DOI:
10.1039/C3RA44066D
(Paper)
RSC Adv., 2014,
4, 4872-4877
Monodisperse polylactide microcapsules with a single aqueous core prepared via spontaneous emulsification and solvent diffusion†
Received
1st August 2013
, Accepted 9th December 2013
First published on 10th December 2013
Abstract
A simple approach to preparing monodisperse poly(D,L-lactide) (PDLLA) microcapsules with a single aqueous core is developed. The method is based on automatic water-in-oil-in-water double emulsion formation from oil-in-water single emulsion via spontaneous emulsification which voluntarily disperses part of continuous aqueous phase into the dispersed oil phase dissolving oil-soluble amphiphilic diblock copolymer, poly(D,L-lactide)-b-poly(2-dimethylaminoethyl methacylate)(PDLLA-b-PDMAEMA), followed by coalescence of tiny water droplets within the polymer droplets, coupled with quick precipitation of polymers by diluting the emulsion with water. In this study, we have investigated the effect of PDLLA to PDLLA-b-PDMAEMA ratios and flow rates of each solution during preparing the emulsion on the final morphology and the size of the microcapsules. It was found that the polymer blend ratio played a crucial role in determining internal structure of the microcapsules. The microcapsules size decreased with the increment of the flow rate ratios of the continuous phase to the dispersed phase and eventually reached 10 μm, while maintaining narrow size distribution. In addition, we have demonstrated that the microcapsules can encapsulate both hydrophilic and hydrophobic compounds during the formation.
1. Introduction
Biodegradable polymeric microcapsules having a large hollow space are of great interest because of their potential for encapsulating vast quantities of large guest materials within their empty cores. The microcapsules can be applied to various applications including drug delivery carriers for sustained release,1 personal care ingredients, agrochemicals,2 and medical imaging agents.3 Many fabrication techniques for the microcapsules have been developed such as internal phase separation of emulsion droplets,4 water-in-oil-in-water (W/O/W) emulsion formation, coupled with solidification techniques (e.g. solvent evaporation5 and UV or heat polymerization6), miniemulsion polymerization,7 layer-by-layer assembly,8 interfacial polymerisation of polymer droplets,9 supramolecular self-assembly,10 and surface-initiated atom transfer radical polymerization on template colloidal particles.11
Regarding preparation of poly(D,L-lactide) (PDLLA) microcapsules with aqueous cores, many studies have been demonstrated by W/O/W emulsion-solvent evaporation method.12 In this method, the microcapsules are produced via evaporation of volatile organic solvent from the prefabricated complex emulsion droplets. The complex emulsion droplets are typically produced in a two-step emulsification, including water-in-oil (W/O) emulsion formation by emulsifying the inner aqueous phase in the middle oil phase, and the second emulsification with the W/O emulsion and the external surfactant aqueous solution. However, each emulsification process results in polydisperse emulsion droplets, leading to microcapsules with poorly controlled internal structures and broader size distribution.
Recently, coaxial microcapillary devices have enabled to produce highly controlled double emulsions and the microcapsules in a continuous manner.13 However, the fabrication of the devices is difficult because it requires shaping and manual alignment of microcapillaries. In addition, to keep periodicity of the double emulsions formation, one must precisely control the flow rates of the innermost, middle, and outermost fluids, which gives rise to limitation on production of smaller microcapsules less than 50 μm. In addition, after the droplet formation, it takes several minutes to hours to solidify microcapsules via evaporation of organic solvent, which is a rate-determining step in the whole process. Therefore, simple preparation of monodisperse PDLLA microcapsules with tunable size and internal structure using a simple microfluidic device is still challenging.
In this report, we present a straightforward method for the preparation of monodisperse PDLLA microcapsules with a single aqueous core without any operation of complex fluids. This technique is based on “droplet-to-particle” technology consisting of microfluidic emulsification and solvent diffusion, with some modification, previously reported by our group.14 Monodisperse oil-in-water (O/W) emulsion droplets were used in this study and oil-soluble amphiphilic diblock copolymer was introduced into the organic phase to induce spontaneous emulsification,15 dispersing part of continuous aqueous phase into the dispersed oil phase. This process has enabled to convert O/W emulsion into W/O/W emulsion automatically. By using ethyl acetate (EA) as the organic solvent, solidification of the droplets was easily achieved by just diluting the emulsion with excess volume of pure water because of high solubility of EA in water (8.3 wt% at 20 °C) and microcapsules with a single aqueous core were eventually formed. This quick removal of EA from the droplets shortens the preparation time about several hundreds times compared with conventional techniques using halogenated solvents. In addition, all components that we use in the microcapsule formation are biocompatible, which is great advantageous to applications for biomedical field. Furthermore, easy flow control using a simple Y-shaped microfluidic device makes it possible to prepare monodisperse microcapsules as small as 10 μm in the diameter. To the best of our knowledge, this is the first report to fabricate monodisperse PDLLA microcapsules with a single aqueous core using spontaneous emulsification via amphiphilic diblock copolymer and subsequent solvent diffusion.
2. Experimental section
Materials
Poly(D,L-lactide) (PDLLA) and poly(ethylene glycol)-b-poly(D,L-lactide) (PEG-b-PDLLA) were synthesized by ring-opening polymerization of D,L-lactide in the presence of tin(II) 2-ethylhexanoate as a catalyst using lauryl alcohol and poly(ethylene glycol) monomethyl ether (PEG, Mn = 4000, Mw/Mn = 1.06) as an initiator, respectively. The D,L-lactide was purchased from Purac (Netherlands). The PEG was kindly supplied from NOF (Japan). Tin(II) 2-ethylhexanoate (Sn(Oct)2), ethyl acetate (EA), ethylene glycol, triethylamine (TEA), chloroform, 0.1 M HCl aqueous solution, sodium bicarbonate (NaHCO3), anhydrous magnesium sulfate (MgSO4), toluene, 2-propanol, N,N,N′,N′′,N′′-pentamethyldiethylenetriamine (PMDETA), tetrahydrofuran (THF), n-hexane, basic alumina, neutral activated alumina, dimethylformamide (DMF), CuCl(I), and 2-(dimethylamino)ethylmethacrylate (DMAEMA) were obtained from Wako Pure Chemical Industries, Ltd. (Japan). 2-Bromoisopropionyl bromide was purchased from Sigma-Aldrich, Ltd. (Japan). DMAEMA was passed through a column of basic alumina to remove stabilizing agents and stored under argon atmosphere at −20 °C. CuCl(I) was purified by washing with glacial acetic acid until the liquid being colourless followed by ethanol in a Schlenk flask under argon atmosphere. The ultra pure water was produced by a Millipore Milli-Q purification system (EMD Millipore Corporation, USA).
Synthesis of 2-hydroxyethyl 2-bromopropionate (HEBP)
2-Bromoisopropionyl bromide (0.04661 mol) was added dropwise to a cold solution of ethylene glycol (0.99276 mol) and triethylamine (0.04357 mol) at 0 °C for 1 h. The reaction was continued at 0 °C for another 2 h and then heated to 40 °C for 5 h. The reaction mixture was cooled, added to 200 mL of water, and extracted with chloroform five times, and then the organic layer was washed successfully with 0.1 M HCl aqueous solution, saturated NaHCO3 aqueous solution, and water. The organic layer was then dried over MgSO4 and evaporated by a rotary evaporator to precipitate a product. The yield was 63%.
Synthesis of bromine-terminated polylactide macroinitiator (PDLLA-Br)
PDLLA macroinitiator, PDLLA-Br, was synthesized by ring-opening polymerization of D,L-lactide using HEBP as an initiator in the presence of Sn(Oct)2 as a catalyst. D,L-Lactide (12.0 g, 83.3 mmol), HEBP (230 mg, 1.17 mmol), and a toluene solution of Sn(Oct)2 (20.8 mg mL−1, 50 μL) were placed into an glass ampoule, which was sealed under reduced pressure and immersed in an oil bath at 130 °C. The polymerization was carried out for 24 h. After cooling, the reaction mixture was dissolved in chloroform and excess 2-propanol was added. The precipitated polymer was recovered and washed with 2-propanol three times. The product was dried under reduced pressure over night. The product yield was 80%.
Synthesis of polylactide-b-poly(2-dimethylaminoethylmethacrylate) (PDLLA-b-PDMAEMA)
The diblock copolymer was synthesized by atom transfer radical polymerization of DMAEMA using the PDLLA-Br as a macroinitiator. The synthesis of the diblock copolymer was performed in DMF at 60 °C in the presence of CuCl/PMDETA catalyst system under argon atmosphere. CuCl(I) (13.7 mg, 0.14 mmol) and PDLLA-Br (Mn = 9600, Mw/Mn = 1.15, 1.3286 g, 0.14 mmol) were placed in a Schlenk flask and then purged by three repeated vacuum/argon cycles. Degassed DMF (6 mL) was then added to the solution to dissolve the macroinitiator and the catalyst. After that DMAEMA (0.81 mL, 5.5 mmol) and PMDETA (28.9 μL, 0.14 mmol) were injected in the flask. The flask was immersed in an oil bath at 60 °C. The polymerization was performed for 12 h. Then, the reaction flask was cooled down to room temperature and the solution was diluted with excess amount of THF. The copper catalyst was extracted by passing the diluted solution through a column of neutral activated alumina. The purified copolymer was precipitated in n-hexane three times, filtered off, and dried under reduced pressure at 40 °C overnight. The product yield was 71%.
Gel permeation chromatography (GPC) analysis
The molecular weight distribution (Mw/Mn) analysis of the synthesized polymers was performed with GPC (HLC-8220GPC, TOSOH, Japan) at 40 °C, equipped with a refractive index detector and columns (TSKguardcolumn SuperH-H, TSKgel SuperHM-H, and TSKgel SuperH2000, TOSOH, Japan). THF was used as the eluent at a flow rate of 0.6 mL min−1. The calibration curve was prepared by using monodisperse polystyrene standards.
1H NMR analysis
Chemical structures of the synthesized initiator and polymers were determined from 1H NMR spectra. The analysis was carried out using a JEOL FT NMR System (JMN-AL300, JEOL, Japan) at room temperature in CDCl3. The number averaged molecular weights (Mn) of PDLLA-Br and PDLLA-b-PDMAEMA were calculated from 1H NMR spectra.
Preparation of monodisperse PDLLA microcapsules
A microfluidic device used in this study consists of Y-shaped channel (126 μm- and 136 μm-width channels with 75 μm in depth) made by SUS and a glass plate. The continuous aqueous phase and the dispersed organic phase were pumped independently at adjustable flow rates using syringe pumps connected to the device via Teflon tubing. The aqueous solution saturated with EA containing 1 wt% of water-soluble-PEG-b-PDLLA (w-PEG-b-PDLLA, Mn = 4400, Mw/Mn = 1.05, hydrophile-lipophile balance (HLB) = 18.2) was used as the continuous phase and an EA solution composed of PDLLA (Mn = 9600, Mw/Mn = 1.31) and PDLLA-b-PDMAEMA (Mn = 16
200, Mw/Mn = 1.28) was used as the dispersed organic phase. The PDLLA to PDLLA-b-PDMAEMA ratio was varied from 10/0 to 6/4 (w/w). The flow rates of the dispersed phase and the continuous phase were 120 μL h−1and 1.5–15 mL h−1, respectively. For fluorescence microscopy, a trace amount of Nile red and 1 mg mL−1 of calcein were added to the dispersed phase and the continuous phase, respectively. The obtained O/W emulsion was transferred to a bath filled with 100 mL of ultra pure water through Teflon tubing (Φ = 0.5 mm, L = 20 cm) whose exit tip was submerged in the water. The EA was then rapidly removed from the droplet to the large volume of pure water by solvent diffusion under gentle stirring at 120 rpm, leading to precipitation of PDLLA microcapsules. The microcapsules were washed with ultra pure water three times by centrifugation (himac CF 15R, Hitachi, Japan) (3000 rpm, 3 min) to remove the surfactant and unloaded fluorescence agents, followed by freeze-drying overnight, resulting in dried PDLLA microcapsules.
Observation of inner water droplets ripening and formation of microcapsules during solvent diffusion
The dispersed organic phase (100 μL) and the continuous aqueous phase (1 mL) were mixed and then shaken by hand for a few seconds to prepare O/W emulsion. The dispersed phase was an EA solution dissolving PDLLA, PDLLA-b-PDMAEMA (at PDLLA/PDLLA-b-PDMAEMA ratio = 6/4 (w/w)) and trace amount of oil-blue N. The continuous phase was 1 wt% w-PEG-b-PDLLA aqueous solution saturated with EA. After emulsification, small portion of the emulsion was placed on a glass plate and covered with a thin cover slip. The prepared specimen was set up on the stage of optical microscope. To induce solvent diffusion of EA into aqueous phase, pure water was introduced into the gap between the glass slide and the cover slip using a disposal syringe. Using optical microscope, ripening of inner water droplets and the following microcapsule formation process were observed.
Optical microscopy
Microcapsule dispersion before washing was dropped on a glass slide and it was then observed with an optical microscope (BX50, OLYMPUS, Japan) equipped with a digital camera (CS230, OLYMPUS, Japan).
Fluorescence microscopy
Calcein and Nile red-laden microcapsule dispersion was dropped on a glass plate. The sample was observed by a fluorescence microscope (All-in-one type BZ-8000, Keyence, Japan) to visualize the location of the encapsulated compounds. The red fluorescence derived from Nile red was observed with a TRITC filter (OP-66837, Keyence, Japan) (excitation wave length = 540 nm and absorption wave length = 605 nm). The green fluorescence derived from calcein was observed with a GFP-BP filter (OP-66836, Keyence, Japan) (excitation wave length = 470 nm and absorption wave length = 535 nm).
Scanning electron microscopy
Morphology of the microcapsules after freeze-drying was observed by a scanning electron microscope (SEM, S-4700, Hitachi Ltd., Japan) at intensity of 1 kV under various magnifications. A sputter-coater (E-1030 Ion-Sputter, Hitachi Ltd., Japan) was used to coat the samples with Pd–Pt to prevent the samples from charge up. Before the observation, the freeze-dried samples were stored in a desiccator.
Results and discussion
In this study, two kinds of polymers, PDLLA (Mn = 9600, Mw/Mn = 1.31) and oil-soluble amphiphilic diblock copolymer, PDLLA-b-PDMAEMA (Mn = 16
200, Mw/Mn = 1.28) were dissolved in EA, which was used as the dispersed phase. PDLLA-b-PDMAEMA was synthesized by atom transfer radical polymerisation of DMAEMA initiated from PDLLA macroinitiator (Scheme S1 and Fig. S1 in ESI†) and used as a co-surfactant to induce spontaneous emulsification within the organic droplets. For the continuous phase, an aqueous solution saturated with EA dissolving 1 wt% w-PEG-b-PDLLA (Mn = 4400, Mw/Mn = 1.04) was used as a surfactant solution.14 As shown in Fig. 1, monodisperse O/W emulsion droplets were formed in a Y-shaped microfluidic device (Fig. S2 in ESI†). The resultant polymer droplets travelled towards the downstream of the channel, where water molecules are extracted from the continuous aqueous phase into the polymer droplets through the EA/water interface via spontaneous emulsification, resulting in forming W/O/W emulsion with multiple tiny aqueous cores. Then the W/O/W emulsion droplets were diluted with 100 mL of pure water in the precipitation bath, which facilitated extraction of EA into the outer aqueous phase and coalescence of tiny water droplets within each emulsion droplet. Fig. 2 shows optical micrographs illustrating the ripening of inner aqueous droplets in an oil droplet during solvent diffusion. It should be noted that because of high volatility of EA and quick diffusion of EA into the aqueous phase within a few seconds, it was difficult to observe the ripening process using microfluidic approach in situ. Therefore, instead of this, the monitoring was carried out using the model emulsion prepared by hand shaking on a glass slide covered with a thin cover slip. We induced the EA diffusion by adding pure water into the system step-by-step. The result clearly shows that as the solvent diffusion proceeds, the volume of the emulsion droplet (coloured blue) gradually decreases and simultaneously, tiny water droplets (white) in the emulsion droplet become bigger and eventually form a single core. When using microfluidic approach, it is considered that monodisperse microcapsules would be formed in the collection bath under the same process, within a few seconds after onset of solvent diffusion. The detailed mechanism will be discussed later.
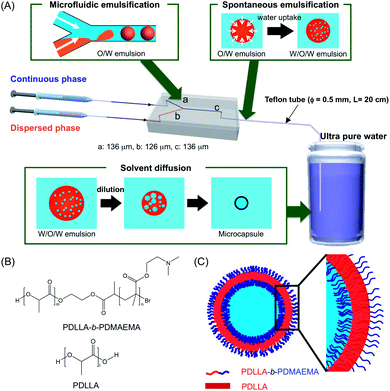 |
| Fig. 1 (A) Schematic illustration of monodisperse microcapsule fabrication process using microfluidic emulsification, spontaneous emulsification, followed by solvent diffusion. (B) Chemical structures of PDLLA-b-PDMAEMA and PDLLA. (C) Schematic illustration of the final microcapsules, the shell of which is composed of PDLLA-b-PDMAEMA and PDLLA. | |
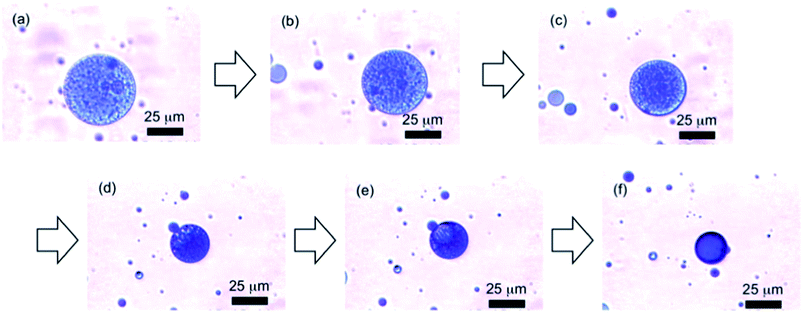 |
| Fig. 2 Optical micrographs of inner water droplets ripening, followed by formation of the microcapsules during EA diffusion into the continuous phase. (a) Polydisperse model emulsion droplets soon after emulsification by shake by hand. The organic phase (blue region) already had many small water droplets (white dots). (b–e) After introduction of small amount of pure water from the left side of the image, rapid reduction of organic phase in size and evolution of inner water droplets were observed. (f) Microcapsule with a single aqueous core was eventually formed. | |
We investigated the effect of blend ratio of PDLLA/PDLLA-b-PDMAEMA on microcapsule structures. Fig. 3 shows optical micrographs and SEM images of the resultant microcapsules prepared by varying PDLLA/PDLLA-b-PDMAEMA ratios. When using only PDLLA, we obtained monodisperse microparticles with a compact internal structure (Fig. 3(a)), whilst monodisperse microcapsules with dark contrast were obtained at the ratio of 9/1 (Fig. 3(b)). The dark contrast means that there are many cores inside the microcapsules. With an increase of the ratio to 6/4, we obtained the microcapsules with a single aqueous core (Fig. 3(c)). In addition, the diameter of the microcapsules was increased with increasing the copolymer ratio, indicating that the polymer droplets extracted water molecules from the outer aqueous phase during the process.
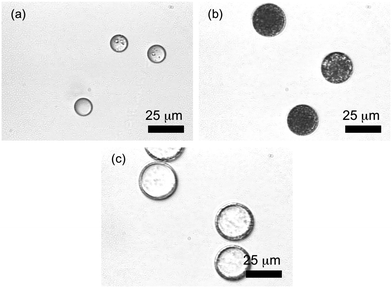 |
| Fig. 3 Effect of the blend ratio of PDLLA/PDLLA-b-PDMAEMA on microcapsule structures. The ratio was (a) 10/0, (b) 9/1 and (c) 6/4 (w/w). | |
Using the ratio at 6/4, we tuned the diameter of the microcapsules by altering the flow rate of the continuous phase (Qc) whilst maintaining that of the dispersed phase (Qd) constant. As shown in Fig. 4, the diameter of the microcapsules decreased from 32.6 to 10.3 μm with increasing the Qc. It was also found that the coefficient of variation (CV) values were increased as increasing the Qc, which was due to unstable emulsification in the device when the Qc was increased. However, the CV values were kept at less than 10% regardless of the flow conditions, indicating that the microcapsules had relatively narrow size distribution.
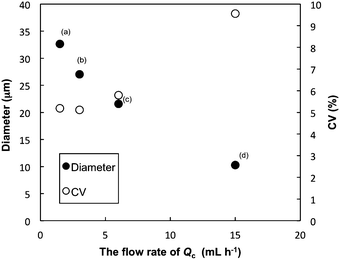 |
| Fig. 4 Effect of the Qc on the microcapsule diameter and the CV value. The Qc was (a) 1.5, (b) 3.0, (c) 6.0 and (d) 15 mL h−1 while the Qd was fixed at 120 μL h−1. | |
Fig. 5 shows the optical micrographs and the SEM images of the microcapsules prepared by changing the flow rates upon emulsification. The results clearly revealed that the microcapsules had a single aqueous core regardless of the microcapsule size. These results indicate that our technique can tune microcapsule size in a controlled manner. It is also important to note that our technique has enabled to produce monodisperse microcapsules with less than 30 μm in the diameter, which is almost impossible when using microcapillary devices since the microcapsule diameter greatly depends on the diameter of the initial W/O emulsion droplets. From the point of view of the microcapsule size, our technique is especially useful for preparing monodisperse microcapsules with less than 50 μm.
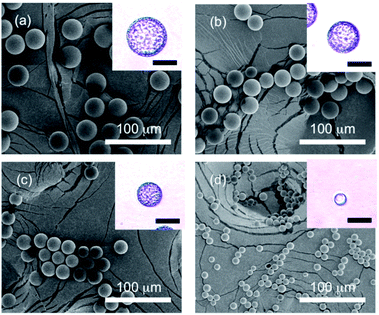 |
| Fig. 5 Optical and SEM images of the microcapsules prepared by varying Qc at a fixed Qd (120 μL h−1). Qc = (a) 1.5, (b) 3.0, (c) 6.0 and (d) 15 mL h−1. The inset scale bars represent 25 μm. | |
Furthermore, we achieved encapsulating both hydrophilic compound and hydrophobic compounds in the microcapsules. As a hydrophilic compound, we dissolved calcein in the continuous phase and a hydrophobic compound, Nile red, was also dissolved in the dispersed phase. As shown in Fig. 6, the core of the microcapsules clearly displayed green fluorescence derived from calcein, implying that the water-uptake from the continuous phase would occur during which the polymer droplets travel towards the downstream of the channel. On the other hand, the shell of the microcapsules showed red fluorescence attributing to Nile red, indicating that hydrophobic compounds dissolved in the dispersed phase can be easily entrapped within the shell of the microcapsules. It was confirmed that the time scale for the release of these encapsulated species in pure water at pH = 7 was long and the fluorescence of both dyes was observed for more than 3 days, implying that the release rate of the substances from the microcapsules is very slow. We will investigate the detailed release profile of the encapsulated substances in the future.
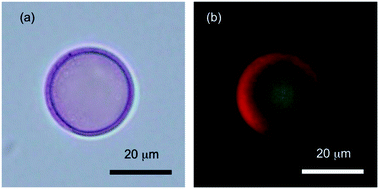 |
| Fig. 6 Fluorescence micrographs of the microcapsules encapsulating calcein in the core and Nile red in the shell. (a) Bright image and (b) overlay image of green and red fluorescence filtered images. | |
Finally, we propose the microcapsule formation mechanism. Monodisperse O/W emulsion whose interface is stabilized by w-PEG-b-PDLLA is firstly produced at the Y-junction in the microfluidic device (Fig. 7(i)). Then water molecules in the continuous phase are extracted to the polymer droplets in the device through the O/W emulsion interface to form tiny water droplets stabilized by amphiphilic PDLLA-b-PDMAEMA within the emulsion droplets. Since the copolymer dissolves in EA as a reverse micellar structure with a hydrophilic PDMAEMA core and a hydrophobic PDLLA shell so as to minimize the interfacial energy, the hydrophilic PDMAEMA core is capable of entrapping aqueous molecules (Fig. 7(ii)). After that, when the droplets are brought into contact with a great amount of pure water, EA diffusion from emulsion droplets to the surrounding pure water phase quickly occurs due to its higher solubility in water, leading to the volume reduction of the emulsion droplets. Simultaneously, an increase in PDLLA-b-PDMAEMA concentration within EA droplets causes microemulsion instability because of its lower solubility in EA, facilitating ripening of inner water droplets within each EA droplet (Fig. 7(iii)). Further evolution of the inner water droplets results in bigger ones, and eventually forms double emulsion (Fig. 7(iv)). Continuous diffusion of EA into the outer aqueous phase leads to precipitation of the polymers from the interface between the emulsion droplets and the continuous phase, yielding monodisperse microcapsules with a single aqueous core (Fig. 7(v)). Due to rapid extraction of EA into the precipitation bath, the transition from the emulsion to the solid microcapsules has completed within a few seconds, which is great advantageous to continuous production of the microcapsules using microfluidics.
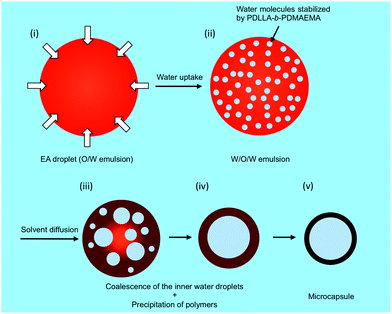 |
| Fig. 7 Proposed microcapsule formation mechanism using spontaneous emulsification and solvent diffusion. | |
Conclusions
We have described a facile and continuous process for fabricating monodisperse PDLLA microcapsules with a single aqueous core through spontaneous emulsification and solvent diffusion, coupled with microfluidic system. This technique does not require any complex fluids operations. The size of the microcapsule was readily controlled by the flow rates on the emulsion preparation, resulting in the microcapsules with 10 to 33 μm in the diameter. The microcapsules were capable of encapsulating either hydrophobic or hydrophilic compounds during the formation. Further study will be performed to apply this technique to integrated microfluidic system that contains in situ solvent diffusion unit and fabrication of advanced functional materials. We believe that this process has great potential for producing polymeric microcapsules applied to biomedical applications.
Acknowledgements
This work was partly supported by Industrial Technology Research Grant Program in 2011 from New Energy and Industrial Technology Development Organisation (NEDO) of Japan.
Notes and references
- J. K. Oh, Soft Matter, 2011, 7, 5096 RSC; T. Ishihara, T. Kubota, T. Choi, M. Takahashi, E. Ayano, H. Kanazawa and M. Higaki, Int. J. Pharm., 2009, 375, 148 CrossRef CAS PubMed.
- T. Takei, M. Yoshida, K. Yanagi, Y. Hatate, K. Shiomori and S. Kiyoyama, Polym. Bull., 2008, 61, 119 CrossRef CAS PubMed.
- E. Pisani, N. Tsapis, B. Galaz, M. Santin, R. Berti, N. Taulier, E. Kurtisovski, O. Lucidarme, M. Ourevitch, B. T. Doan, J. C. Beloeil, B. Gillet, W. Urbach, S. L. Bridal and E. Fattal, Adv. Funct. Mater., 2008, 18, 2963 CrossRef CAS.
- M. Yasukawa, E. Kamio and T. Ono, ChemPhysChem, 2011, 12, 263 CrossRef CAS PubMed; Y. Zhao, J. Fickert, K. Landfester and D. Crespy, Small, 2012, 8, 2954–2958 CrossRef PubMed; T. Watanabe, Y. Kimura and T. Ono, Langmuir, 2013, 29, 14082 CrossRef PubMed.
- S.-H. Kim, J. W. Kim, D.-H. Kim, S.-H. Han and D. A. Weitz, Small, 2013, 9, 124 CrossRef CAS PubMed.
- B. Kim, H. S. Lee, J. Kim and S.-H. Kim, Chem. Commun., 2013, 49, 1865 RSC.
- W. Li, J. A. Yoon and K. Matyjaszewski, J. Am. Chem. Soc., 2010, 132, 7823 CrossRef CAS PubMed.
- K. Kondo, T. Kida, Y. Ogawa, Y. Arikawa and M. Akashi, J. Am. Chem. Soc., 2010, 132, 8236 CrossRef CAS PubMed; A. J. Khopade and F. Caruso, Chem. Mater., 2004, 16, 2107 CrossRef.
- B. Jiang, C. Gao and J. Shen, Colloid Polym. Sci., 2006, 284, 513 CAS.
- J. Zhang, R. J. Coulston, S. T. Jones, J. Geng, O. A. Scherman and C. Abell, Science, 2012, 335, 690 CrossRef CAS PubMed; C. A. Serra, B. Cortese, I. U. Khan, N. Anton, M. H. J. M. Croon, V. Hessel, T. Ono and T. Vandamme, Macromol. React. Eng., 2013, 7, 414 CrossRef.
- Z. Ma, X. Jia, J. Hu, G. Zhang, F. Zhou, Z. Liu and H. Wang, Langmuir, 2013, 29, 5631 CrossRef CAS PubMed.
- Y.-Y. Yang, T.-S. Chung and N. P. Ng, Biomaterials, 2001, 22, 231 CrossRef CAS.
- H. C. Shum, J.-W. Kim and D. A. Weitz, J. Am. Chem. Soc., 2008, 130, 9543 CrossRef CAS PubMed; H. C. Shum, Y.-J. Zhao, S.-H. Kim and D. A. Weitz, Angew. Chem., Int. Ed., 2011, 50, 1648 CrossRef PubMed; S.-W. Choi, Y. Zhang and Y. Xia, Adv. Funct. Mater., 2009, 19, 2943 CrossRef PubMed; F. Tu and D. Lee, Langmuir, 2012, 28, 9944 CrossRef PubMed; M. Windbergs, Y. Zhao, J. Heyman and D. A. Weitz, J. Am. Chem. Soc., 2013, 135, 7933 CrossRef PubMed.
- T. Watanabe, T. Ono and Y. Kimura, Soft Matter, 2011, 7, 9894 RSC.
- C. A. Miller, Colloids Surf., 1988, 29, 89 CrossRef CAS; X. Yu, Z. Zhao, W. Nie, R. Deng, S. Liu, R. Liang, J. Zhu and X. Ji, Langmuir, 2011, 27, 10265 CrossRef PubMed.
Footnote |
† Electronic supplementary information (ESI) available: NMR spectra, optical microphotograph of the emulsion and fluorescence microphotograph of the microcapsules. See DOI: 10.1039/c3ra44066d |
|
This journal is © The Royal Society of Chemistry 2014 |
Click here to see how this site uses Cookies. View our privacy policy here.