spectrometer using KBr disks dispersed with sample powders in the 4000–400 cm−1 range (Bruker, German). Magnetic data were collected using crystals of the samples on a Quantum Design MPMS-XL-7 SQUID magnetometer. The data were corrected using Pascal's constants to calculate the diamagnetic susceptibility, and experimental corrections for the sample holder were applied.
Synthesis of 1–6
[Fe12La4(μ4-O)6(μ3-O)4(μ3-OH)4(3-TCA)24] (1).
[Fe3O(3-TCA)6(H2O)3](3-TCA) (0.1 mmol) and La(NO3)3·6H2O (0.1 mmol) were added to 9 mL MeCN solution, stirred for 5 min and transferred to a 23 mL Teflon-lined autoclave and heated to 135 °C for 2 days. After cooling to room temperature, brown crystals were collected. The yield was ca. 20% based on La. Anal. calcd for C120H76Fe12S24O62La4 (%): C, 31.99; H, 1.70. Found (%): C, 31.62; H, 2.12. FT-IR (cm−1): 3420w, 3112w, 2170w, 1575m, 1520s, 1438s, 1357s, 878m, 832m, 759s, 694m.
{[Fe24Sm8(μ4-O)12(μ3-O)8(μ3-OH)8(3-TCA)46(NO3)2]·4CH3CN} (2).
A similar procedure as that for 1 was used for this complex except that La(NO3)3·6H2O was replaced by Sm(NO3)3·6H2O and the heating temperature was 125 °C. The yield was ca. 28% based on Sm. Anal. calcd for C238H158Fe24S46O126N6Sm8 (%): C, 31.28; H, 1.74; N, 0.92. Found (%): C, 31.79; H, 2.19; N, 1.36. FT-IR (cm−1): 3109w, 2366w, 2330w, 1579s, 1526m, 1438s, 1358s, 873w, 833w, 758s, 697w.
[Fe12Gd4(μ4-O)6(μ3-O)4(μ3-OH)4(3-TCA)24] (3).
A similar procedure as that for 1 was used for this complex except that La(NO3)3·6H2O was replaced by Gd(NO3)3·6H2O and the heating temperature was 140 °C. The yield was ca. 25% based on Gd. Anal. calcd for C120H76Fe12S24O62Gd4 (%): C, 31.48; H, 1.67. Found (%): C, 31.03; H, 2.20. FT-IR (cm−1): 3414w, 3118m, 1575s, 1523s, 1442s, 1351s, 1126m, 840m, 758s, 695m, 462m.
[Fe12Dy4(μ4-O)6(μ3-O)4(μ3-OH)4(3-TCA)24] (4).
A similar procedure as that for 1 was used for this complex except that La(NO3)3·6H2O was replaced by Dy(NO3)3·6H2O and the heating temperature was 135 °C. The yield was ca. 23% based on Dy. Anal. calcd for C120H76Fe12S24O62Dy4 (%): C, 31.34; H, 1.67. Found (%): C, 31.01; H, 2.09. FT-IR (cm−1): 3364s, 1617s, 1512s, 1462m, 1338s, 842m, 776m, 722m, 648m.
[Fe12La4(μ4-O)6(μ3-O)4(μ3-OH)4(m-MOBA)24] (5).
[Fe3O(m-MOBA)6(H2O)3](m-MOBA) (0.1 mmol) and La(NO3)3·6H2O (0.1 mmol) were added to 9 mL MeCN solution, stirred for 5 min and transferred to a 23 mL Teflon-lined autoclave and heated to 130 °C for 2 days. After cooling to room temperature, brown crystals were collected. The yield was ca. 23% based on La. Anal. calcd for C192H172Fe12O86La4 (%): C, 45.38; H, 3.41. Found (%): C, 44.97; H, 3.73. FT-IR (cm−1): 3418w, 3132w, 2366w, 1558 s, 1454m, 1406s, 1248m, 1124m, 1037m, 764s, 464m.
{[Fe12Sm4(μ4-O)6(μ3-O)4(μ3-OH)4(m-MOBA)24]·4CH3CN} (6).
A similar procedure as that for 5 was used for this complex except that La(NO3)3·6H2O was replaced by Sm(NO3)3·6H2O and the heating temperature was 140 °C. The yield was ca. ∼26% based on Sm. Anal. calcd for C199H181Fe12N4O86Sm4 (%): C, 45.30; H, 3.45; N, 1.06. Found (%): C, 44.61; H, 3.44; N, 0.59. FT-IR (cm−1): 3358s, 2843w, 2361m, 1567s, 1456s, 1410s, 1284m, 1249m, 1044m, 764s, 465m.
Crystallographic studies
X-ray single-crystal diffraction data for complexes 1–5 and 6 were collected on a SCX-Mini diffractometer at 293(2) K and a Rigaku RAXIS-RAPID diffractometer at 113(2) K with Mo-Kα radiation (λ = 0.71073 Å) by ω scan mode, respectively. The program CrystalClear17 was used for integration of the diffraction profiles. All the structures were solved by direct methods using the SHELXS program of the SHELXTL package and refined by full-matrix least-squares methods with SHELXL (semi-empirical absorption corrections were applied using SADABS program).18 Metal atoms in complexes 1–6 were located from E-maps and other non-hydrogen atoms were located in successive difference Fourier syntheses and refined with anisotropic thermal parameters on F2. The hydrogen atoms of the ligands were generated theoretically onto the specific atoms and refined isotropically with fixed thermal factors. The hydrogen atoms of hydroxyl groups were not assigned. Detailed crystallographic data are summarized in Table 1. CCDC 894850–894855 (complexes 1–6) contain the supplementary crystallographic data for this paper.
Table 1 Crystal data and structure refinement parameters for complexes 1–6
|
1
|
2
|
3
|
4
|
5
|
6
|
R
1 = ∑‖Fo| − |Fc‖/∑|Fo|.
wR2 = [∑[w(Fo2 − Fc2)2]/∑w(Fo2)2]1/2.
|
Chemical formula |
C120H76Fe12S24O62La4 |
C238H158Fe24S46N6O126Sm8 |
C120H76Fe12S24O62Gd4 |
C120H76Fe12S24O62Dy4 |
C192H172Fe12O86La4 |
C199H181Fe12N4O86Sm4 |
Formula weight |
4505.17 |
9135.66 |
4578.55 |
4599.55 |
5081.13 |
5276.08 |
Crystal system |
Monoclinic |
Triclinic |
Monoclinic |
Monoclinic |
Triclinic |
Triclinic |
Space group |
C2/c |
P![[1 with combining macron]](https://www.rsc.org/images/entities/char_0031_0304.gif) |
C2/c |
C2/c |
P![[1 with combining macron]](https://www.rsc.org/images/entities/char_0031_0304.gif) |
P![[1 with combining macron]](https://www.rsc.org/images/entities/char_0031_0304.gif) |
a (Å) |
29.727(6) |
17.441(4) |
29.688(6) |
29.630(6) |
17.982(4) |
17.790(3) |
b (Å) |
27.890(6) |
18.664(4) |
27.889(6) |
27.930(6) |
19.269(4) |
18.955(3) |
c (Å) |
20.138(4) |
28.858(6) |
20.013(4) |
19.955(4) |
19.494(4) |
19.512(4) |
α/° |
90 |
107.129(3) |
90 |
90 |
60.62(3) |
118.971(11) |
β/° |
95.33(3) |
91.4500(10) |
95.96(3) |
96.10(3) |
68.77(3) |
95.426(17) |
γ/° |
90 |
115.983(4) |
90 |
90 |
74.31(3) |
106.737(13) |
V/Å3 |
16 624(6) |
7939(3) |
16 480(6) |
16 421(6) |
5450.5(19) |
5293.0(16) |
Z
|
4 |
1 |
4 |
4 |
1 |
1 |
D
calcd/g cm−3 |
1.798 |
1.911 |
1.845 |
1.860 |
1.502 |
1.655 |
μ/mm−1 |
2.399 |
2.903 |
2.993 |
3.208 |
1.622 |
1.979 |
T/K |
293(2) |
293(2) |
293(2) |
293(2) |
293(2) |
113(2) |
R
int
|
0.1692 |
0.0339 |
0.1288 |
0.1438 |
0.1365 |
0.0434 |
R
[I > 2σ(I)] |
0.1216 |
0.0895 |
0.1023 |
0.1024 |
0.1045 |
0.0533 |
wRb[all data] |
0.2897 |
0.2400 |
0.2437 |
0.2149 |
0.2815 |
0.1623 |
Results and discussion
Synthesis considerations
A step-by-step strategy was employed to synthesize six new Fe12Ln4 clusters, which can guide the synthesis of high-nuclear 3d–4f clusters. In the solvothermal reaction, an anhydrous environment is necessary to successfully obtain target complexes. The starting material is a triangular Fe3O cluster, but it was transformed into Fe4O4 moieties in the final product, which may be facilitated by the addition of Ln(NO3)3. Besides the method in the Experimental section, we have tried a one-step method to construct target clusters. Unfortunately, no single crystals were afforded for structural analysis in our efforts to prepare the clusters. Notably, the synthetic temperatures and yields of 1–6 have some small differences and their stabilities are not very good.
Structural characterization
Complexes 1–4 and 5–6 are isostructural, respectively, with a similar structure as in the reported study15 by our group, although complex 2 consists of two different [Fe12Sm4] clusters, one containing 24 3-TCA ligands and another including 22 3-TCA ligands and 2 NO3− anions. Therefore, herein only the structure of 3 is described briefly. Single-crystal X-ray diffraction analysis reveals that complex 3 crystallizes in the C2/c space group. The asymmetric unit of 3 consists of six FeIII ions, two GdIII ions, three μ4-O2− anions, two μ3-O2− anions, two μ3-OH groups and twelve 3-TCA ligands. The ranges of Gd–O and Fe–O bond lengths are 2.323(10)–2.551(9) Å and 1.816(9)–2.111(10) Å, respectively, and O–Gd–O and O–Fe–O angles fall in the ranges of 67.5(3)–146.0(4)° and 80.9(4)–168.2(5)°. Each of the FeIII ions has a distorted octahedral coordination geometry and all of the GdIII ions are in a distorted square-antiprism fashion. As shown in Fig. 1a and 1c, two μ3-OH− and two O2− anions bridge four FeIII ions to form Fe4O2(OH)2 cubane units, and two of these cubane units “sandwich” four central FeIII ions via four μ3-O2− bridges. The connection of two cubane units and one central Fe4 unit forms a Fe12O10(OH)4 core along with the two Fe4O2(OH)2 cubane units. Further, four GdIII ions are linked to Fe12O10(OH)4 through six μ4-O2− anions, forming the Fe12Gd4 core of 3. In other words, the four GdIII ions are linked between the cubanes and the central Fe4 group. In addition, twenty η1:η1-μ2-3-TCA ligands and four η1:η2-μ3-3-TCA ligands occupy the periphery of the Fe12Gd4 core and complete the coordination spheres of the FeIII and GdIII centers by the O atoms of the carboxylate group. The molecular structure of 6 based on m-MOBA is shown in Fig. 1b.
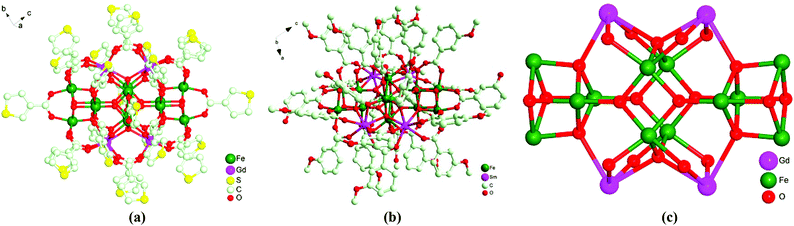 |
| Fig. 1 (a) The molecular structure of 3; (b) the molecular structure of 6; (c) the Fe12Gd4 core of 3. | |
Magnetic properties
Magnetic measurements were carried out on crystalline samples of 1–6 and their magnetic properties were investigated by solid state magnetic susceptibility measurements in the 2–300 K range at 1 kOe field and the isothermal field-dependent magnetizations M(H) at fields up to 50/70 kOe. The alternating current (ac) susceptibility measurements for 2, 4 and 6 were performed at low temperatures under Hdc = 0 Oe and Hac = 3.5 Oe for variable frequencies (from 1488 Hz to 3 Hz). Despite the fact that they contain a similar structure, they show varying magnetic behaviors as discussed below.
The magnetic properties in the form of χMT vs. T plots of 1–6 are shown in Fig. 2. Because 1 and 5 contain diamagnetic LaIII ions, they can be simplified as a Fe12 core from the magnetic point of view. For 1 and 5, the χMT values are 22.49 and 20.90 emu mol−1 K at 300 K, respectively, which are much lower than the expected value of 52.5 emu mol−1 K for twelve FeIII ions (S = 5/2, g = 2) suggesting dominant antiferromagnetic (AF) interactions between FeIII ions in 1 and 5.2f As the temperature decreases, the value of χMT slowly decreases down to a minimum value of 19.31 emu mol−1 K at 120 K for 1 and 19.11 emu mol−1 K at 140 K for 5, further indicating AF coupling between metal centres. Upon lowering the temperature to 3.5 K and 7 K, χMT abruptly increases to the maximum values (39.91 and 36.82 emu mol−1 K, respectively), showing ferromagnetic (F) interactions between FeIII ions in 1 and 5. Further cooling the temperature, χMT decreases down to the minimum values of 39.31 and 34.47 emu mol−1 K at 2 K. It is noteworthy that in the temperature range 2–300 K both F and AF interactions exist in the Fe12 core of 1 and 5 but at a specific temperature only one is dominant given that the Fe–O–Fe angles are about 95° and 130°.
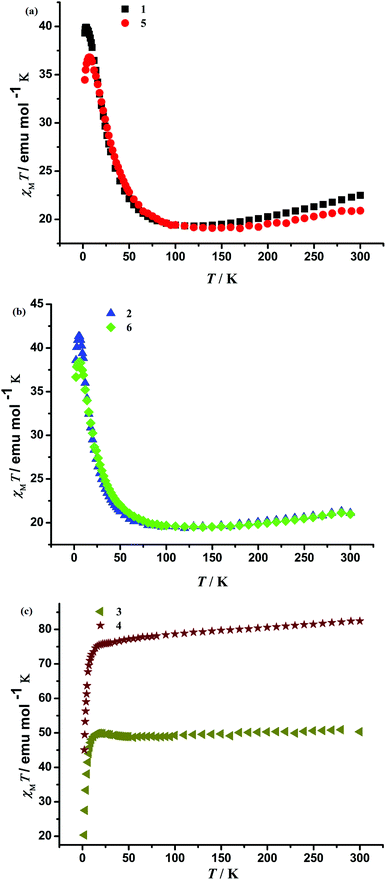 |
| Fig. 2 Temperature dependence of magnetic susceptibilities in the forms of χMT at an applied field of 1 kOe for 1–6. | |
For 2 and 6, the values for χMT at 300 K are 21.12 and 20.99 emu mol−1 K, much lower than the theoretical value of 52.86 emu mol−1 K for four SmIII (6H5/2, L = 5, g = 2/7) and twelve FeIII ions,15,19 which indicates strong AF coupling between metal centers. The χMT values decrease gradually until 95 and 140 K, reaching 19.55 and 19.51 emu mol−1 K, respectively. When the temperature decreases, the χMT values increase, reaching maximum values of 41.32 and 38.40 emu mol−1 K at 5 and 6 K, respectively. The χMT products then decrease with a further lowering of the temperature and attain values of 38.59 and 36.68 emu mol−1 K at 2 K because of zero-field splitting and/or AF interaction among Fe12Sm4 clusters. As a result, both F and AF interactions exist between metal ions.
For 3, the value for χMT at room temperature is 50.32 emu mol−1 K, much lower than the theoretical value of 84.02 emu mol−1 K for four GdIII (8H7/2, L = 0, g = 2) and twelve FeIII ions, which indicates strong AF coupling between metal centers. Upon cooling, the χMT value remains nearly constant, reaching 49.89 emu mol−1 K at 18 K, and then decreases rapidly to a value of 20.35 emu mol−1 K at 2 K. This is because dominant AF interaction among Fe12Gd4 clusters exists in 3. For 4, the value for χMT at room temperature is 82.45 emu mol−1 K, lower than the theoretical value of 109.18 emu mol−1 K for four DyIII (6H15/2, L = 5, g = 3/4) and twelve FeIII ions, which indicates strong AF coupling between metal ions. Upon cooling, the χMT value linearly decreases, reaching 75.84 emu mol−1 K at 22 K, and then decreases rapidly to a value of 45.07 emu mol−1 K at 2 K. This is attributed to dominant AF interaction among Fe12Dy4 clusters.
The M vs. H curves (at 2 K) for 1–6 are shown in Fig. 3. For 1, the magnetization at 2 K tends to saturation values of 17.37Nβ at 50 kOe and the experimental magnetization curve is below the red line that presents the Brillouin function for S = 8 ground state with g = 2.1, confirming again the existence of AF coupling between the FeIII ions (Fig. S1, ESI†). The magnetization for 5 is similar to that for 1. For 2 and 6, M increases quickly at very low field. In the high field region the increase of magnetization is slow, reaching 19.03 and 19.09Nβ at 50 kOe, which are consistent with the theoretical saturated value of 18.89Nβ. For 3, M increases linearly at low field and reaches 46.19Nβ at 50 kOe, which is a little higher than the theoretical value of 44.04Nβ. The M vs. H curve of 4 is similar to that of 3. The magnetizations for 2, 3, 4 and 6 at 2 K tend to saturation values, which may be ascribed to weak AF interactions between FeIII ions and LnIII ions.
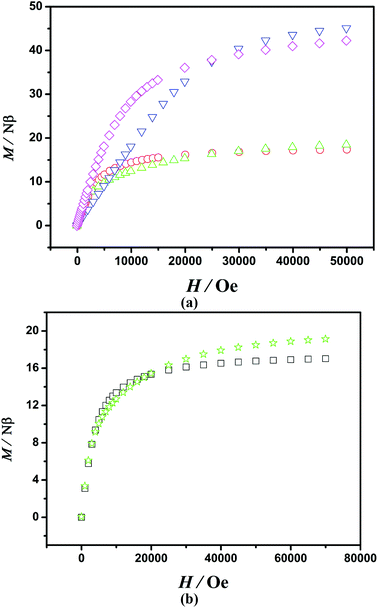 |
| Fig. 3 The M vs. H plots at 2 K for 1 ( ), 2 ( ), 3 ( ), 4 ( ), 5 (□) and 6 ( ). | |
Table 2 shows susceptibility data at 300 K for the Fe12Ln4 series of 1–6. ΔχMT, the contribution to the χMT value of the Ln4 part in Fe12Ln4, is calculated as χMT (Fe12Ln4) – χMT (1 or 5). For 2, the ΔχMT value is −1.37 emu mol−1 K, which indicates AF coupling between SmIII ions and FeIII ions at 300 K. For 3 and 4, the ΔχMT values at 300 K are far below than that expected for a Fe12 unit and four GdIII/DyIII ions, indicating that the magnetic exchange interactions between the GdIII/DyIII and FeIII ions are very weak. For 6, the ΔχMT value is almost zero, which suggests that the magnetic interactions between GdIII and GdIII/FeIII ions at room temperature are negligible.
Table 2 Susceptibility data for the Fe12Ln4 series of 1–6. Units: emu mol−1 K
|
Fe12La4 (1) |
Fe12Sm4 (2) |
Fe12Gd4 (3) |
Fe12Dy4 (4) |
Fe12La4 (5) |
Fe12Sm4 (6) |
ΔχMT (2/3/4) = χMT (Fe12Ln4) − χMT (Fe12La4, 1) of experimental values. ΔχMT (6) = χMT (Fe12Sm4, 6) − χMT (Fe12La4, 5).
|
4 × (Ln) contribution |
— |
1.44 |
31.5 |
56.68 |
— |
1.44 |
Theoretical χMT (300 K) |
52.5 |
53.9 |
84.0 |
109.18 |
52.5 |
53.9 |
Experimental χMT (300 K) |
22.49 |
21.12 |
50.32 |
82.45 |
20.90 |
20.99 |
ΔχMTa |
— |
−1.37 |
+27.83 |
+59.96 |
— |
+0.09 |
In order to elucidate possible SMM behavior, ac measurements for 2, 4 and 6 were performed. ac susceptibility measurements for 2 and 6 indicate that whereas the in-phase curves (χ′) at high temperature are almost consistent above 3 K, peaks arise below 3 K, and a frequency dependent out-of-phase signal appears, indicating a slow relaxation behavior of the magnetization (Fig. 4). However, the maximum value of χ′′ was not observed even at the low temperature (2 K) and high frequency (1488 Hz) due to the limit of the magnetometer. The pre-exponential factor (τ0) and energy barrier (U) to reverse the magnetization can be roughly estimated from the ln(χ′′M/χ′M) vs. 1/T plot at 997 Hz for 2 and 1488 Hz for 6 by considering a single relaxation time (Fig. S2, ESI†). The least-squares fitting of the experimental data through the expression χ′′M/χ′M = 2πυτ0 exp(U/kBT) gave τ0 ≈ 5.9 × 10−8 s and U ≈ 9.8 K for 2, and τ0 ≈ 1.0 × 10−7 s and U ≈ 8.7 K for 6, respectively. The ac signals suggest the prevalence of quantum tunnelling effect at low temperature. Due to the absence of out-of-phase ac signals in 1 and 5 (Fig. S3, ESI†), the slow magnetic relaxation in 2 and 6 can be attributed to magnetic anisotropy induced by the introduction of SmIII ions with unquenched spin–orbit coupling.
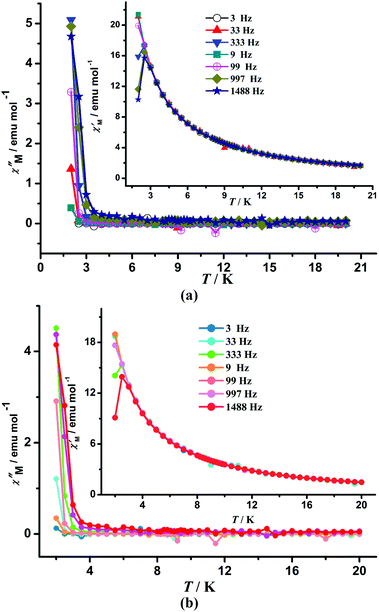 |
| Fig. 4 Temperature dependence of the ac χM at different frequencies with Hdc = 0 Oe for 2 (a) and 6 (b). | |
Because slow relaxation of magnetization is experimentally observed only over a short range of temperatures and no maximum of χ′′ is found at the low temperatures technically available, the estimation of these characteristic parameters might not be very accurate, but τ0 values are consistent with the expected values (τ0 = 10−6–10−11 s) for SMMs.20 ac susceptibility measurement for 4 indicates that whereas all the in-phase curves (χ′) are almost consistent without peaks, a weak frequency dependent out-of-phase signal appears, indicating a slow relaxation behavior of the magnetization (Fig. S4, ESI†). The energy barrier and characteristic relaxation time could not be obtained because the maximum value of χ′′ was not found due to the 2 K temperature limit of the instrument. Therefore, slow magnetic relaxation behaviors for 2, 4 and 6 were observed, and 2 and 6 exhibit SMM-like properties. Notably, SmIII-containing complexes displaying SMM-like behaviors are rare because the anisotropy of SmIII ions is weaker than that of other anisotropic lanthanide ions such as TbIII and DyIII ions. Additionally, compared with the first SmIII SMM Fe12Sm4 cluster derived from benzoic acid,15 complexes 2 and 6 exhibit SMM-like behaviors because of the relatively high measurement temperatures (above 2 K).
Conclusions
In summary, we present here six new Fe12Ln4 clusters synthesized with a step-by-step strategy. Varying magnetic properties of the six complexes may result from distinct monocarboxylate ligands and 4f metal ions. ac magnetic measurements show frequency dependence of the out-of-phase magnetic susceptibility (χ′′) suggesting slow relaxation of the magnetization for 2, 4 and 6. No obvious χ′′ peak but strong field-dependent χ′ and χ′′ signals can be found, suggesting SMM-like properties for 2 and 6, and the existence of zero-field quantum tunnelling at low temperature. Therefore, the studied series of Fe12Ln4 clusters provides a good example for the construction of high-nuclear Fe–Ln clusters with different magnetic behaviors.
Acknowledgements
This work was financially supported by NSFC (21371102 and 21003078), the NSF of Tianjin, China (11JCYBJC04100) and the Foundation of State Key Laboratory of Coordination Chemistry.
Notes and references
-
(a) S. Xiang, Y. He, Z. Zhang, H. Wu, W. Zhou, R. Krishna and B. Chen, Nat. Commun., 2012, 3, 954 CrossRef PubMed;
(b) Q. Chen, Z. Chang, W. C. Song, H. Song, H. B. Song, T. L. Hu and X. H. Bu, Angew. Chem., Int. Ed., 2013, 52, 11550–11553 CrossRef CAS PubMed;
(c) S. D. Han, W. C. Song, J. P. Zhao, Q. Yang, S. J. Liu, Y. Li and X. H. Bu, Chem. Commun., 2013, 49, 871–873 RSC;
(d) Z. B. Han, R. Y. Lu, Y. F. Liang, Y. L. Zhou, Q. Chen and M. H. Zeng, Inorg. Chem., 2012, 51, 674–679 CrossRef PubMed;
(e) R. J. Wei, Q. Huo, J. Tao, R. B. Huang and L. S. Zheng, Angew. Chem., Int. Ed., 2011, 50, 8940–8943 CrossRef PubMed;
(f) J. P. Tong, F. Shao, J. Tao, R. B. Huang and L. S. Zheng, Inorg. Chem., 2011, 50, 2067–2069 CrossRef CAS PubMed.
-
(a) R. Sessoli and A. K. Powell, Coord. Chem. Rev., 2009, 253, 2328–2341 CrossRef PubMed;
(b) M. Mannini, F. Pineider, P. Sainctavit, C. Danieli, E. Otero, C. Sciancalepore, A. M. Talarico, M. A. Arrio, A. Cornia, D. Gatteschi and R. Sessoli, Nat. Mater., 2009, 8, 194–197 CrossRef PubMed;
(c) F. Pointillart, K. Bernot, R. Sessoli and D. Gatteschi, Chem.–Eur. J., 2007, 13, 1602–1609 CrossRef PubMed;
(d) W. G. Wang, A. J. Zhou, W. X. Zhang, M. L. Tong, X. M. Chen, M. Nakano, C. C. Beedle and D. N. Hendrickson, J. Am. Chem. Soc., 2007, 129, 1014–1015 CrossRef PubMed;
(e) P. L. Feng, C. C. Beedle, C. Koo, W. Wernsdorfer, M. Nakano, S. Hill and D. N. Hendrickson, Inorg. Chem., 2008, 47, 3188–3204 CrossRef PubMed;
(f) A. Baniodeh, I. J. Hewitt, V. Mereacre, Y. H. Lan, G. Novitchi, C. E. Anson and A. K. Powell, Dalton Trans., 2011, 40, 4080–4086 RSC;
(g) S. J. Liu, W. C. Song, L. Xue, S. D. Han, Y. F. Zeng, L. F. Wang and X. H. Bu, Sci. China: Chem., 2012, 55, 1064–1072 CrossRef CAS;
(h) S. D. Jiang, B. W. Wang, H. L. Sun, Z. M. Wang and S. Gao, J. Am. Chem. Soc., 2011, 133, 4730–4733 CrossRef CAS PubMed;
(i) L. Q. Wei, K. Zhang, Y. C. Feng, Y. H. Wang, M. H. Zeng and M. Kurmoo, Inorg. Chem., 2011, 50, 7274–7283 CrossRef CAS PubMed.
-
(a) T. S. Venkatakrishnan, S. Sahoo, N. Bréfuel, C. Duhayon, C. Paulsen, A. L. Barra, S. Ramasesha and J. P. Sutter, J. Am. Chem. Soc., 2010, 132, 6047–6056 CrossRef CAS PubMed;
(b) I. Bhowmick, E. A. Hillard, P. Dechambenoit, C. Coulon, T. D. Harris and R. Clérac, Chem. Commun., 2012, 48, 9717–9719 RSC;
(c) J. Ferrando-Soria, D. Cangussu, M. Eslava, Y. Journaux, R. Lescouëzec, M. Julve, F. Lloret, J. Pasán, C. Ruiz-Pérez, E. Lhotel, C. Paulsen and E. Pardo, Chem.–Eur. J., 2011, 17, 12482–12494 CrossRef CAS PubMed.
-
(a) V. M. Mereacre, A. M. Ako, R. Clérac, W. Wernsdorfer, G. Filoti, J. Bartolomé, C. E. Anson and A. K. Powell, J. Am. Chem. Soc., 2007, 129, 9248–9249 CrossRef CAS PubMed;
(b) G. Novitchi, W. Wernsdorfer, L. F. Chibotaru, J. P. Costes, C. E. Anson and A. K. Powell, Angew. Chem., Int. Ed., 2009, 48, 1614–1619 CrossRef CAS PubMed;
(c) A. Okazawa, T. Nogami, H. Nojiri and T. Ishida, Inorg. Chem., 2008, 47, 9763–9765 CrossRef CAS PubMed;
(d) Y. Y. Zhu, C. Cui, Y. Q. Zhang, J. H. Jia, X. Guo, C. Gao, K. Qian, S. D. Jiang, B. W. Wang, Z. M. Wang and S. Gao, Chem. Sci., 2013, 4, 1802–1806 RSC.
-
(a) S. Nayak, O. Roubeau, S. J. Teat, C. M. Beavers, P. Gamez and J. Reedijk, Inorg. Chem., 2010, 49, 216–221 CrossRef CAS PubMed;
(b) X. J. Kong, Y. P. Ren, W. X. Chen, L. S. Long, Z. P. Zheng, R. B. Huang and L. S. Zheng, Angew. Chem., Int. Ed., 2008, 47, 2398–2401 CrossRef CAS PubMed;
(c) X. J. Kong, Y. P. Ren, L. S. Long, Z. P. Zheng, R. B. Huang and L. S. Zheng, J. Am. Chem. Soc., 2007, 129, 7016–7017 CrossRef CAS PubMed;
(d) C. Benelli and D. Gatteschi, Chem. Rev., 2002, 102, 2369–2388 CrossRef CAS PubMed.
-
(a) T. C. Stamatatos, S. J. Teat, W. Wernsdorfer and G. Christou, Angew. Chem., Int. Ed., 2009, 48, 521 CrossRef CAS PubMed;
(b) I. R. Jeon and R. Clérac, Dalton Trans., 2012, 41, 9569–9586 RSC;
(c) J. W. Sharples, Y. Z. Zheng, F. Tuna, E. J. L. McInnes and D. Collison, Chem. Commun., 2011, 47, 7650–7652 RSC;
(d) Y. L. Miao, J. L. Liu, J. Y. Li, J. D. Leng, Y. C. Ou and M. L. Tong, Dalton Trans., 2011, 40, 10229–10236 RSC;
(e) Y. N. Guo, G. F. Xu, W. Wernsdorfer, L. Ungur, Y. Guo, J. K. Tang, H. J. Zhang, L. F. Chibotaru and A. K. Powell, J. Am. Chem. Soc., 2011, 133, 11948–11951 CrossRef CAS PubMed.
-
(a) H. L. C. Feltham, R. Clérac, A. K. Powell and S. Brooker, Inorg. Chem., 2011, 50, 4232–4234 CrossRef CAS PubMed;
(b) A. Mishra, A. J. Tasiopoulos, W. Wernsdorfer, K. A. Abboud and G. Christou, Inorg. Chem., 2007, 46, 3105–3115 CrossRef CAS PubMed;
(c) S. K. Langley, L. Ungur, N. F. Chilton, B. Moubaraki, L. F. Chibotaru and K. S. Murray, Chem.–Eur. J., 2011, 17, 9209–9218 CrossRef CAS PubMed;
(d) T. Kajiwara, M. Nakano, K. Takahashi, S. Takaishi and M. Yamashita, Chem.–Eur. J., 2011, 17, 196–205 CrossRef CAS PubMed.
-
(a) V. Mereacre, A. Baniodeh, C. E. Anson and A. K. Powell, J. Am. Chem. Soc., 2011, 133, 15335–15337 CrossRef CAS PubMed;
(b) V. Mereacre, Y. H. Lan, R. Clérac, A. M. Ako, W. Wernsdorfer, G. Buth, C. E. Anson and A. K. Powell, Inorg. Chem., 2011, 50, 12001–12009 CrossRef CAS PubMed;
(c) C. Aronica, G. Pilet, G. Chastanet, W. Wernsdorfer, J. F. Jacquot and D. Luneau, Angew. Chem., Int. Ed., 2006, 45, 4659–4662 CrossRef CAS PubMed.
-
(a) D. Gatteschi, R. Sessoli and A. Cornia, Chem. Commun., 2000, 725–732 RSC;
(b) R. Bagai, M. R. Daniels, K. A. Aboud and G. Christou, Inorg. Chem., 2008, 47, 3318–3327 CrossRef CAS PubMed;
(c) L. F. Jones, A. Batsanov, E. K. Brechin, D. Collison, M. Helliwell, T. Mallah, E. J. L. McInnes and S. Piligkos, Angew. Chem., Int. Ed., 2002, 41, 4318–4321 CrossRef CAS;
(d) L. F. Jones, P. Jensen, B. Moubaraki, K. J. Berry, J. F. Boas, J. R. Pibrow and K. S. Murray, J. Mater. Chem., 2006, 16, 2690–2697 RSC;
(e) D. M. Low, L. F. Jones, A. Bell, E. K. Brechin, T. Mallah, E. Riviére, S. J. Teat and E. J. L. McInnes, Angew. Chem., Int. Ed., 2003, 42, 3781–3784 CrossRef CAS PubMed;
(f) H. Oshio, N. Hoshino and T. Ito, J. Am. Chem. Soc., 2000, 122, 12602–12603 CrossRef CAS.
-
(a) Y. Z. Zheng, Y. H. Lan, C. E. Anson and A. K. Powell, Inorg. Chem., 2008, 47, 10813–10815 CrossRef CAS PubMed;
(b) P. H. Lin, T. J. Burchell, L. Ungur, L. F. Chibotaru, W. Wernsdorfer and M. Murugesu, Angew. Chem., Int. Ed., 2009, 48, 9489–9492 Search PubMed;
(c) S. J. Liu, J. P. Zhao, W. C. Song, S. D. Han, Z. Y. Liu and X. H. Bu, Inorg. Chem., 2013, 52, 2103–2109 CrossRef CAS PubMed.
-
(a) V. Mereacre, D. Prodius, Y. H. Lan, C. Turta, C. E. Anson and A. K. Powell, Chem.–Eur. J., 2011, 17, 123–128 CrossRef CAS PubMed;
(b) S. Mukherjee, M. R. Daniels, R. Bagai, K. A. Abboud, G. Christou and C. Lampropoulos, Polyhedron, 2010, 29, 54–65 CrossRef CAS PubMed.
-
(a) Y. Q. Wang, X. M. Zhang, X. B. Li, B. W. Wang and E. Q. Gao, Inorg. Chem., 2011, 50, 6314–6322 CrossRef CAS PubMed;
(b) Y. W. Li, J. P. Zhao, L. F. Wang and X. H. Bu, CrystEngComm, 2011, 13, 6002–6006 RSC;
(c) Y. W. Li, J. R. Li, L. F. Wang, B. Y. Zhou, Q. Chen and X. H. Bu, J. Mater. Chem. A, 2013, 1, 495–499 RSC.
- A. M. Ako, V. Mereacre, R. Clérac, I. J. Hewitt, Y. H. Lan, C. E. Anson and A. K. Powell, Dalton Trans., 2007, 5245–5247 RSC.
- D. Schray, G. Abbas, Y. H. Lan, V. Mereacre, A. Sundt, J. Dreiser, O. Waldmann, G. E. Kostakis, C. E. Anson and A. K. Powell, Angew. Chem., Int. Ed., 2010, 49, 5185–5188 CrossRef CAS PubMed.
- Y. F. Zeng, G. C. Xu, X. Hu, Z. Chen, X. H. Bu, S. Gao and E. C. Sañudo, Inorg. Chem., 2010, 49, 9734–9736 CrossRef CAS PubMed.
- R. F. Weinland and A. Herz, Ber. Dtsch. Chem. Ges., 1912, 45, 2662 CrossRef.
-
Rigaku, Process-Auto, Rigaku Americas Corporation, The Woodlands, Texas, 1998 Search PubMed.
-
G. M. Sheldrick, SHELXTL NT Version 5.1. Program for Solution and Refinement of Crystal Structures, University of Göttingen, Germany, 1997 Search PubMed.
- S. Sanz, K. Ferreira, R. D. McIntosh, S. J. Dalgarno and E. K. Brechin, Chem. Commun., 2011, 47, 9042–9044 RSC.
-
(a) B. Joarder, A. K. Chaudhari, G. Rogez and S. K. Ghosh, Dalton Trans., 2012, 41, 7695–7699 RSC;
(b) Y. D. Luo, G. M. Sun, D. M. Li and F. Luo, Inorg. Chem. Commun., 2011, 14, 778–785 CrossRef CAS PubMed;
(c) Z. H. Zhang, Y. Song, T. A. Okamura, Y. Hasegawa, W. Y. Sun and N. Ueyama, Inorg. Chem., 2006, 45, 2896–2902 CrossRef CAS PubMed.
Footnote |
† Electronic supplementary information (ESI) available. CCDC 894850–894855. For ESI and crystallographic data in CIF or other electronic format see DOI: 10.1039/c3qi00083d |
|
This journal is © the Partner Organisations 2014 |
Click here to see how this site uses Cookies. View our privacy policy here.