DOI:
10.1039/C4QO00185K
(Research Article)
Org. Chem. Front., 2014,
1, 1083-1090
A versatile fluorescent dye based on naphthalimide: highly selective detection of Hg2+ in aqueous solution and living cells and its aggregation-induced emission behaviour†
Received
1st July 2014
, Accepted 2nd September 2014
First published on 3rd September 2014
Abstract
A simple and versatile fluorescent dye NPS based on 1,8-naphthalimide was successfully designed and prepared. It was found that NPS exhibited excellent selectivity for Hg2+ over many other metal ions including Zn2+, Mn2+, Ba2+, Ni2+, Cu2+, Co2+, Pb2+, Mg2+, Cd2+, Fe2+, Al3+, Ag+, Na+, and Li+. In particular, NPS displayed a significant fluorescence “turn-on” to Hg2+ in aqueous solution and living cells, which could be potentially used for quantification of Hg2+. Moreover, two model compounds were rationally designed and synthesized, from which further insight into the sensing mechanism and binding mode of NPS with Hg2+ was obtained. Surprisingly, NPS exhibited interesting twisted intramolecular charge transfer (TICT) and aggregation-induced emission (AIE) behaviours in aqueous solution.
Introduction
The Hg(II) ion, one of the most prevalent toxic metal ions in the environment, has attracted considerable attention during the past few years within modern chemistry and biology.1 Recent studies have indicated that Hg2+ can easily pass through the biological membranes and cause some serious human health problems including DNA damage, mitosis impairment, and permanent damage to the central nervous system.2 Therefore, there is a rising demand for the determination of Hg2+ both in the environment and biological systems. Currently, many approaches, such as atomic absorption spectroscopy, inductively coupled plasma mass spectrometry, and electrochemistry, have been established for the detection of Hg2+.3 However, most of these methods are restricted to the complex instruments and procedures, high cost, or low sensitivity. Because of the operation simplicity, low cost, high sensitivity, real-time detection, and readily available in vivo or in vitro cell imaging, the fluorescence technique has evolved to be one of the most efficient approaches for the detection of Hg2+.4
Up to now, many fluorescent probes for Hg2+ have been reported in the literature.5,6 However, being one of the heavy metal ions, binding with Hg2+ generally causes fluorescence quenching through an efficient spin–orbit coupling,7 which is not only disadvantageous for a high signal output during detection but also undesirable for analytical purposes. Moreover, many of the reported fluorescent probes for Hg2+ lack good selectivity toward potential competitors such as Ag+, Pb2+, and Cu2+ due to their similar chemical behaviour to Hg2+.8 Furthermore, some of the known Hg2+ fluorescent sensors sometimes suffer from the intricate synthesis procedure and the relatively high costs of starting materials,9 thus hindering their further applications. Therefore, it is still necessary to design and synthesize a new fluorescent “turn-on” probe that can detect Hg2+ with high selectivity and sensitivity both in aqueous solution and living cells through a simple synthetic approach.
In continuation of our interest in the fluorescent probes and fluorescent materials,10 herein, we report a novel fluorescent probe NPS based on naphthalimide (Fig. 1). It was found that NPS displayed excellent selectivity for Hg2+ over a wide range of metal ions including Zn2+, Mn2+, Ba2+, Ni2+, Cu2+, Co2+, Pb2+, Mg2+, Cd2+, Fe2+, Al3+, Ag+, Na+, and Li+ in aqueous solution.
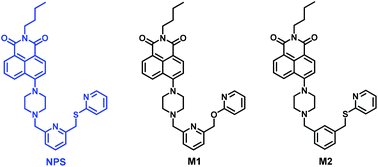 |
| Fig. 1 The structures of fluorescent probe NPS and model compounds M1 and M2. | |
The addition of Hg2+ induced a remarkable 10-fold enhancement in the emission intensity of NPS. Furthermore, through the comparison with two model compounds M1 and M2, the detailed sensing mechanism and binding model of NPS with Hg2+ were proposed. Additionally, it was interesting to find that compound NPS surprisingly showed TICT and AIE behaviours in aqueous solution depending on the polarity of the solvents. Considering the fact that both of the performances of detection of Hg2+ and the AIE behaviour were based on the naphthalimide fluorophore, this study not only provided a highly efficient sensor for Hg2+ detection in aqueous solution and living cells but also enriched the luminous family of AIE molecules.
Results and discussion
Synthesis of NPS, M1, and M2
As shown in Scheme 1, the compounds NPS, M1, and M2 were readily synthesized from N-butyl-4-bromo-1,8-naphthalimide through a three-step reaction in good yields. The structures of all compounds were well characterised by 1H NMR, 13C NMR, and HRMS. Moreover, the structure of the fluorescent probe NPS was unambiguously determined by means of X-ray single-crystal diffraction. The ORTEP diagram of NPS indicated that the molecule was nearly planar and exhibited an open receptor suitable for binding metal ions (Scheme 1).
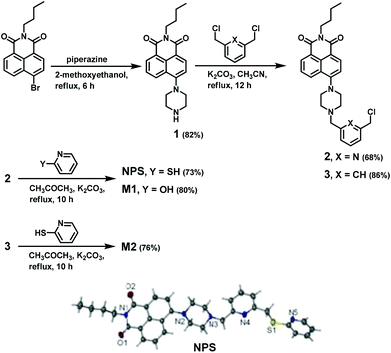 |
| Scheme 1 Synthesis of compounds NPS, M1, and M2; crystal structure of NPS. | |
The fluorescence sensing of Hg2+ by NPS in aqueous solution and living cells
In order to eliminate the disturbance of pH during the detection as well as probe the optimum sensing conditions, the absorption and fluorescence properties of NPS on changing pH were investigated. As shown in Fig. S1,† within the pH range of 10.0–5.1, a slight change in the absorption spectra of NPS was observed. However, due to the protonation of the pyrazine nitrogen that linked to the naphthalimide ring in strong acidic environment, the absorption spectra of NPS blue-shifted from 406 nm to 385 nm with the decrease of the pH from 5.1 to 3.1. Moreover, as shown in Fig. S1c,† almost no fluorescence intensity change of NPS within the pH range of 11.0 to 7.0 was observed, suggesting that NPS was quite stable within the pH range of 7.0–11.0. When the pH value was gradually decreased from 7.0, the emission intensity of NPS gradually increased, which was attributed to the inhibition of the photo-induced electron transfer (PET) process. Therefore, the obtained results demonstrated that NPS could work within the pH range of 7.0 to 11.0, which was suitable for the physiological detection.
The binding selectivity studies of NPS to metal ions in aqueous solution (THF–H2O, 1
:
1, pH 7.4, 10 mM Tris-HCl) were performed by recording the fluorescence changes of the solutions with the addition of each representative metal ion including Zn2+, Mn2+, Ba2+, Hg2+, Ni2+, Cu2+, Co2+, Pb2+, Mg2+, Cd2+, Fe2+, Al3+, Ag+, Na+, and Li+. As shown in Fig. 2a–b, the free NPS showed a weak fluorescence in aqueous solution mainly due to the PET process from the electron-rich receptor to the excited naphthalimide fluorophore. Upon adding Hg2+ to a solution of NPS, a significant enhancement of fluorescence was observed. However, under the identical conditions, almost no change of fluorescence spectra was found with the addition of other ions. The obtained results indicated that NPS was a highly selective fluorescence “turn-on” probe for Hg2+ in aqueous solution. In addition, achieving the high selectivity toward Hg2+ over the other competitive species coexisting is a very important feature to evaluate the performance of the fluorescent probe NPS. Therefore, the competition experiments of NPS were carried out as well. As shown in Fig. 2c, the addition of Hg2+ into the solution of NPS in the presence of another metal ion, the fluorescence emission spectra displayed nearly an identical pattern to that with Hg2+ only. Thus, the competition studies further suggested that NPS was a highly selective fluorescent probe for Hg2+.
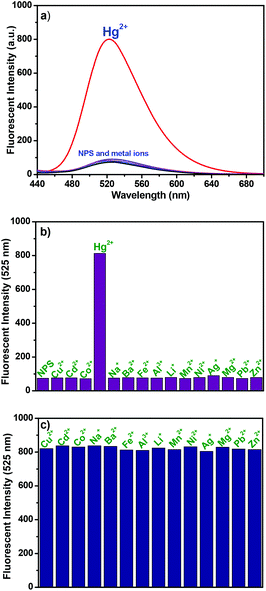 |
| Fig. 2 (a) The fluorescence spectra and (b) fluorescent intensity at 525 nm of NPS (10 μM) in the presence of 8 equivalents of the metal ion such as Zn2+, Mn2+, Ba2+, Hg2+, Ni2+, Cu2+, Co2+, Pb2+, Mg2+, Cd2+, Fe2+, Al3+, Ag+, Na+, and Li+ in aqueous solution (THF–H2O, 1 : 1, v/v, 10 mM Tris-HCl, pH 7.4). (c) The fluorescent intensity at 525 nm of NPS (10 μM) with 8 equivalents of various metal ions, and then added 8 equivalents of Hg2+ in aqueous solution (THF–H2O, 1 : 1, v/v, 10 mM Tris-HCl, pH 7.4) (λex = 405 nm. slits: 5, 5 nm). | |
The responses of NPS to Hg2+ were further investigated in detail through the fluorescent technique as shown in Fig. 3. With the addition of Hg2+ into the solution of NPS, the fluorescence increased gradually by about 10-fold without any significant shift in the emission maximum; however, the absorption spectra displayed a hypsochromic shift (ca. 6 nm) with a slight change in extinction coefficients (Fig. S3†). Notably, the fluorescence intensity of NPS at 525 nm increased almost linearly (R2 = 0.9859) with the increase of Hg2+ concentration (1–30 μM). Moreover, through the concentration-dependent fluorescence changes, the detection limit of NPS for the determination of Hg2+ was determined to be 6.28 × 10−8 M. These results indicated that NPS was sensitive to Hg2+ and could be potentially used to quantitatively detect the Hg2+ concentration in aqueous solution. In order to elucidate the binding stoichiometry of NPS with Hg2+, Job's plot analysis was conducted. As shown in Fig. 4, a maximum at 0.5 M fraction of Hg2+ was observed, which indicated that NPS formed a 1
:
1 binding stoichiometrical complex with Hg2+. Through the fluorescence titration of NPS with Hg2+ (Fig. 3a), the association constant (Ka) between NPS and Hg2+ was established to be 5.4 × 104 M−1 by using nonlinear least-square analysis. Thus, the Kd between NPS and Hg2+ was calculated to be 0.18 × 10−4 M through the equation of Kd = 1/Ka, which might be the reason that the emission of NPS continued to increase until the addition of 7 equivalents Hg2+ although NPS formed a 1
:
1 binding stoichiometrical complex with Hg2+. Moreover, as shown in Fig. S4,† compared to the case of NPS in protonation, the addition of Hg2+ to the solution of NPS induced a lower fluorescence enhancement. The results suggested that Hg2+ can still quench the fluorescence to some extent through spin–orbit effect despite blocking the PET upon Hg2+ binding.
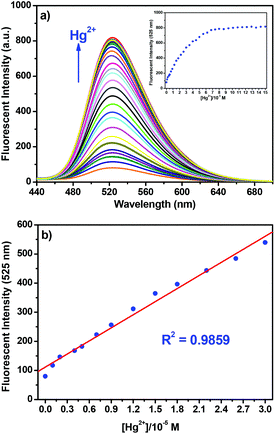 |
| Fig. 3 (a) Fluorescence spectra of NPS (10 μM) upon the addition of Hg2+ (0.1–15 equivalents) in aqueous solution (THF–H2O, 1 : 1, v/v, 10 mM Tris-HCl, pH 7.4). Inset (a) and (b) curve of fluorescence intensity at 525 nm of NPS (10 μM) versus increasing concentrations of Hg2+ (λex = 405 nm, slits: 5, 5 nm). | |
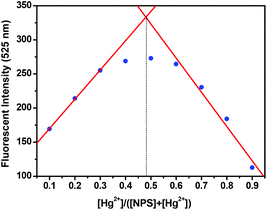 |
| Fig. 4 Job's plot of NPS and Hg2+ ([NPS] + [Hg2+] = 20 μM) in aqueous solution (THF–H2O, 1 : 1, v/v, 10 mM Tris-HCl, pH 7.4) (λex = 405 nm, slits: 5, 5 nm). | |
With the aim of gaining further insight into the fluorescence sensing mechanism of NPS for Hg2+, the fluorescence spectra of free NPS and the two model compounds M1 and M2 were examined. As shown in Fig. 5, the weak fluorescence of these three compounds clearly revealed that the piperazine nitrogen played a significant role in quenching the fluorescence of the naphthalimide fluorophore through the PET process. The weaker fluorescence of NPS compared to M1 indicated that the sulfur atom in the NPS moiety was involved in quenching the fluorescence as well. Moreover, the similar fluorescence spectra of NPS and M2 revealed that the nitrogen atom in 2,6-bis(chloromethyl)pyridine played a negligible role in the fluorescence quenching. In addition to the fluorescence quenching via the PET mechanism, the high degree of molecular flexibility of NPS might also have the fundamental implication.
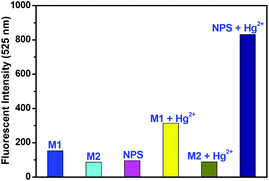 |
| Fig. 5 Fluorescence intensity at 525 nm of NPS, M1, and M2 (all compounds were 10 μM) in the absence and presence of 8 equivalents of Hg2+ in aqueous solution (THF–H2O, 1 : 1, v/v, 10 mM Tris-HCl, pH 7.4) (λex = 405 nm, slits: 5, 5 nm). | |
The fluorescence off–on change of NPS responding to Hg2+ might be attributed to the PET mechanism as follows. In the absence of Hg2+, free NPS displayed a weak fluorescence because of the lone electron pairs of the piperazine nitrogen and sulfur atom being located close to the naphthalimide moiety, which induced an intramolecular photoinduced electron transfer. However, in the presence of Hg2+, the PET process was blocked and the complex was more rigid, thereby a significant enhancement of fluorescence was observed.
With the aim to understand the binding model of NPS with Hg2+, the fluorescence responses of NPS, M1, and M2 to Hg2+ were determined as shown in Fig. 5 and S6.† The lower binding abilities of M1 and M2 with Hg2+ compared to NPS verified that both the sulfur atom and the nitrogen atom in 2,6-bis(chloromethyl)pyridine played an important role in the binding of NPS with Hg2+. Generally, when nitrogen, which is also the donor of a push–pull system, chelates to metal ions, it can induce a hypsochromic shift in absorption or emission maximum.11 Thus, as shown in Fig. S3,† the observation of the hypsochromic shift of the absorption wavelength of NPS with the addition of Hg2+ indicated that the pyrazine nitrogen linking to the naphthalimide ring was involved in Hg2+ chelation. Moreover, as mentioned above, the significant enhancement of fluorescence with the adding of Hg2+ suggested that the other pyrazine nitrogen also participated in the binding thereby inhibited the PET process.
In order to further elucidate the binding mode of NPS with Hg2+, the 1H NMR titration experiments of Hg2+ (0.1–0.3 equivalent) were conducted. As shown in Fig. S7† with the addition of Hg2+, the resonance signals corresponding to the piperazine protons (H3 and H4) displayed an obvious downfield, which suggested that the piperazine nitrogens participated in the binding process. Similarly, the apparent downfield shifts of H1 and H2 indicated that the sulfur atom was involved in the binding process as well. The results further confirmed the aforementioned sensing mechanism. However, due to the poor solubility of the complex (NPS binding with Hg2+) in the mixture solution of CD3CN and DMSO-d6 as well as the spin–orbit effect of Hg2+, the further addition of Hg2+ caused the broadening of the peaks in 1H NMR spectra.
Therefore, based on the above studies including absorption and fluorescence titration spectra, the Job's plots, the comparison with model compounds, the coordination chemistry of Hg2+, and the 1H NMR titration experiments of Hg2+, the sensing mechanisms and binding modes of the probe NPS with Hg2+ were proposed as shown in Fig. 6.
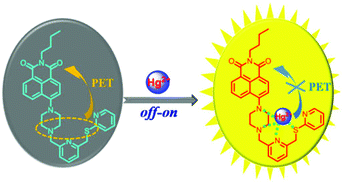 |
| Fig. 6 Proposed binding model of NPS with Hg2+. | |
The fluorescence imaging experiments of NPS in living cells were carried out as shown in Fig. 7 to further develop the potential application of NPS in biological systems. Hela cells were incubated with NPS in DMEM (Dulbecco's modified Eagle's medium, containing 5% DMF) for 30 min, followed by the addition of 5.0 equivalents Hg2+ and then incubated for another 20 min. The cells were washed twice with PBS buffer solution. It was found that non-fluorescence was nearly detected when Hela cells were treated with the NPS probe only. By contrast, when Hela cells were incubated with the NPS probe treated with Hg2+, a bright blue fluorescence was observed (Fig. 7e). The results suggested that NPS can be employed for imaging of Hg2+ in living cells.
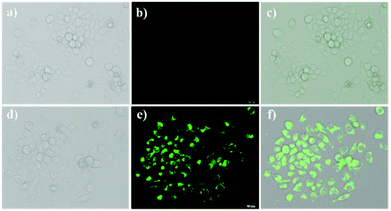 |
| Fig. 7 (a) Bright-field transmission image of Hela cells pre-incubated with probe NPS (10 μM) in DMEM (containing 5% DMF) for 30 min, washed two times. (b) Fluorescence image of (a). (c) Overlay of (a) and (b). (d) Bright-field transmission image of Hela cells pre-incubated with probe NPS (10 μM) in DMEM (containing 5% DMF) for 30 min, washed twice, and then treated with Hg2+ (5.0 equiv.). (e) Fluorescence image of (d). (f) Overlay of (d) and (e). | |
The AIE behaviour of NPS
Generally, aggregation-caused quenching (ACQ) phenomenon is common to most of the aromatic hydrocarbons and their derivatives.12 The ACQ effect was usually caused by the formation of sandwich-shaped excimers and exciplexes aided by the collisional interactions between the aromatic molecules in the excited and ground states. In 2001, Tang et al. discovered a series of silole derivatives that were non-emissive in dilute solutions but highly emissive upon being aggregated, which was defined as “aggregation-induced emission” (AIE).13 Taking advantage of the AIE effect, recently, a variety of AIE fluorogens have been developed with wide applications in organic light-emitting diodes, chemo-sensing, bio-probing, etc.14 Up to now, the AIE fluorogens were mainly limited to tetraphenylsilole (TPS) and tetraphenylethene (TPE). Very recently, Li et al. reported a new organic dye based on 1,8-naphthalimide, which exhibited the significant AIE characteristic and could be utilized as a bio-probe specified to illuminate cell membranes.15 Inspired by these successful examples, further fluorescence studies were carried out to evaluate the AIE behaviour of NPS in this case. As shown in Fig. 8, with the gradual addition of water to the THF solution of NPS, the emission intensity decreased gradually accompanied with a slight bathochromic shift when the fraction of water (fw) was less than 70%. In contrast, at higher fw (from 70% to 100%), the emission intensity of NPS dramatically increased upon increasing water content, which was consistent with a typical AIE feature.
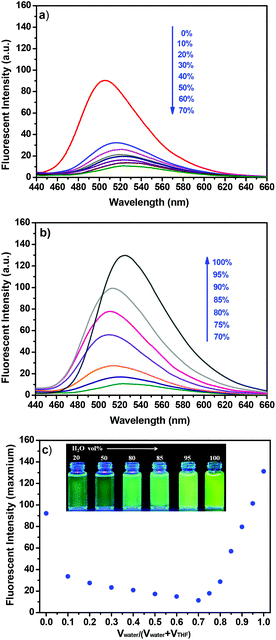 |
| Fig. 8 The fluorescence spectra of NPS in the THF–H2O mixture with different fractions (a, 0–70%, b, 70–100%). (c) Plots of fluorescence areas of NPSversus water fraction in the THF–H2O mixture. Inset, the digital photos of NPS in the THF–H2O mixture under irradiation of a UV lamp at 365 nm (λex = 405 nm, slits: 2.5, 5 nm). | |
In order to elucidate the change of emission of NPS with different H2O fractions in THF, a possible mechanism was proposed as follows. In general, twisted intramolecular charge transfer (TICT) occurs in a luminogen system containing donor (D) and acceptor (A) units with push–pull interactions.14a Usually, in the polar solvent, a bathochromic shift for the TICT molecule is observed because the polar solvent alters its ground and excited states and narrows its energy gap.16 Moreover, when a TICT molecule is dissolved in a polar solvent, the charge-separated conformation resulting from intramolecular twisting is stabilized by the solvent effect. Thus the excited state often decayed non-radiatively and the fluorescence emission is weak.17
As shown in Fig. 8a, the emission intensity of NPS decreased gradually accompanied with a bathochromic shift upon increasing the fraction of water (fw), less than 70%. Furthermore, as shown in Fig. 9, an obvious bathochromic shift (ca. 45 nm) for NPS was observed upon increasing the solvent polarity. The results indicated that the TICT feature dominated the emission intensity of NPS with a relatively low polarity (fw less than 70%) of the solvent. However, as shown in Fig. 8b, when the H2O fraction was more than 70% in THF, NPS began to aggregate in the mixed solvent and the induced emission intensity increased gradually since the aqueous mixture became more polar, which is consistent with the feature of the AIE effect. Thus, NPS was found to be an interesting fluorescent dye that displayed both TICT and AIE activity in aqueous solution, in which the TICT effect played a dominant role in the solution with relatively low polarity but was converted to the AIE effect in the highly polar solution. Based on the mechanisms discussed above, a diagram for the TICT and AIE processes of NPS was proposed as shown in Fig. 10. The further studies of model compounds M1 and M2 revealed that they displayed similar AIE behaviours with NPS as shown in Fig. S8.†
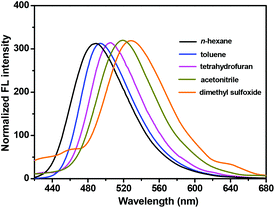 |
| Fig. 9 Normalized emission of NPS in different solvents. | |
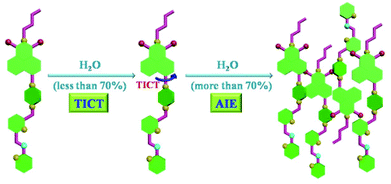 |
| Fig. 10 Proposed mechanisms for the TICT and AIE processes in NPS. | |
Conclusions
In conclusion, a simple and versatile fluorescent dye NPS based on naphthalimide was successfully developed. The structure of NPS was well verified by 1H NMR, 13C NMR, HRMS, and X-ray single-crystal diffraction. It was found that the newly designed probe NPS featured excellent performances in the detection of Hg2+ as well as the TICT and AIE behaviours in aqueous solution. For instance, NPS was highly sensitive and selective in the detection of Hg2+ in aqueous solution with a fluorescence “turn-on” characteristic. The linear fashion of the fluorescence intensity enhancement of NPS with the concentration of Hg2+ indicated that it could be used for quantification of Hg2+ in aqueous solution. Moreover, the detailed sensing mechanism and binding model of NPS with Hg2+ were studied via the absorption and fluorescence titration spectra, the Job's plots, and the comparison with model compounds. The living cell image experiments demonstrated that NPS could be used as a fluorescent probe for monitoring Hg2+ in living cells. Furthermore, it was found that the NPS surprisingly exhibited both TICT and AIE behaviours in aqueous solution depending on the polarity of solvents. Thus this study not only provided a highly efficient sensor for Hg2+ detection in aqueous solution and living cells but also enriched the luminous family of AIE molecules.
Experimental
Synthesis
Compound NPS: Anhydrous potassium carbonate (174 mg, 1.26 mmol), compounds 2 (300 mg, 0.63 mmol) and 2-mercaptopyridine (168 mg, 1.51 mmol) were dissolved in acetone (12 mL), and the reaction mixture was refluxed for 10 h under an argon atmosphere. The mixture was filtered, and the solvent was removed in vacuum to give a yellow solid. The crude product was then chromatographed on silica gel using dichloromethane–methanol (20/1, v/v) as an eluant to afford NPS as a yellow solid. Yield: 254 mg (73%). Mp: 131 °C. 1H NMR (CDCl3, 400 MHz) δ 8.57 (dd, J = 7.2, 1.0 Hz, 1H), 8.51 (d, J = 8.0 Hz, 1H), 8.46–8.41 (m, 1H), 8.38 (d, J = 8.4 Hz, 1H), 7.68 (dd, J = 8.2, 7.4 Hz, 1H), 7.63 (t, J = 8.0 Hz, 1H), 7.51–7.44 (m, 1H), 7.41 (d, J = 7.6 Hz, 2H), 7.21 (t, J = 8.0 Hz, 2H), 7.00–6.96 (m, 1H), 4.60 (s, 2H), 4.16 (t, J = 8.0 Hz, 2H), 3.92 (s, 2H), 3.37 (s, 4H), 2.96 (s, 4H), 1.74–1.65 (m, 2H), 1.49–1.38 (m, 2H), 0.96 (t, J = 7.2 Hz, 3H). 13C NMR (CDCl3, 100 MHz) δ: 164.41, 163.95, 158.35, 157.91, 157.25, 155.79, 149.35, 137.16, 136.00, 132.45, 131.00, 130.19, 129.79, 126.08, 125.61, 123.25, 122.02, 121.78, 121.68, 119.62, 116.76, 114.91, 64.19, 53.23, 52.85, 40.05, 36.01, 30.25, 20.39, 13.88. HR-ESI-MS calcd for C32H34N5O2S [(M + H)+]: 552.2428, found: 552.2445.
The other two model compounds M1 and M2 were synthesized as shown in Schemes S1–S3.†
Acknowledgements
The work was financially supported by the National Natural Science Foundation of China (no. 21302058 and 21322206), the Key Basic Research Project of Shanghai Science and Technology Commission (no. 13JC1402200), Fok Ying Tung Education Foundation (no. 131014), the Research Fund for the Doctoral Program of Higher Education of China (no. 20130076120006), and the Opening Project of Shanghai Key Laboratory of Chemical Biology.
Notes and references
-
(a) A. Renzoni, F. Zino and E. Franchi, Environ. Res., 1998, 77, 68 CrossRef CAS PubMed;
(b) P. Grandjean, P. Weihe, R. F. White and F. Debes, Environ. Res., 1998, 77, 165 CrossRef CAS PubMed;
(c) P. B. Tchounwou, W. K. Ayensu, N. Ninashvili and D. Sutton, Environ. Toxicol., 2003, 18, 149 CrossRef CAS PubMed;
(d) H. H. Harris, I. J. Pickering and G. N. George, Science, 2003, 301, 1203 CrossRef CAS PubMed.
-
(a) T. W. Clarkson, L. Magos and G. J. Myers, N. Engl. J. Med., 2003, 349, 1731 CrossRef CAS PubMed;
(b) J. Mutter, J. Naumann, C. Sadaghiani, R. Schneider and H. Wallach, Neuroendocrinol. Lett., 2004, 25, 331 CAS.
-
(a) J. S. Santos, M. Guardia, A. Pastor and M. L. P. Santos, Talanta, 2009, 80, 207 CrossRef PubMed;
(b) L. Feng and Z. Chen, Sens. Actuators, B, 2007, 122, 600 CrossRef CAS PubMed;
(c) K. H. Chen, H. W. Wang, B. S. Kang, C. Y. Chang, Y. L. Wang, T. P. Lele, F. Ren, S. J. Pearton, A. Dabiran, A. Osinsky and P. P. Chow, Sens. Actuators, B, 2008, 134, 386 CrossRef CAS PubMed.
- For selected reviews on the Hg2+ fluorescent probes, see:
(a) E. M. Nolan and S. J. Lippard, Chem. Rev., 2008, 108, 3443 CrossRef CAS PubMed;
(b) H. N. Kim, W. X. Ren, J. S. Kim and J. Yoon, Chem. Soc. Rev., 2012, 41, 3210 RSC For selected examples on the fluorescent probes for metal ions, see:
(c) H. Li, J. Fan and X. Peng, Chem. Soc. Rev., 2013, 42, 7943 RSC;
(d) L. M. Hyman and K. J. Franz, Coord. Chem. Rev., 2012, 256, 2333 CrossRef CAS PubMed;
(e) S. Anbu, S. Shanmugaraju, R. Ravishankaran, A. Karanda and P. S. Mukherjee, Dalton Trans., 2012, 41, 13330 RSC;
(f) S. K. Sahoo, D. Sharma, R. K. Bera, G. Crisponic and J. F. Callan, Chem. Soc. Rev., 2012, 41, 7195 RSC;
(g) S. Anbu, S. Shanmugaraju, R. Ravishankaran, A. Karanda and P. S. Mukherjee, Inorg. Chem. Commun., 2012, 25, 26 CrossRef CAS PubMed;
(h) A. Bencini and V. Lippolis, Coord. Chem. Rev., 2012, 256, 149 CrossRef CAS PubMed;
(i) H. M. Kim and B. R. Cho, Chem. – Asian. J., 2011, 6, 58 CrossRef CAS PubMed;
(j) K. Jobe, C. H. Brennan, M. Motevalli, S. M. Goldup and M. Watkinson, Chem. Commun., 2011, 47, 6036 RSC;
(k) J. Pancholi, D. J. Hodson, K. Jobe, G. A. Rutter, S. M. Goldup and M. Watkinson, Chem. Sci., 2014, 5, 3528 RSC;
(l) Z. Xu, J. Yoon and D. R. Spring, Chem. Soc. Rev., 2010, 39, 1996 RSC;
(m) M. Beija, C. A. M. Afonso and J. M. G. Martinho, Chem. Soc. Rev., 2009, 38, 2410 RSC;
(n) D. T. Quang and J. S. Kim, Chem. Rev., 2010, 110, 6280 CrossRef CAS PubMed.
-
(a) E. M. Nolan and S. J. Lippard, J. Am. Chem. Soc., 2003, 125, 14270 CrossRef CAS PubMed;
(b) A. B. Descalzo, R. Martínez-Máñez, R. Radeglia, K. Rurack and J. Soto, J. Am. Chem. Soc., 2003, 125, 3418 CrossRef CAS PubMed;
(c) Y.-K. Yang, K.-J. Yook and J. Tae, J. Am. Chem. Soc., 2005, 127, 16760 CrossRef CAS PubMed;
(d) A. Caballero, R. Martínez, V. Lloveras, I. Ratera, J. Vidal-Gancedo, K. Wurst, A. Tárrage, P. Molina and J. Veciana, J. Am. Chem. Soc., 2005, 127, 15666 CrossRef CAS PubMed;
(e) M. H. Lee, J.-S. Wu, J. W. Lee, J. H. Jung and J. S. Kim, Org. Lett., 2007, 9, 2501 CrossRef CAS PubMed;
(f) H. Yang, Z. Zhou, K. Huang, M. Yu, F. Li, T. Yi and C. Huang, Org. Lett., 2007, 9, 4729 CrossRef CAS PubMed;
(g) S. V. Wegner, A. Okesli, P. Chen and C. He, J. Am. Chem. Soc., 2007, 129, 3474 CrossRef CAS PubMed;
(h) S. Yoon, E. W. Miller, Q. He, P. H. Do and C. J. Chang, Angew. Chem., Int. Ed., 2007, 46, 6658 CrossRef CAS PubMed;
(i) X. Zhang, Y. Xiao and X. Qian, Angew. Chem., Int. Ed., 2008, 47, 8025 CrossRef CAS PubMed;
(j) D. Wu, A. B. Descalzo, F. Weik, F. Emmerling, Z. Shen, X.-Z. You and K. Rurack, Angew. Chem., Int. Ed., 2008, 47, 193 CrossRef CAS PubMed;
(k) F. Song, S. Watanabe, P. E. Floreancig and K. Koide, J. Am. Chem. Soc., 2008, 130, 16460 CrossRef CAS PubMed;
(l) M. Santra, D. Ryu, A. Chatterjee, S.-K. Ko, I. Shin and K. H. Ahn, Chem. Commun., 2009, 2115 RSC;
(m) M. Dong, Y.-W. Wang and Y. Peng, Org. Lett., 2010, 12, 5310 CrossRef CAS PubMed;
(n) J. Du, J. Fan, X. Peng, P. Sun, J. Wang, H. Li and S. Sun, Org. Lett., 2010, 12, 476 CrossRef CAS PubMed;
(o) W. Lin, X. Cao, Y. Ding, L. Yuan and L. Long, Chem. Commun., 2010, 46, 3529 RSC;
(p) M. Suresh, A. K. Mandal, S. Saha, E. Suresh, A. Mandoli, R. D. Liddo, P. P. Parnigotto and A. Das, Org. Lett., 2010, 12, 5406 CrossRef CAS PubMed;
(q) C. Chen, R. Wang, L. Guo, N. Fu, H. Dong and Y. Yuan, Org. Lett., 2011, 13, 1162 CrossRef CAS PubMed;
(r) A. Mitra, A. K. Mittal and C. P. Rao, Chem. Commun., 2011, 47, 2565 RSC;
(s) H. Zheng, X.-J. Zhang, X. Cai, Q.-N. Bian, M. Yan, G.-H. Wu, X.-W. Lai and Y.-B. Jiang, Org. Lett., 2012, 14, 1986 CrossRef CAS PubMed;
(t) W. Xuan, C. Chen, Y. Cao, W. He, W. Jiang and W. Wang, Chem. Commun., 2012, 48, 7292 RSC;
(u) J. Jiang, W. Liu, J. Cheng, L. Yang, H. Jiang, D. Bai and W. Liu, Chem. Commun., 2012, 48, 8371 RSC;
(v) K. Bera, A. K. Das, M. Nag and S. Basak, Anal. Chem., 2014, 86, 2740 CrossRef CAS PubMed;
(w) M. Tian, L. Liu, Y. Li, R. Hu, T. Liu, H. Liu, S. Wang and Y. Li, Chem. Commun., 2014, 50, 2055 RSC;
(x) J. W. Lee, H. S. Jung, P. S. Kwon, J. W. Kim, R. A. Bartsch, Y. Kim, S.-J. Kim and J. S. Kim, Org. Lett., 2008, 10, 3801 CrossRef CAS PubMed;
(y) M. H. Lee, S. W. Lee, S. H. Kim, C. Kang and J. S. Kim, Org. Lett., 2009, 11, 2101 CrossRef CAS PubMed.
-
(a) S. Zhang, J. Geng, W. Yang and X. Zhang, RSC Adv., 2014, 4, 12596 RSC;
(b) J. Wen, Z. Geng, Y. Yin and Z. Wang, Dalton Trans., 2011, 40, 9737 RSC;
(c) B. Sen, M. Mukherjee, S. Pal, K. Dhara, S. K. Mandal, A. R. Khuda-Bukhshc and P. Chattopadhyay, RSC Adv., 2014, 4, 14919 RSC;
(d) K. Kanagaraj, K. Bavanidevi, T. J. Chow and K. Pitchumani, RSC Adv., 2014, 4, 11714 RSC;
(e) X. Zhang, X.-J. Huang and Z.-J. Zhu, RSC Adv., 2013, 3, 24891 RSC;
(f) A. Singh, S. Kaur, N. Singh and N. Kaur, Org. Biomol. Chem., 2014, 12, 2302 RSC;
(g) H. Jiang, J. Jiang, J. Cheng, W. Dou, X. Tang, L. Yang, W. Liu and D. Bai, New J. Chem., 2014, 38, 109 RSC;
(h) X. Chen, X. Meng, S. Wang, Y. Cai, Y. Wu, Y. Feng, M. Zhua and Q. Guo, Dalton Trans., 2013, 42, 14819 RSC;
(i) J. Isaad and A. E. Achari, Analyst, 2013, 138, 3809 RSC;
(j) P. Xi, L. Huang, G. Xie, F. Chen, Z. Xu, D. Bai and Z. Zeng, Dalton Trans., 2011, 40, 6382 RSC;
(k) K. Tsukamoto, Y. Shinohara, S. Iwasaki and H. Maeda, Chem. Commun., 2011, 47, 5073 RSC;
(l) M.-H. Yang, C. R. Lohani, H. Cho and K.-H. Lee, Org. Biomol. Chem., 2011, 9, 2350 RSC;
(m) Y.-K. Yang, S.-K. Ko, I. Shin and J. Tae, Org. Biomol. Chem., 2009, 7, 4590 RSC;
(n) H. Lu, L. Xiong, H. Liu, M. Yu, Z. Shen, F. Li and X. You, Org. Biomol. Chem., 2009, 7, 2554 RSC;
(o) D. Zhang, M. Li, M. Wang, J. Wang, X. Yang, Y. Ye and Y. Zhao, Sens. Actuators, B, 2013, 177, 997 CrossRef CAS PubMed;
(p) D. Homraruen, T. Sirijindalert, L. Dubas, M. Sukwattanasinitt and A. Ajavakom, Tetrahedron, 2013, 69, 1617 CrossRef CAS PubMed;
(q) L. N. Neupane and K.-H. Lee, Tetrahedron, 2013, 54, 5007 CrossRef CAS PubMed;
(r) M. J. Culzoni, A. M. de la Peña, A. Machuca, H. C. Goicoechea, R. Brasca and R. Babiano, Talanta, 2013, 117, 288 CrossRef CAS PubMed;
(s) D.-H. Kim, J. Seong, H. Lee and K.-H. Lee, Sens. Actuators, B, 2014, 196, 421 CrossRef CAS PubMed;
(t) J. Zhang, Y. Zhou, W. Hu, L. Zhang, Q. Huang and T. Ma, Sens. Actuators, B, 2013, 183, 290 CrossRef CAS PubMed;
(u) S. Ozturk and S. Atilgan, Tetrahedron Lett., 2014, 55, 70 CrossRef CAS PubMed.
-
(a) X.-J. Zhu, S.-T. Fu, W.-K. Wong, J.-P. Guo and W.-Y. Wong, Angew. Chem., Int. Ed., 2006, 45, 3150 CrossRef CAS PubMed;
(b) S. Tatay, P. Gaviña, E. Coronado and E. Palomares, Org. Lett., 2006, 8, 3857 CrossRef CAS PubMed;
(c) M.-H. Ha-Thi, M. Penhoat, V. Michelet and I. Leray, Org. Lett., 2007, 9, 1133 CrossRef CAS PubMed;
(d) M. G. Choi, D. H. Ryu, H. L. Jeon, S. Cha, J. Cho, H. H. Joo, K. S. Hong, C. Lee, S. Ahn and S.-K. Chang, Org. Lett., 2008, 10, 3717 CrossRef CAS PubMed;
(e) M. Tian and H. Ihmels, Chem. Commun., 2009, 3175 RSC;
(f) R. Koteeswari, P. Ashokkumar, E. J. P. Malar, V. T. Ramakrishnan and P. Ramamurthy, Chem. Commun., 2011, 47, 7695 RSC;
(g) P. Thirupathi and K.-H. Lee, Bioorg. Med. Chem., 2013, 21, 7964 CrossRef CAS PubMed.
-
(a) X. Guo, X. Qian and L. Jia, J. Am. Chem. Soc., 2004, 126, 2272 CrossRef CAS PubMed;
(b) S. Lee, B. A. Rao and Y.-A. Son, Sens. Actuators, B, 2014, 196, 388 CrossRef CAS PubMed;
(c) B. Bag and A. Pal, Org. Biomol. Chem., 2011, 9, 4467 RSC;
(d) Y. Zhao, Z. Lin, C. He, H. Wu and C. Duan, Inorg. Chem., 2006, 45, 10013 CrossRef CAS PubMed.
-
(a) J. Wang, X. Qian and J. Cui, J. Org. Chem., 2006, 71, 4308 CrossRef CAS PubMed;
(b) T. Cheng, T. Wang, W. Zhu, Y. Yang, B. Zeng, Y. Xu and X. Qian, Chem. Commun., 2011, 47, 3915 RSC.
-
(a) C.-B. Huang, H.-R. Li, Y. Luo and L. Xu, Dalton Trans., 2014, 43, 8102 RSC;
(b) L. Xu, Y. Xu, W. Zhu, C. Yang, L. Han and X. Qian, Dalton Trans., 2012, 41, 7212 RSC;
(c) S. Chen, L.-J. Chen, H.-B. Yang, H. Tian and W. Zhu, J. Am. Chem. Soc., 2012, 134, 13596 CrossRef CAS PubMed;
(d) X.-D. Xu, J. Zhang, L.-J. Chen, X.-L. Zhao, D.-X. Wang and H.-B. Yang, Chem. – Eur. J., 2012, 18, 1659 CrossRef CAS PubMed;
(e) X.-D. Xu, J. Zhang, L.-J. Chen, R. Guo, D.-X. Wang and H.-B. Yang, Chem. Commun., 2012, 48, 11223 RSC;
(f) B. Jiang, L.-J. Chen, L. Xu, S.-Y. Liu and H.-B. Yang, Chem. Commun., 2013, 49, 6977 RSC;
(g) L.-J. Chen, G.-Z. Zhao, B. Jiang, B. Sun, M. Wang, L. Xu, J. He, Z. Abliz, H. Tan, X. Li and H.-B. Yang, J. Am. Chem. Soc., 2014, 136, 5993 CrossRef CAS PubMed;
(h) Z.-Y. Li, L. Xu, C.-H. Wang, X.-L. Zhao and H.-B. Yang, Chem. Commun., 2013, 49, 6194 RSC;
(i) N.-W. Wu, J. Zhang, C.-H. Wang, L. Xu and H.-B. Yang, Monatsh. Chem., 2013, 144, 553 CrossRef CAS;
(j) M. He, Q. Han, J. He, Q. Li, Z. Abliz, H. Tan, L. Xu and H. Yang, Chin. J. Chem., 2013, 31, 663 CrossRef CAS PubMed;
(k) L. Xu, M.-L. He, H.-B. Yang and X. Qian, Dalton Trans., 2013, 42, 8218 RSC;
(l) N.-W. Wu, J. Zhang, D. Ciren, Q. Han, L.-J. Chen, L. Xu and H.-B. Yang, Organometallics, 2013, 32, 2536 CrossRef CAS;
(m) Z.-Y. Li, W. Wu, Q. Zhang, B. Jin, J. Hua, H.-B. Yang and H. Tian, Chem. – Asian J., 2013, 8, 2660 CrossRef CAS PubMed;
(n) W. Wu, J. Zhang, H. Yang, B. Jin, Y. Hu, J. Hua, C. Jing, Y. Long and H. Tian, J. Mater. Chem., 2012, 22, 5382 RSC;
(o) L.-J. Chen, J. Zhang, J. He, X.-D. Xu, N.-W. Wu, D.-X. Wang, Z. Abliz and H.-B. Yang, Organometallics, 2011, 30, 5590 CrossRef CAS;
(p) W. Wu, X. Xu, H. Yang, J. Hua, X. Zhang, L. Zhang, Y. Long and H. Tian, J. Mater. Chem., 2011, 21, 10666 RSC;
(q) X.-D. Xu, J. Zhang, X. Yu, L.-J. Chen, D.-X. Wang, T. Yi, F. Li and H.-B. Yang, Chem. – Eur. J., 2012, 18, 16000 CrossRef CAS PubMed.
-
(a) Z. Xu, Y. Xiao, X. Qian, J. Cui and D. Cui, Org. Lett., 2005, 7, 889 CrossRef CAS PubMed;
(b) C. Zhang, Z. Liu, Y. Li, W. He, X. Gao and Z. Guo, Chem. Commun., 2013, 49, 11430 RSC.
-
(a)
J. B. Birks, Photophysics of Aromatic Molecules, Wiley, London, 1970 Search PubMed;
(b)
J. Malkin, Photophysical and Photochemical Properties of Aromatic Compounds, CRC, Boca Raton, 1992 Search PubMed.
-
(a) J. Luo, Z. Xie, J. W. Y. Lam, L. Cheng, H. Chen, C. Qiu, H. S. Kwok, X. Zhan, Y. Liu, D. Zhu and B. Z. Tang, Chem. Commun., 2001, 1740 RSC;
(b) B. Z. Tang, X. Zhan, G. Yu, P. P. S. Lee, Y. Liu and D. Zhu, J. Mater. Chem., 2001, 11, 2974 RSC.
-
(a) Y. Hong, J. W. Y. Lam and B. Z. Tang, Chem. Commun., 2009, 4332 RSC;
(b) Y. Hong, J. W. Y. Lam and B. Z. Tang, Chem. Soc. Rev., 2011, 40, 5361 RSC;
(c) D. Ding, K. Li, B. Liu and B. Z. Tang, Acc. Chem. Res., 2013, 46, 2441 CrossRef CAS PubMed.
- Y. Li, Y. Wu, J. Chang, M. Chen, R. Liu and F. Li, Chem. Commun., 2013, 49, 11335 RSC.
- H. Tong, Y. Dong, M. Haeussler, Y. Hong, J. W. Y. Lam, H. H. Y. Sung, I. D. Williams, H. S. Kwok and B. Z. Tang, Chem. Phys. Lett., 2006, 428, 326 CrossRef CAS PubMed.
- Z. R. Grabowski, K. Rotkiewicz and W. Rettig, Chem. Rev., 2003, 103, 3899 CrossRef PubMed.
Footnotes |
† Electronic supplementary information (ESI) available. CCDC 1011046. For ESI and crystallographic data in CIF or other electronic format see DOI: 10.1039/c4qo00185k |
‡ H. Un and C. Huang contributed equally to this work. |
|
This journal is © the Partner Organisations 2014 |
Click here to see how this site uses Cookies. View our privacy policy here.