Four-connected metal–organic frameworks constructed by tetracarboxylate acid-based ligands†
Received
2nd April 2014
, Accepted 28th May 2014
First published on 30th May 2014
Abstract
Three tetracarboxylate acid ligands, 5,5′-(1H-1,2,3-triazole-1,4-diyl)diisophthalic acid (H4TADIP), 5-(bis(4-carboxybenzyl)amino)isophthalic acid (H4BCBAIP), and 5-(3,5-dicarboxybenzyloxy)isophthalic acid (H4DBIP) have been reacted with Mn(II), Co(II) and In(III) salts to construct four new metal–organic frameworks, [Mn2(TADIP)(DMF)3]·2DMF·3H2O (1), [Co2(TADIP)(DEF)3]·0.5DEF (2), [Mn2(BCBAIP)(DMF)3]·7.5DMF (3), and (Me2NH2)[In(DBIP)]·3DMF·7H2O (4) under solvothermal conditions. Due to the rotation ability of these ligands that exhibit a quadrangle or tetrahedral geometry, these compounds show diverse three-dimensional four-connected topologies. Compound 1 is composed of binuclear Mn(II) units and quadrangle type ligand H4TADIP with pts topology, while compound 2 possesses a zeolitic abw topology constructed from the 4-connected tetrahedral type ligand H4TADIP and binuclear Co(II) units. Compound 3 is a 3D structure possessing a zeolitic sod topology from the coordination of binuclear Mn(II) units with the tetrahedral type ligand H4BCBAIP. Compound 4 is an anionic framework constructed from tetrahedral type ligand H4DBIP and In(III) ions that has a topology of unc. Variable temperature magnetic susceptibility measurements show that compound 1 exhibits antiferromagnetic behavior. The selective dye-adsorption and luminescent properties of compound 4 have been studied in detail.
Introduction
In recent years, considerable effort has been focused on metal–organic frameworks (MOFs) due to not only their aesthetic structures, but also their versatile applications such as gas storage and separation,1 catalysis,2 magnetism,3 luminescence,4 and so on. Some recent excellent reviews have deeply discussed all aspects of the development and progress in the last two decades.5 Among these studies, development of new MOFs with diverse structures has always drawn great attention. The common strategy of crystal engineering has parted the structures into inorganic secondary building units (I-SBUs) and organic secondary building units (O-SBUs). I-SBUs can be thought as “joint” and O-SBUs as “strut”.6 The rational combination of I-SBUs and O-SBUs has been an important strategy in the design, prediction and synthesis of diverse MOFs.
Setting aside I-SBUs, which are too complicated to describe,7 organic linkers that can be regarded as O-SBUs participate in the construction of frameworks. Through selection of organic linkers with different geometries (2-, 3-, and 4-connected ligands can be thought as linear, triangular, planar quadrangular or tetrahedral, respectively), a variety of MOFs with different topologies and properties have been synthesized. In the selection of organic linkers, 4-connected tetracarboxylate ligands with rigid plane quadrangle or tetrahedral geometry are widely employed.8 For example, in 2005 Chen et al. reported the synthesis of MOF-505 for the first time by utilization of 4-connected tetracarboxylate ligand H4BPTC (H4BPTC = 3,3′,5,5′-biphenyltetracarboxylic acid) to react with a copper salt.9 Such a MOF has a topology of nbo and exhibits good adsorption behavior to H2. Schröder and coworkers developed a series of tetracarboxylate ligands with expansion of the length, and obtained a number of MOFs with different structures, which have good properties in gas adsorption.10 Some other groups also reported MOFs synthesized with 4-connected tetracarboxylate ligands based on isophthalic acid.11 However, most works reported up to now have made use of 4-connected ligands to react with Cu, Zn or In salts to form MOFs with topologies of nbo, pts, fcu, etc. From the crystal engineering point of view, many more structures might be built by rational exploitation of 4-connected I-SBU and O-SBU, so there remain lots of MOFs to be constructed when considering the features of these SBUs.
On the other hand, zeolite-like MOFs (ZMOFs) with sod, rho, and mtn topologies have been explored these years and found their applications in lots of areas.12 In order to build ZMOFs, research mainly focused on the utilization of imidazole, imidazole carboxylic acid and heterocyclic carboxylic acid by the judicious mimicking of the Si–O–Si bond angle of about 145° through the employment of L–M–L angles.13 Recently, Zheng et al. reported another synthetic strategy for the creation of ZMOFs using carboxylic acid.14 It is not surprising that ZMOFs can be constructed by the linkage of 4-connected I-SBU and O-SBU through direct coordination interaction. Feng and coworkers reported several ZMOFs with sod and rho topologies based on 4-connected boron imidazolate complexes and 4-connected Li+ or Cu+ ions.12e,13c However, reports are still rare on the construction of ZMOFs with 4-connected tetracarboxylate acid (Scheme 1).
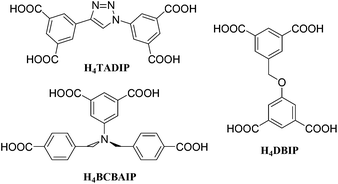 |
| Scheme 1 Representation of the three ligands used in this work. | |
Recently, we have paid great attention to the design and synthesis of MOFs with novel ligands based on isophthalic acid.15,16 Some of the MOFs formed exhibited sensing behaviors for the detection of metal ions and nitroaromatic explosives.15b,c We have designed and synthesized a ZMOF with sod topology through the 4 + 4 synthetic strategy by cross-linking the designed 4-connected tetracarboxylate ligand with the 4-connected In3+ ion.16 Here, we use three tetracarboxylate acid ligands, 5,5′-(1H-1,2,3-triazole-1,4-diyl)diisophthalic acid (H4TADIP), 5-(bis(4-carboxybenzyl)amino)isophthalic acid (H4BCBAIP) and 5-(3,5-dicarboxybenzyloxy)isophthalic acid (H4DBIP) to react with Mn(II), Co(II) and In(III) salts to construct diverse structures. As mentioned in previous work, for ligand H4TADIP,15b,17 the two isophthalic acids linked to the 1,2,3-triazole ring can rotate with the C–N and C–C single bonds in some content, which can make the ligand act as two different geometries, i.e. planar quadrangular and tetragonal, and these can potentially lead to a number of different structures with metal ions. In ligand H4BCBAIP, the free rotation of the C–N single bond may introduce a ligand with tetrahedral configuration. As for ligand H4DBIP, Cao and others have reported the usage of such a ligand for the preparation of MOFs with diverse topologies.11j,k We present here four MOFs based on these three tetracarboxylate acid ligands, [Mn2(TADIP)(DMF)3]·DMF (1), [Co2(TADIP)(DEF)3]·DEF (2), [Mn2(BCBAIP)(DMF)3]·7.5DMF (3), and (Me2NH2)[In(DBIP)]·3DMF·7H2O (4). Compound 1 has a pts topology, while 2 has a zeolitic abw topology. Compound 3 possesses a zeolitic sod topology, and compound 4 has an unc topology.
Experimental
Materials and physical measurements
All reagents were purchased commercially and used without further purification. H4TADIP,15bH4BCBAIP16 and H4DBIP15e were synthesized according to the procedures reported by our group previously. Thermogravimetric analyses (TGA) were performed under air atmosphere from room temperature to 800 °C with a heating rate of 10 °C min−1 on TGA Q500 thermogravimetric analyzer. Elemental analyses (C, H and N) were carried out with a vario MICRO elemental analyzer. The infrared (IR) spectra were recorded from 400 to 4000 cm−1 on a Nicolet Impact 410 FTIR spectrometer using KBr pellets. Powder X-ray diffraction (PXRD) data were collected on a Rigaku D-Max 2550 diffractometer with Cu-Kα radiation (λ = 1.5418 Å) over the 2θ range of 4–40° at room temperature. Temperature dependent magnetic susceptibility data were recorded on a Quantum-Design MPMS-XL SQUID magnetometer under an applied field of 1 kOe over the temperature range of 2–300 K. Photoluminescent spectra were obtained with a RF-5301 PC spectrometer. The solid state UV-vis absorption spectra were taken on a HITACHI U-4100 spectrophotometer while the liquid spectra were taken on a SHIMADZU UV-2450 UV-visible spectrophotometer.
Single-crystal X-ray studies
Single-crystal X-ray diffraction measurements were carried out on a Rigaku R-AXIS RAPID diffractometer for compound 1 and a Bruker Apex II CCD diffractometer for compounds 2–4, with graphite monochromated Mo Kα (λ = 0.71073 Å) radiation at 293 or 296 K. Data processing was accomplished with the SAINT processing program.18 The structures of these compounds were solved by direct method and refined on F2 by full-matrix least-squares with the SHELEX-97 program.19 For compound 1, all non-hydrogen atoms were refined with anisotropic displacement parameters except the C and N atoms of coordinated DMF molecules. Some restraints like dfix were used on the DMF molecules for a better geometry configuration. The hydrogen atoms on the aromatic rings were placed geometrically with isotropic thermal parameters 1.2 times those of the attached carbon atoms. As for compound 2, all non-hydrogen atoms were refined with anisotropic displacement parameters except the C and N atoms of coordinated DMF molecules. Some restraints like dfix were used for a better geometry configuration. For compound 3, the C25 was split to C25 and C25′. All non-hydrogen atoms on the backbone were refined with anisotropic displacement parameters except the terminal DMF molecules. The restraint dfix was used to the terminal DMF molecules for a better geometry. For compound 4, all non-hydrogen atoms were refined with anisotropic displacement parameters (except for C9, C9′, O9 and O9′ in compound 4). Some restraints like exyz, eadp and dfix were used to refine the frameworks. Attempts to locate and model the highly disordered solvent molecules in the pores were unsuccessful. Therefore, further refinement with the SQUEEZE routine of PLATON was used to remove the diffraction contribution from guests to produce a set of solvent-free diffraction intensities.20 The summary of the crystallographic data and refinement parameters is given in Table 1. The selective bond lengths and angles are given in Tables S1–S4 (ESI†). The contribution of the solvent molecules has been incorporated in both the empirical formula and formula weight in Table 1. The formula was designated from the electrons resulted after the SQUEEZE routine and the experimental results (see CIF).
Table 1 Crystallographic data for 1–4
Compound |
1
|
2
|
3
|
4
|
R
1 = ∑||Fo| − |Fc||/∑|Fo|.
wR2 = [∑[w(Fo2 − Fc2)2]/∑w(Fo2)2]1/2.
|
Formula |
C33H48Mn2N8O16 |
C35.5H45.5Co2N6.5O11.5 |
C111H153Mn4N23O37 |
C26H43InN3O23 |
fw |
922.65 |
865.145 |
2621.29 |
880.44 |
Temp (K) |
296(2) |
293(2) |
296(2) |
296(2) |
Wavelength (Å) |
0.71073 |
0.71073 |
0.71073 |
0.71073 |
Cryst syst |
Tetragonal, P 21c |
Monoclinic, P21/n |
Hexagonal, R3c |
Tetragonal, P41212 |
a (Å) |
19.1999(10) |
10.213(2) |
38.9881(10) |
18.0570(3) |
b (Å) |
19.1999(10) |
17.607(4) |
38.9881(10) |
18.0570(3) |
c (Å) |
25.815(3) |
28.123(6) |
22.2962(11) |
13.4495(5) |
V (Å3) |
9516.3(13) |
5000.3(17) |
29 351.2(18) |
4385.28(19) |
Z
|
8 |
4 |
16 |
4 |
F(000) |
2791 |
1556 |
7164 |
900 |
θ range (°) |
1.59–28.29 |
3.07–27.48 |
1.04–28.30 |
1.59–28.29 |
Reflns collected/unique |
69 096/11 809 |
45 488/11 374 |
71 268/16 106 |
32 419/5452 |
R
int
|
0.1481 |
0.1065 |
0.0698 |
0.0467 |
Data/restraints/params |
11 809/9/359 |
11 374/8/408 |
16 106/2/391 |
5452/0/148 |
GOF on F2 |
1.047 |
1.041 |
1.010 |
1.026 |
R
1
, wR2b [I > 2σ(I)] |
0.0896, 0.2219 |
0.0810, 0.2022 |
0.0445, 0.0680 |
0.0276, 0.0520 |
R
1
, wR2b (all data) |
0.1784, 0.2723 |
0.1462, 0.2298 |
0.0967, 0.0730 |
0.0344, 0.0530 |
Synthesis of [Mn2(TADIP)(DMF)3]·2DMF·3H2O (1).
A mixture of MnCl2·4H2O (6 mg, 0.03 mmol), H4TADIP (5 mg, 0.013 mmol) and 70 μL of AcOH in DMF (4 mL) was added to a 20 mL vial, then the solution was heated at 110 °C for 48 h to give colourless block-shaped crystals. Anal. Calcd for C33H48Mn2N8O16: C, 42.96; H, 5.24; N, 12.14%; Found: C, 41.66; H, 5.01; N, 11.36%. IR spectrum (KBr, cm−1): 3418 (br), 3088 (w), 2928 (w), 1660 (s), 1615 (s), 1551 (m), 1441 (m), 1390 (s), 1246 (m), 1103 (m), 1061 (m), 908 (m).
Synthesis of [Co2(TADIP)(DEF)3]·0.5DEF (2).
A mixture of Co(NO3)2·6H2O (10 mg, 0.034 mmol), H4TADIP (5 mg, 0.013 mmol) and AcOH (50 μL) in DEF (1 mL) was added to a 20 mL vial, then the solution was heated at 110 °C for 48 h to give colourless block-shaped crystals. Anal. Calcd for C35.5H45.5Co2N6.5O11.5: C, 49.28; H, 5.30; N, 10.52%. Found: C, 48.60; H, 5.759; N, 10.33%. IR spectrum (KBr, cm−1): 3405 (br), 3094 (w), 2977 (m), 2938 (w), 2884 (w), 1631 (s), 1592 (m), 1452 (s), 1382 (s), 1304 (w), 1265 (m), 1211 (m), 1118 (w), 1109 (w), 1051(w), 1028 (w), 998 (w).
Synthesis of [Mn2(BCBAIP)(DMF)3]·7.5DMF (3).
A mixture of Mn(NO3)2 aqueous (48 μL, 0.5 mmol) and H4BCBAIP (22.5 mg, 0.05 mmol) was dissolved in DMF (5 mL) and HNO3 (10 μL, 2.7 M in DMF), after stirring for 15 min, the mixture was sealed in a vial and kept at 95 °C for 72 h. After cooling to room temperature, the light brown needle-like crystals were collected by filtration, washed with DMF and air-dried. Anal. Calcd for C111H153Mn4N23O37: C, 50.86; H, 5.88; N, 12.29%; found: C, 48.28; H, 5.75; N, 9.63%. IR (KBr, cm−1): 3406 (br), 3063 (w), 2925 (w), 1663 (s), 1603 (s), 1551 (s), 1388 (s), 1242 (m), 1176 (w), 1151 (w), 1104 (m), 1064 (m), 1015 (m), 993 (w).
Synthesis of (Me2NH2)[In(DBIP)]·3DMF·7H2O (4).
In(NO3)·xH2O (20 mg) and H4DBIP (10 mg, 0.03 mmol) were dissolved in a 20 mL vial with DMF (1 mL) and HNO3 (0.5 mL, 2.7 M in DMF), the mixture was then stirred for 30 min and sealed. After being kept at 85 °C for 12 h, the reaction mixture was cooled to room temperature, the light brown block crystals were collected, washed with DMF, and air dried. Anal. Calcd for C26H43InN3O23: C, 35.47; H, 4.92; N, 4.77%; found: C, 34.67; H, 5.617; N, 4.93%. IR (KBr, cm−1) 3445 (br), 2965 (w), 2931 (w), 2886 (w), 2792 (w), 2468 (br), 1654 (s), 1625 (m), 1569 (s), 1469 (s), 1380 (s), 1257 (s), 1133 (s), 1106 (s), 1033 (s).
The dye-adsorption experiments
Several batches of compound 4 (25 mg) were added to the ethanol solution (2 mL) containing 0.05 mg of different dyes including Methylene Blue (MB), Coumarin 6 (C6), Coumarin 343 (C343), Rhodamine B (RB), Methyl Orange (MO), respectively. The liquid state UV-vis adsorption spectra were taken every at fixed intervals over a period of 6 h. The products obtained were named MB@4, C6@4, C343@4, RB@4 and MO@4.
Results and discussion
Crystal structures
Structural description of compound 1.
Compound 1 crystallizes in the tetragonal space group P
21c. The asymmetric unit consists of two crystallographically independent Mn(II) ions (Mn1, Mn2), one TADIP4− anion and three terminal DMF molecules coordinated to the Mn2 ion. The Mn1 ion is six-coordinated with six oxygen atoms (O4–O6, O8–O10) from four TADIP4− anions to form a distorted octahedral geometry. The Mn2 ion is six-coordinated with three oxygen atoms (O3, O7, O9) from three TADIP4− anions and another three (O1, O2, O11) from the DMF molecules to form an octahedral geometry. The average Mn–O bond length ranges from 2.067(5) to 2.305(5) Å. In compound 1, the carboxylate groups of TADIP4− display three kinds of coordination modes: the bridging bismonodentate mode (O3–C11–O5, O4–C18–O7), the chelating bidentate mode (O8–C5–O10), and the bridging-chelating mode (O6–C1–O9), where O9 acts as a μ2 atom. In the crystal structure of compound 1, Mn1 and Mn2 act as a binuclear unit with a Mn⋯Mn distance of 3.556 Å. The binuclear unit is then linked by four organic TADIP4− anions to form a 4-connected 3D framework (Fig. 1). When viewed along the c axis, it can be seen that there exist two different kinds of one-dimensional channels, which can be further parted into four due to their left & right orientation. The ellipsoid-type channel is blocked in the middle by the coordinated DMF molecules, while the square one has a diameter of about 5 Å (considering the van der Waals radii) (Fig. 1c). Topological analysis shows that 1 has a pts topology with a point symbol of (42·84) when considering the Mn2 units and TADIP4− as 4-connected nodes (Fig. 1e,f). The solvent accessible volume is 43.2% of the unit cell volume, calculated from the crystal structure using the PLATON program.
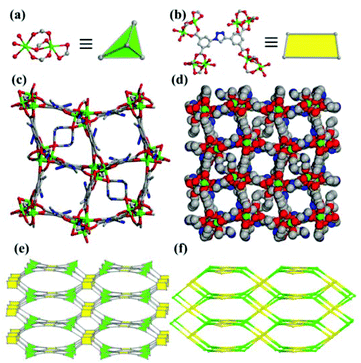 |
| Fig. 1 (a) The coordination environment of binuclear Mn(II) cluster viewed as a 4-connected I-SBU; (b) the ligand H4TADIP coordinated to four binuclear Mn(II) I-SBUs and viewed as a 4-connected O-SBU; (c) the 3D framework of 1; (d) space-filling model of channels along the [001] direction; (e) polyhedral view of the pts topology; (f) the pts topology. | |
Structural description of compound 2.
Compound 2 crystallizes in the monoclinic space group P21/n. The asymmetric units consist of two crystallographically independent Co(II) ions (Co1, Co2), one TADIP4− anion and three terminal DEF molecules coordinated to the Co2 ion. The Co1 ion is 4-coordinated with four oxygen atoms (O1, O3, O5, O11) from four TADIP4− anions to form a tetrahedral geometry, while the Co2 ion is 6-coordinated with three oxygen atoms (O4, O6, O10) from three TADIP4− anions and another three oxygen atoms (O7–O9) from the DEF molecules to form an octahedron. The average Co–O bond length ranges from 1.959(4) to 2.105(5) Å. In compound 2, the carboxylate groups of ligand TADIP4− display two kinds of coordination modes: the bridging bismonodentate mode (O4–C1–O3, O5–C14–O6, O10–C15–O11), and the monodentate mode (O1–C10–O2). In the crystal structure of compound 2, the Co1 and Co2 ions act as a binuclear unit with a Co⋯Co distance of 3.648 Å. The binuclear unit is then linked by four organic TADIP4− anions to form a 4-connected 3D framework. There exist three dimensional cross-typed channels in the framework, one is about 5 × 6 Å along the b axis, while the other is blocked by the coordinated DEF molecules and has a pore of about 3 × 6 Å along the a axis (Fig. 2c,d). The topological analysis reveals that compound 2 has a zeolite-type abw topology with a point symbol of (42·63·8) when considering the Co2 unit and the TADIP4− ligand as 4-connected building blocks (Fig. 2e,f). The solvent accessible volume is 37.6% of the unit cell volume, calculated from the crystal structure using the PLATON program.
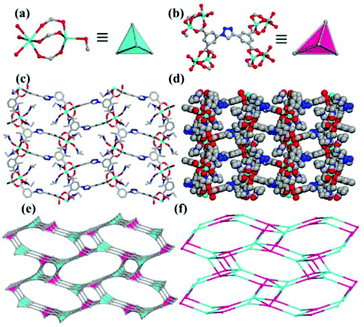 |
| Fig. 2 (a) The coordination environment of binuclear Co(II) cluster viewed as a 4-connected I-SBU; (b) the ligand H4TADIP coordinated to four binuclear Co(II) I-SBUs and viewed as a 4-connected O-SBU; (c) the 3D framework of 2; (d) space-filling model of channels along the [100] direction; (e) polyhedral view of the abw topology; (f) the abw topology. | |
Structural description of compound 3.
Compound 3 crystallizes in the hexagonal R3c space group. The asymmetric unit contains two crystallographically independent Mn(II) ions (Mn1, Mn2), half of an organic BCBAIP4− anion, and three terminal DMF molecules coordinated to the binuclear Mn cluster. The Mn1 ion is six-coordinated with six oxygen atoms from three carboxylate (O4, O6, O8) and three DMF molecules (O9, O10, O11) to form an octahedral geometry, while the Mn2 ion is six-coordinated with six oxygen atoms from four carboxylates (O1–O5, O7) to form a distorted octahedral geometry. The average Mn–O bond length ranges from 2.096(2) to 2.319(2) Å. In this compound, the organic ligand exhibits three kinds of coordination mode: the bridging bismonodentate mode (O5–C24–O6, O7–C1–O8), the chelating bidentate mode (O1–C22–O2), and the bridging-chelating mode (O3–C23–O4), where O4 acts as a μ2 atom. In the compound, the Mn1 and Mn2 ion act as a Mn2 binuclear unit with a Mn⋯Mn distance of 3.499 Å. The binuclear unit is then linked by four organic BCBAIP4− anions to form a 4-connected 3D framework. When viewed along the c axis, one kind of one dimensional channel can be found. The pore dimension of the channel is approximately 11 Å. Fig. 3c illustrates the compound along the c axis with the hexagonal windows. As shown in Fig. S5,† the hexagonal window is constructed by three O-SBUs (BCBAIP4−) and three I-SBUs (Mn2). Further connection of the hexagonal windows can form a SOD cage with twelve I-SBUs and twelve O-SBUs. This cage has a maximum space of about 15 Å, with a dimension of about 11 Å along the windows. When considering the Mn2 cluster as a 4-connected inorganic building unit and BCBAIP4− as a 4-connected organic linker, a topological analysis reveals that this compound has a zeolitic type sod topology (Fig. 3e,f). Such a topology has been realized in our previously reported work using the same ligand and In3+ ions.16 The void volume of compound 3 with the removal of guest solvent molecules is about 54.4% by using PLATON/SQUEEZE. However, although the framework of compound 3 exhibits porous features, the investigation of the N2 adsorption–desorption properties failed.
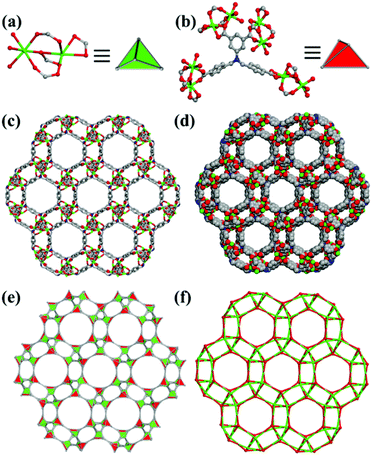 |
| Fig. 3 (a) The coordination environment of binuclear Mn(II) cluster viewed as a 4-connected I-SBU; (b) the ligand H4BCBAIP coordinated to four binuclear Mn(II) I-SUBs and viewed as a 4-connected O-SBU; (c) the 3D framework of 3; (d) space-filling model of channels along the [001] direction; (e) polyhedral view of the sod topology; (f) the sod topology. | |
Structural description of compound 4.
Compound 4 crystallizes in the tetragonal space group P41212. The asymmetric unit consists of one independent In3+ ion, half of a DBIP4− anion, and one Me2NH2+ cation, which was decomposed from the solvent DMF molecules to balance the negative charged framework. The In3+ ion is located on a crystallographically-imposed 2-fold axis. Each In3+ ion is four coordinated to four carboxylate groups from four DBIP4− ligands, and serves as a tetrahedral 4-connected inorganic building unit. Each carboxylate group in an organic ligand adopts a bidentate chelating mode, and coordinates with one indium ion to form a 4-connected organic linker. Further linkage of the 4-connected {In(O2C)4} inorganic building unit with 4-connected organic linker forms a 3D framework (Fig. 4c,d). The structure of compound 4 features two types of one-dimensional channels along the c axis. The large one is about 14 Å, and the small one is 11 × 7 Å. Topological analysis of this network gives rise to a 4-connected net of unc topology with a point symbol of (66) (Fig. 4e,f). The void volume of 4 calculated using PLATON/SQUEEZE is 68.8% of the unit cell volume upon removal of the Me2NH2+ cations and guest solvent molecules.
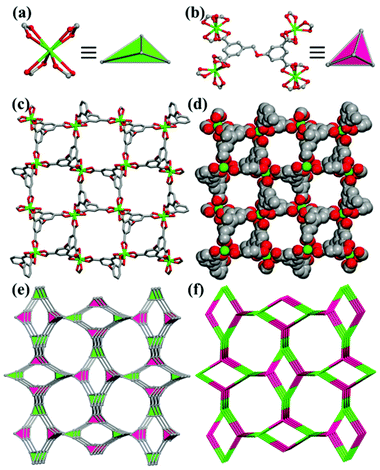 |
| Fig. 4 (a) The coordination environment of In(III) unit viewed as a 4-connected I-SBU; (b) the ligand H4DBIP coordinated to four binuclear In(III) I-SBUs and viewed as a 4-connected O-SBU; (c) the 3D framework of 4; (d) space-filling model of channels along the [001] direction; (e) polyhedral view of the unc topology; (f) the unc topology. | |
Summary of the coordination modes for these ligands and their usage as 4-connected linkers
A summary of the diverse coordination modes of ligands H4TADIP and H4BCBAIP is listed in Fig. 5. The coordination modes of H4DBIP have been depicted in previous work reported by Cao and coworkers.11k It can be seen that all the three ligands chosen here can rotate with different functional groups or bonds to adopt diverse geometries. For compound 1, the ligand H4TADIP can be regarded as a rectangular geometry, while in compounds 2–4, these ligands can be regarded as tetrahedral building blocks. Combined with external factors such as temperature, pH, solvent, and metal salts, these ligands could adopt a tetrahedral or rectangular geometry to fit the need of a better coordination. Thus, the usage of such semi-rigid ligands to coordinate with the metal ions can lead to the formation of 4-connected MOFs with diverse topologies.
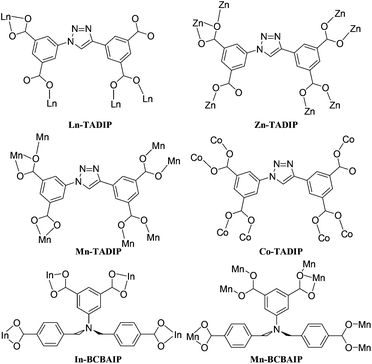 |
| Fig. 5 Summary of diverse coordination modes for H4TADIP and H4BCBAIP. | |
Magnetic properties of compound 1
For compound 1, the Mn1 and Mn2 ions act as a binuclear unit with a Mn⋯Mn distance of 3.556 Å. The temperature-dependent magnetic susceptibility measurement of compound 1 has been performed on the powder sample in the ranges of 2–300 K under a field of 1 kOe. As shown in Fig. 6, the observed value of χMT per Mn unit is 4.58 cm3 K mol−1 at room temperature, which is slightly higher than the value expected for a free Mn(II) ion (4.375 cm3 K mol−1). Upon cooling, the χMT value decreases gradually, until a sharp decrease is observed below 50 K to a value of 0.37 cm3 K mol−1 at 2 K, this feature is possibly the result of intramolecular antiferromagnetic interactions dominating the magnetic properties in compound 1. The plot of χM−1vs. T over the temperature range 25–300 K obeys the Curie–Weiss law [χ = C/(T − θ)] with C = 4.88 cm3 K mol−1 and θ = −18.40 K (Fig. S6†).
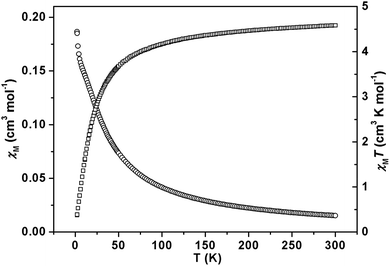 |
| Fig. 6 Temperature dependence of χM (○) and χMT (□) vs. T plots for compound 1 under an applied field of 1 kOe from 2–300 K. | |
The luminescent properties of compound 4
The UV-vis spectra of the free ligand H4DBIP and compound 4 in the solid state are shown in Fig. S9.† The ligand shows the absorption from 200 to 350 nm with the maximum at about 316 nm. The maximum absorption of compound 4 is slightly red-shifted (about 8 nm) compared to the ligand and has an absorption ranging from 200 to 300 nm. Their luminescent spectra are also investigated. When excited at 316 nm, the solid state luminescent spectrum of H4DBIP exhibits a maximum emission at about 380 nm. The emission spectrum of 4 was obtained with an excitation wavelength of 324 nm, and shows a maximum emission at about 460 nm. Compound 4 shows a relatively superior red-shift compared to H4DBIP which might be ascribed to the ligand-to-metal charge transfer (LMCT) (Fig. 7).21
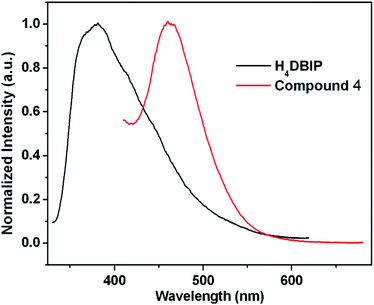 |
| Fig. 7 The luminescent spectra of compound 4 and H4DBIP. | |
The selective dye-adsorption kinetics
To evaluate the porosity of compound 4, a nitrogen (at 77 K) adsorption experiment was performed. Unfortunately, no significant nitrogen uptake was observed. In order to demonstrate the pore accessibility and considering the existence of Me2NH2+ cation in the framework, dye-adsorption experiments were performed using compound 4 with differently charged dyes (cationic MB, RB; neutral C343, C6; anionic MO). When immersed into the ethanol solutions of Methylene Blue (MB), Coumarin 6 (C6), Coumarin 343 (C343), Rhodamine B (RB), and Methyl Orange (MO) for 6 h at room temperature, compound 4 presents different kinetic adsorption behavior toward these dyes as monitored using UV-vis absorption spectroscopy (Fig. S7†). Compound 4 exhibits an exchange rate of MB > C343 > RB > C6 > MO. The diverse exchange behaviors of compound 4 towards these dyes may be ascribed to the anionic nature of the framework and the resulting exchangeable moderate pore size. The Me2NH2+ cation in the channels may be exchanged rapidly by the cationic MB molecules, while RB is resisted due to its large molecule dimensions, and the neutral C343 and C6 are not the favored dyes for the exchange. The negatively charged MO is hard to exchange. To further demonstrate the selectivity of compound 4 for the cationic dyes, especially MB, and considering the easily distinguishable UV-vis spectra, the dyes of MB, MO and RB were mixed together to observe the unique selection behaviors of compound 4 to the cationic dyes. As shown in Fig. 8, compound 4 shows an obvious selection ability for the cationic MB molecules and absorbs the most amount of MB, about 85.1% estimated from the UV-vis absorption spectra.
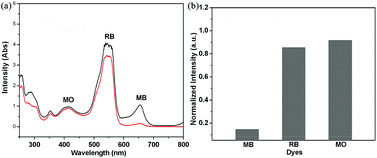 |
| Fig. 8 (a) The UV-vis absorption spectra for the mixture of MB, RB and MO in ethanol before (black line) and after (red line) the addition of compound 4. (b) Peak-height ratios of MB, RB and MO after the addition of compound 4 to the initial intensity. | |
Conclusions
Four novel MOFs were synthesized by the coordination of metal ions (Mn, Co, In) with rigid and semi-rigid tetracarboxylate acid ligands such as H4TADIP, H4BCBAIP, and H4DBIP under solvothermal conditions. Due to the fact that these ligands can exhibit diverse geometries with free rotation through characteristic bonds or functional groups, these ligands can be regarded as 4-connected linkers with quadrangle or tetrahedral building blocks. All of these synthesized MOFs are 4-connected frameworks composed of the above mentioned 4-connected inorganic secondary building units (I-SBUs) and organic secondary building units (O-SBUs). Topological analyses of these compounds reveal the formation of four-connected pts and unc topologies for compounds 1 and 4, and zeolitic abw and sod topologies for compounds 2 and 3. Compound 1 shows an antiferromagnetic behavior. Compound 4 was used to selectively exchange the cationic dyes due to the anionic nature of the frameworks. The anionic nature of compound 4 can be further used in the selective exchange of the cationic MB dye from the mixture of MB, RB and MO in ethanol solution. This work may help to further develop 4-connected metal–organic frameworks with diverse topologies through a 4 + 4 synthetic strategy, and further extension of this work with other metal ions is in progress in our laboratory.
Acknowledgements
We thank the National Natural Science Foundation of China (grants: 91122029 and 21320102001) and the State Basic Research Project of China (grants: 2011CB808703 and 2014CB931802) for support of this work. Z.L. thanks the open project in Key Lab of Functional Inorganic Material Chemistry (Heilongjiang University), Ministry of Education, P. R. China.
Notes and references
-
(a) J. R. Li, R. J. Kuppler and H. C. Zhou, Chem. Soc. Rev., 2009, 38, 1477–1504 RSC;
(b) L. J. Murray, M. Dincǎ and J. R. Long, Chem. Soc. Rev., 2009, 38, 1294–1314 RSC;
(c) J.-R. Li, J. Sculley and H.-C. Zhou, Chem. Rev., 2012, 112, 869–932 CrossRef CAS PubMed;
(d) M. P. Suh, H. J. Park, T. K. Prasad and D.-W. Lim, Chem. Rev., 2012, 112, 782–835 CrossRef CAS PubMed;
(e) K. Sumida, D. L. Rogow, J. A. Mason, T. M. McDonald, E. D. Bloch, Z. R. Herm, T.-H. Bae and J. R. Long, Chem. Rev., 2012, 112, 724–781 CrossRef CAS PubMed.
-
(a) M. Heitbaum, F. Glorius and I. Escher, Angew. Chem., Int. Ed., 2006, 45, 4732–4762 CrossRef CAS PubMed;
(b) D. Farrusseng, S. Aguado and C. Pinel, Angew. Chem., Int. Ed., 2009, 48, 7502–7513 CrossRef CAS PubMed;
(c) J. Lee, O. K. Farha, J. Roberts, K. A. Scheidt, S. T. Nguyen and J. T. Hupp, Chem. Soc. Rev., 2009, 38, 1450–1459 RSC;
(d) A. Corma, H. García and F. X. Llabres i Xaména, Chem. Rev., 2010, 110, 4606–4655 CrossRef CAS PubMed;
(e) Y. Liu, W. Xuan and Y. Cui, Adv. Mater., 2010, 22, 4112–4135 CrossRef CAS PubMed;
(f) M. Yoon, R. Srirambalaji and K. Kim, Chem. Rev., 2012, 112, 1196–1231 CrossRef CAS PubMed.
-
(a) D. Maspoch, D. Ruiz-Molina and J. Veciana, J. Mater. Chem., 2004, 14, 2713–2723 RSC;
(b) M. Kurmoo, Chem. Soc. Rev., 2009, 38, 1353–1379 RSC;
(c) P. Dechambenoit and J. R. Long, Chem. Soc. Rev., 2011, 40, 3249–3265 RSC;
(d) D. F. Weng, Z. M. Wang and S. Gao, Chem. Soc. Rev., 2011, 40, 3157–3181 RSC.
-
(a) M. D. Allendorf, C. A. Bauer, R. K. Bhakta and R. J. T. Houk, Chem. Soc. Rev., 2009, 38, 1330–1352 RSC;
(b) J. Rocha, L. D. Carlos, F. A. Almeida Paz and D. Ananias, Chem. Soc. Rev., 2011, 40, 926–940 RSC;
(c) Y. Cui, Y. Yue, G. Qian and B. Chen, Chem. Rev., 2012, 112, 1126–1162 CrossRef CAS PubMed;
(d) L. E. Kreno, K. Leong, O. K. Farha, M. Allendorf, R. P. Van Duyne and J. T. Hupp, Chem. Rev., 2012, 112, 1105–1125 CrossRef CAS PubMed.
-
(a) B. Chen, S. Xiang and G. Qian, Acc. Chem. Res., 2010, 43, 1115–1124 CrossRef CAS PubMed;
(b) A. Bétard and R. A. Fischer, Chem. Rev., 2012, 112, 1055–1083 CrossRef PubMed;
(c) P. Horcajada, R. Gref, T. Baati, P. K. Allan, G. Maurin, P. Couvreur, G. Férey, R. E. Morris and C. Serre, Chem. Rev., 2012, 112, 1232–1268 CrossRef CAS PubMed.
-
(a) J. Kim, B. Chen, T. M. Reineke, H. Li, M. Eddaoudi, D. B. Moler, M. O'Keeffe and O. M. Yaghi, J. Am. Chem. Soc., 2001, 123, 8239–8247 CrossRef CAS PubMed;
(b) M. Eddaoudi, D. B. Moler, H. L. Li, B. L. Chen, T. M. Reineke, M. O'Keeffe and O. M. Yaghi, Acc. Chem. Res., 2001, 34, 319–330 CrossRef CAS PubMed;
(c) D. J. L. Tranchemontagne, Z. Ni, M. O'Keeffe and O. M. Yaghi, Angew. Chem., Int. Ed., 2008, 47, 5136–5147 CrossRef CAS PubMed.
- D. J. Tranchemontagne, J. L. Mendoza-Cortés, M. O'Keeffe and O. M. Yaghi, Chem. Soc. Rev., 2009, 38, 1257–1283 RSC.
-
(a) L. Ma, A. Jin, Z. Xie and W. Lin, Angew. Chem., Int. Ed., 2009, 48, 9905–9908 CrossRef CAS PubMed;
(b) B. Zheng, Z. Liang, G. Li, Q. Huo and Y. Liu, Cryst. Growth Des., 2010, 10, 3405–3409 CrossRef CAS;
(c) L. Wen, P. Cheng and W. Lin, Chem. Sci., 2012, 3, 2288–2292 RSC;
(d) Y. Yan, S. Yang, A. J. Blake and M. Schröder, Acc. Chem. Res., 2014, 47, 296–307 CrossRef CAS PubMed.
- B. Chen, N. W. Ockwig, A. R. Millward, D. S. Contreras and O. M. Yaghi, Angew. Chem., Int. Ed., 2005, 44, 4745–4749 CrossRef CAS PubMed.
-
(a) X. Lin, J. Jia, X. Zhao, K. M. Thomas, A. J. Blake, G. S. Walker, N. R. Champness, P. Hubberstey and M. Schröder, Angew. Chem., Int. Ed., 2006, 45, 7358–7364 CrossRef CAS PubMed;
(b) S. Yang, X. Lin, A. J. Blake, K. M. Thomas, P. Hubberstey, N. R. Champness and M. Schröder, Chem. Commun., 2008, 6108–6110 RSC;
(c) X. Lin, I. Telepeni, A. J. Blake, A. Dailly, C. M. Brown, J. M. Simmons, M. Zoppi, G. S. Walker, K. M. Thomas, T. J. Mays, P. Hubberstey, N. R. Champness and M. Schröder, J. Am. Chem. Soc., 2009, 131, 2159–2171 CrossRef CAS PubMed;
(d) S. Yang, X. Lin, A. Dailly, A. J. Blake, P. Hubberstey, N. R. Champness and M. Schröder, Chem. – Eur. J., 2009, 15, 4829–4835 CrossRef CAS PubMed;
(e) S. Yang, X. Lin, A. J. Blake, G. S. Walker, P. Hubberstey, N. R. Champness and M. Schröder, Nat. Chem., 2009, 1, 487–493 CrossRef CAS PubMed.
-
(a) Y. Liu, J. F. Eubank, A. J. Cairns, J. Eckert, V. C. Kravtsov, R. Luebke and M. Eddaoudi, Angew. Chem., Int. Ed., 2007, 46, 3278–3283 CrossRef CAS PubMed;
(b) A. J. Cairns, J. A. Perman, L. Wojtas, V. C. Kravtsov, M. H. Alkordi, M. Eddaoudi and M. J. Zaworotko, J. Am. Chem. Soc., 2008, 130, 1560–1561 CrossRef CAS PubMed;
(c) Y.-G. Lee, H. R. Moon, Y. E. Cheon and M. P. Suh, Angew. Chem., Int. Ed., 2008, 47, 7741–7745 CrossRef CAS PubMed;
(d) L. Ma, J. Y. Lee, J. Li and W. Lin, Inorg. Chem., 2008, 47, 3955–3957 CrossRef CAS PubMed;
(e) X.-S. Wang, S. Ma, K. Rauch, J. M. Simmons, D. Yuan, X. Wang, T. Yildirim, W. C. Cole, J. J. López, A. D. Meijere and H.-C. Zhou, Chem. Mater., 2008, 20, 3145–3152 CrossRef CAS;
(f) M. Xue, G. Zhu, Y. Li, X. Zhao, Z. Jin, E. Kang and S. Qiu, Cryst. Growth Des., 2008, 8, 2478–2483 CrossRef CAS;
(g) Y. Hu, S. Xiang, W. Zhang, Z. Zhang, L. Wang, J. Bai and B. Chen, Chem. Commun., 2009, 7551–7553 RSC;
(h) T.-F. Liu, J. Lü, Z. Guo, D. M. Proserpio and R. Cao, Cryst. Growth Des., 2010, 10, 1489–1491 CrossRef CAS;
(i) T.-F. Liu, J. Lü, C. Tian, M. Cao, Z. Lin and R. Cao, Inorg. Chem., 2011, 50, 2264–2271 CrossRef CAS PubMed;
(j) Z.-J. Lin, T.-F. Liu, Y.-B. Huang, J. Lü and R. Cao, Chem. – Eur. J., 2012, 18, 7896–7902 CrossRef CAS PubMed;
(k) Z.-J. Lin, L.-W. Han, D.-S. Wu, Y.-B. Huang and R. Cao, Cryst. Growth Des., 2012, 13, 255–263 CrossRef;
(l) B. Zheng, H. Liu, Z. Wang, X. Yu, P. Yi and J. Bai, CrystEngComm, 2013, 15, 3517–3520 RSC;
(m) B. Zheng, R. Yun, J. Bai, Z. Lu, L. Du and Y. Li, Inorg. Chem., 2013, 52, 2823–2829 CrossRef CAS PubMed;
(n) X. Rao, J. Cai, J. Yu, Y. He, C. Wu, W. Zhou, T. Yildirim, B. Chen and G. Qian, Chem. Commun., 2013, 49, 6719–6721 RSC;
(o) J. Cai, X. Rao, Y. He, J. Yu, C. Wu, W. Zhou, T. Yildirim, B. Chen and G. Qian, Chem. Commun., 2014, 50, 1552–1554 RSC.
-
(a) Q. Fang, G. Zhu, M. Xue, J. Sun, Y. Wei, S. Qiu and R. Xu, Angew. Chem., Int. Ed., 2005, 44, 3845–3848 CrossRef CAS PubMed;
(b) X.-C. Huang, Y.-Y. Lin, J.-P. Zhang and X.-M. Chen, Angew. Chem., Int. Ed., 2006, 45, 1557–1559 CrossRef CAS PubMed;
(c) Y. Liu, V. C. Kravtsov, R. Larsen and M. Eddaoudi, Chem. Commun., 2006, 1488–1490 RSC;
(d) H. Hayashi, A. P. Cote, H. Furukawa, M. O'Keeffe and O. M. Yaghi, Nat. Mater., 2007, 6, 501–506 CrossRef CAS PubMed;
(e) H. Zhang, T. Wu, C. Zhou, S. Chen, P. Feng and X. Bu, Angew. Chem., Int. Ed., 2009, 48, 2542–2545 CrossRef PubMed;
(f) M. H. Alkordi, J. A. Brant, L. Wojtas, V. C. Kravtsov, A. J. Cairns and M. Eddaoudi, J. Am. Chem. Soc., 2009, 131, 17753–17755 CrossRef CAS PubMed.
-
(a) Y.-Q. Tian, Y.-M. Zhao, Z.-X. Chen, G.-N. Zhang, L.-H. Weng and D.-Y. Zhao, Chem. – Eur. J., 2007, 13, 4146–4154 CrossRef CAS PubMed;
(b) R. Banerjee, A. Phan, B. Wang, C. Knobler, H. Furukawa, M. O'Keeffe and O. M. Yaghi, Science, 2008, 319, 939–943 CrossRef CAS PubMed;
(c) T. Wu, J. Zhang, C. Zhou, L. Wang, X. Bu and P. Feng, J. Am. Chem. Soc., 2009, 131, 6111–6113 CrossRef CAS PubMed.
-
(a) S.-T. Zheng, F. Zuo, T. Wu, B. Irfanoglu, C. Chou, R. A. Nieto, P. Feng and X. Bu, Angew. Chem., Int. Ed., 2011, 50, 1849–1852 CrossRef CAS PubMed;
(b) S.-T. Zheng, T. Wu, F. Zuo, C. Chou, P. Feng and X. Bu, J. Am. Chem. Soc., 2012, 134, 1934–1937 CrossRef CAS PubMed.
-
(a) J. Xu, L. Sun, H. Xing, Z. Liang, J. Yu and R. Xu, Inorg. Chem. Commun., 2011, 14, 978–981 CrossRef CAS PubMed;
(b) L. Sun, Y. Li, Z. Liang, J. Yu and R. Xu, Dalton Trans., 2012, 41, 12790–12796 RSC;
(c) L. Sun, H. Xing, J. Xu, Z. Liang, J. Yu and R. Xu, Dalton Trans., 2013, 42, 5508–5513 RSC;
(d) D.-S. Chen, L.-B. Sun, Z.-Q. Liang, K.-Z. Shao, C.-G. Wang, Z.-M. Su and H.-Z. Xing, Cryst. Growth Des., 2013, 13, 4092–4099 CrossRef CAS;
(e) Z. Liang, J. Du, L. Sun, J. Xu, Y. Mu, Y. Li, J. Yu and R. Xu, Inorg. Chem., 2013, 52, 10720–10722 CrossRef CAS PubMed.
- L. Sun, H. Xing, Z. Liang, J. Yu and R. Xu, Chem. Commun., 2013, 49, 11155–11157 RSC.
- X.-J. Wang, P.-Z. Li, L. Liu, Q. Zhang, P. Borah, J. D. Wong, X. X. Chan, G. Rakesh, Y. Li and Y. Zhao, Chem. Commun., 2012, 48, 10286–10288 RSC.
-
Bruker AXS Inc. E. C. P., Madison, WI 53711-5373, USA, 2000.
- G. M. Sheldrick, Acta Crystallogr., Sect. A: Fundam. Crystallogr., 2008, 64, 112–122 CrossRef CAS PubMed.
-
(a) A. Spek, J. Appl. Crystallogr., 2003, 36, 7–13 CrossRef CAS;
(b) A. Spek, Acta Crystallogr., Sect. D: Biol. Crystallogr., 2009, 65, 148–155 CrossRef CAS PubMed.
-
(a) W.-Q. Kan, B. Liu, J. Yang, Y.-Y. Liu and J.-F. Ma, Cryst. Growth Des., 2012, 12, 2288–2298 CrossRef CAS;
(b) J. Guo, J. Yang, Y.-Y. Liu and J.-F. Ma, CrystEngComm, 2012, 14, 6609–6617 RSC;
(c) Z.-J. Lin, Y.-B. Huang, T.-F. Liu, X.-Y. Li and R. Cao, Inorg. Chem., 2013, 52, 3127–3132 CrossRef CAS PubMed.
Footnote |
† Electronic supplementary information (ESI) available: Complete X-ray crystallographic data for 1–4 in CIF format. PXRD patterns, TGA and IR spectra for 1–4. UV-vis, luminescence and dyes adsorption for 4. Selected bond lengths and angles for compound 1–4. CCDC 993507–993510. For ESI and crystallographic data in CIF or other electronic format see DOI: 10.1039/c4qi00047a |
|
This journal is © the Partner Organisations 2014 |
Click here to see how this site uses Cookies. View our privacy policy here.