DOI:
10.1039/C3RA46554C
(Paper)
RSC Adv., 2014,
4, 25692-25697
In2O3 nanostructures: synthesis and chlorobenzene sensing properties
Received
10th November 2013
, Accepted 28th April 2014
First published on 28th April 2014
Abstract
In this work, In2O3 nanorods and nanoparticles have been successfully prepared by solvothermal methods followed by annealing of the precursors at 300 °C. Experimental results show that the morphology and phase are strongly influenced by the solvents used. Moreover, we have developed gas sensors based on ultrathin In2O3 nanorods, which demonstrate excellent o-dichlorobenzene and chlorobenzene sensing properties, superior to those of nanoparticles with a similar size. It is expected that our as-synthesized In2O3 nanorods could potentially be applied in real-time monitoring of polychlorobenzene concentrations in the future.
Introduction
Gas sensors are thought to be one kind of functional detector which could be used for the real-time detection of gases over a wide temperature range.1–4 The performance of gas sensors is strongly dependent on the native composition, size and shape of the sensing materials.5–7 To date, metal oxides such as Fe2O3,5–8 NiO,9–12 SnO2,13–16 CuO,17–19 TiO2,20,21 Co3O4,22,23 WO3,24–26 In2O3,27–42 and so on, have been intensively studied as sensing materials. Among them, In2O3 is one of the most studied gas sensing materials, which is sensitive to a variety of gases (ethanol, H2, CO, CH3OH, NOx, NH3 and H2S) due to its unique characteristics.27–42 For example, some composites such as ZnO–In2O3 nanofibers,30 Bi2O3-core/In2O3-shell nanorods,31 SnO2/In2O3 hetero-nanofibers,32 Pt and Pd nanoparticle decorated In2O3 flower-like nanobundles,33 etc., have shown excellent gas sensing performance for various gases. In addition, different In2O3 nanostructures including nanorods,34,35 nanowires,36–38 hollow nano- and microspheres,39–41 other structures,42 etc., have been reported to display better gas sensing performance. Among these nanostructures, one-dimensional (1D) nanostructures, nanorods or nanowires, are promising for us to detect toxic gases with satisfactory performance.6,15 Therefore, obtaining 1D nanostructures, especially with small sizes, is of extreme importance for us to obtain high-performance sensing materials.
In the past decade, the preparation of In2O3 nanostructures has attracted a great deal of attention due to their wide range of applications.27–29,43–46 With regard to 1D nanostructures, there are also many reports on synthetic techniques, such as laser-ablation chemical vapor deposition (CVD),47 CVD,48–50 Au-catalyzed vapor–liquid–solid (VLS),51 hydrogen assisted thermal evaporation,52 phase transformation from InOOH to In2O3 nanowires through lanthanide doping,53 thermal evaporation with an Au catalyst,38 thermal evaporation and condensation,54 epitaxial growth,55 electrospinning,36 and so on. However, most of these methods have drawbacks such as needing high temperatures, noble metal catalysts, substrates, high amounts of energy, etc. 1D In2O3 nanostructures with diameters below 5 nm have not often been obtained by facile methods.47 Therefore, it is still a big challenge for us to obtain ultrathin 1D In2O3 nanostructures by facile methods.
In this work, we report a facile solvothermal route to prepare ultrathin InOOH nanorods and then convert them into the corresponding In2O3 nanorods. We also report a chlorobenzene and o-dichlorobenzene sensor with high sensitivity and a rapid response using single crystalline In2O3 nanorods as the active material. The results indicate that the enhanced chlorobenzene and o-dichlorobenzene sensing performance can be attributed to the rod-like morphology due to the advantages of the effective adsorption and rapid diffusion of the chlorobenzene and o-dichlorobenzene molecules.
Experimental
Synthesis of In2O3 nanostructures
In a typical synthesis of In2O3 nanorods, 2 mmol of In(NO3)3·5H2O was dissolved into 35 ml of diethylenetriamine, and then transferred to a 45 ml Teflon-lined stainless steel autoclave at 150 °C for 20 h, followed by cooling the autoclave down to room temperature naturally. White precipitate was collected by centrifugation, washed with distilled water, absolute alcohol and acetone several times, followed by drying at room temperature for 24 h. Finally, yellow In2O3 nanorods were obtained through annealing the above InOOH sample in air at 300 °C for 2 h with a heating rate of 2 °C min−1, and cooling in air.
The process and parameters of synthesizing In2O3 nanoparticles were the same as for the InOOH and In2O3 nanorods, except the solvent was changed to 5 ml of distilled water and 30 ml of ethanolamine.
Characterization
The morphology and structural characteristics were observed using X-ray diffraction (XRD, Rigaku D/max 2500 diffractometer), scanning electron microscopy (SEM, Hitachi S4800), transmission electron microscope (TEM, JEOL 2010 with an accelerating voltage of 200 kV).
Gas sensing measurements
The fabrication and testing principles of the gas sensor are similar to those described in our previous reports.5,6 Firstly, the as-synthesized In2O3 samples were mixed with terpineol to form a paste and then coated onto the outside surface of an alumina tube with a diameter of 1 mm and a length of 5 mm. A platinum coil through the tube was employed as a heater to control the operating temperature. To improve their stability and repeatability, the gas sensors were aged at 300 °C for 10 h in air. Here, the sensing properties of the sensors were measured by a NS-4003 series gas sensing measurement system (China Zhong-Ke Micro-nano IOT, Internet of Things, Ltd.). The relative humidity (RH) was about 45%. The response and recovery times were defined as the time required for a change of the resistance to reach 90% of the equilibrium value after injecting and removing the detected gas, respectively.
InOOH nanorods were synthesized through the hydrolysis of In(NO3)3·5H2O in diethylenetriamine under solvothermal conditions at 150 °C. The XRD pattern of the as-obtained orthorhombic InOOH nanorods can be well matched with the standard card (JCPDS: 17-0549), as shown in Fig. 1a. The morphology of the as-obtained InOOH nanorods is shown in Fig. 2a and b, which show that the nanorods have a diameter of about 4 nm and a length ranging from 15 to 30 nm. The chemical route to InOOH nanorods in diethylenetriamine can be suggested as eqn (a) and (b):
|
In3+ + 3H2O → In(OH)3 + 3H+
| (a) |
|
In(OH)3 → InOOH + H2O
| (b) |
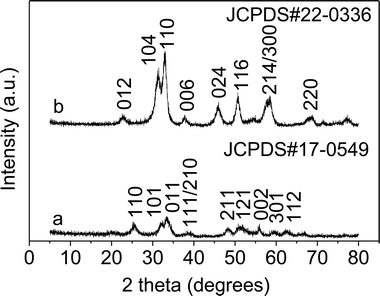 |
| Fig. 1 XRD patterns of the as-obtained nanorods: (a) InOOH, and (b) In2O3. | |
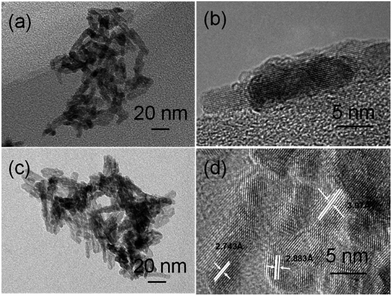 |
| Fig. 2 TEM images of the as-obtained nanorods: (a and b) InOOH, and (c and d) In2O3. | |
The growth of the InOOH nanorods strongly depends on the solvent used, which indicates that diethylenetriamine facilitated the growth of InOOH into 1D nanostructures in our experiment. In addition, InOOH easily grows into 1D nanostructures with 1D orthorhombic crystal growth habits.56,57
In2O3 nanorods could be obtained with the same rodlike shape upon annealing of the corresponding InOOH nanorods at 300 °C, which implies that the In2O3 nanorods are stable at this temperature. The crystalline structure and morphology of the corresponding In2O3 nanorods are given in Fig. 1b and 2c and d, respectively. In Fig. 1b, the XRD pattern of the In2O3 nanorods can be well matched with the standard XRD data file of In2O3 (JCPDS: 22-0336). It should be noted that the as-obtained In2O3 nanorods are highly crystalline, which can be confirmed from the clear crystalline fringes of the nanorods. Moreover, these lattice distances can be well matched with rhombohedral In2O3 (r-In2O3); the distance 3.97 Å corresponds to the lattice plane {012}, 2.833 Å to {104}, and 2.753 Å to {110}.
When aqueous ethanolamine was used as a solvent while the other conditions were kept constant, indium oxide nanocubes were produced. As shown in Fig. 3a, one finds that the as-synthesized nanocubes were composed of mixed phases of In2O3 (JCPDS: 22-0336) and InOOH (JCPDS: 17-0549). The coexistence of In2O3 and InOOH indicates that some of the rhombohedral In2O3 (r-In2O3) could originate from the as-formed InOOH. A low-magnification TEM image (Fig. 4a) shows that the sample is composed of many uniform nanocubes. In Fig. 4b, one clearly sees that the nanoparticles have a size of 5–8 nm.
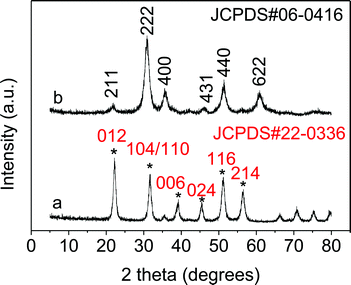 |
| Fig. 3 XRD patterns of the as-obtained nanocubes: (a) indium oxide, and (b) In2O3. | |
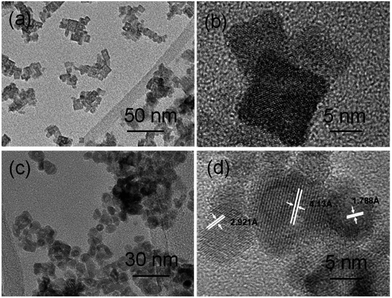 |
| Fig. 4 TEM images of the as-obtained indium oxide nanomaterials: (a and b) nanocubes, and (c and d) nanoparticles. | |
Interestingly, after annealing the mixture at 300 °C in air, pure cubic In2O3 (c-In2O3) was obtained, together with the InOOH and r-In2O3 being transformed to c-In2O3. The XRD pattern of the as-obtained In2O3 (Fig. 3b) could be well matched with the standard JCPDS card (06-0416). In addition, one finds that the regular nanocubes became spherical nanoparticles at higher temperature. Low and high magnification TEM images (Fig. 4c and d) clearly show that our In2O3 consists of uniform nanoparticles. The lattice distances (4.13 Å, 2.921 Å, 1.788 Å) can be matched to the crystal planes {211}, {222} and {440}, respectively.
Due to the smaller size of the In2O3 nanostructures, it is expected that the as-synthesized In2O3 nanostructures will show excellent gas sensing performance in gas sensors. The sensor sensitivity S, which is defined as S = Ra/Rg, where Ra is the resistance of the sensor in air and Rg is the resistance of the sensor in the target gas, is an important factor in gauging the ability of a sensor. Fig. 5 shows the sensitivity–temperature profiles of the two sensors of In2O3 nanorods and nanoparticles to 30 ppm of o-dichlorobenzene. The results indicate that the sensitivities of both the nanorods and nanoparticles are enhanced with an increasing temperature. Moreover, it is obvious that the In2O3 nanorod sensor has a higher sensitivity than the In2O3 nanoparticle sensor over the whole temperature range from 230 to 430 °C. The sensitivities for the In2O3 nanorod sensor to 30 ppm o-dichlorobenzene are 1.2 at 230 °C, 1.4 at 265 °C, 1.7 at 300 °C, 2.4 at 350 °C and 3.3 at 430 °C. Therefore, from the above analysis, in the following section the temperature of 430 °C was chosen for further sensing analysis.
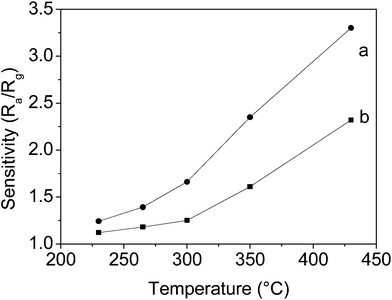 |
| Fig. 5 The sensitivity of the In2O3 sensors towards 30 ppm o-dichlorobenzene at different working temperatures: (a) nanorods, and (b) nanoparticles. | |
Fig. 6 shows the dynamic responses of the In2O3 nanorods and nanoparticles at an operating temperature of 430 °C for various concentrations of o-dichlorobenzene. In Fig. 6a and b, one can see that the response amplitude of the nanorod and nanoparticle sensors increases with increasing o-dichlorobenzene concentration. In Fig. 6c, the corresponding sensitivities of the In2O3 nanorod sensor to o-dichlorobenzene are found to be 2.2 for 10 ppm, 3 for 30 ppm, 5.6 for 50 ppm, 6.5 for 100 ppm, 7.7 for 200 ppm, 11.8 for 500 ppm and 18 for 1000 ppm, which are superior to those of the In2O3 nanoparticle sensor. The corresponding sensitivities of the In2O3 nanoparticle sensor are 1.6, 2.3, 2.9, 3.2, 3.5, 5 and 6.3, respectively. The difference in o-dichlorobenzene sensing performance between the r-In2O3 nanorods and c-In2O3 nanoparticles might be attributed to shape, size, or phase. o-Dichlorobenzene is a typical polar molecule, so its adsorption and desorption are a little sluggish on the In2O3 surface in our study.58,59
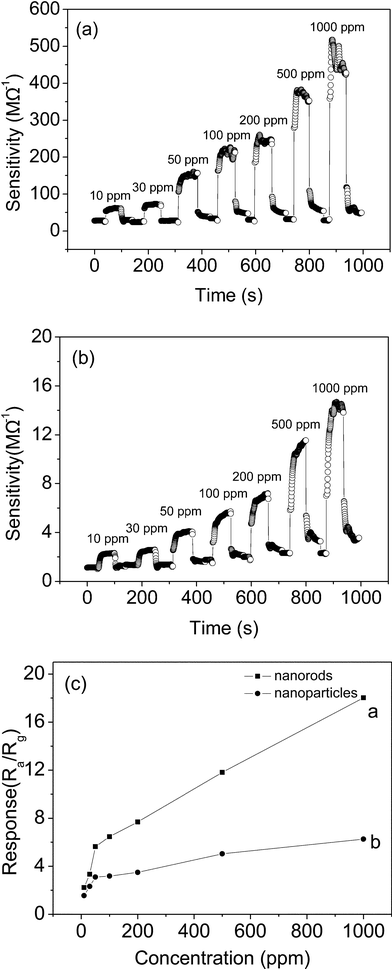 |
| Fig. 6 The real-time response curve of the In2O3 sensor to o-dichlorobenzene with increasing concentration at a working temperature of 430 °C, using nanorods (a) and nanoparticles (b) as sensing materials; (c) a comparison of sensor sensitivities to different o-dichlorobenzene gas concentrations for nanorods and nanoparticles, respectively. | |
In addition, we also investigated the corresponding sensitivities of the In2O3 nanorod and nanoparticle sensors to chlorobenzene. The response curve of the In2O3 sensor increased with an increase in chlorobenzene concentration, as shown in Fig. 7a and b. In Fig. 7c, the In2O3 nanorod sensor has a sensitivity of 2.8 for 10 ppm, 3.2 for 30 ppm, 5.4 for 50 ppm, 6.6 for 100 ppm, 10.5 for 200 ppm, 14.9 for 500 ppm and 20 for 1000 ppm, which are superior to those of the In2O3 nanoparticle sensor. The corresponding sensitivities for the In2O3 nanoparticle sensor are 2.4, 2.7, 4.3, 4.4, 4.8, 6.9 and 9. Obviously, the In2O3 sensor has better sensitivity to chlorobenzene than o-dichlorobezene.
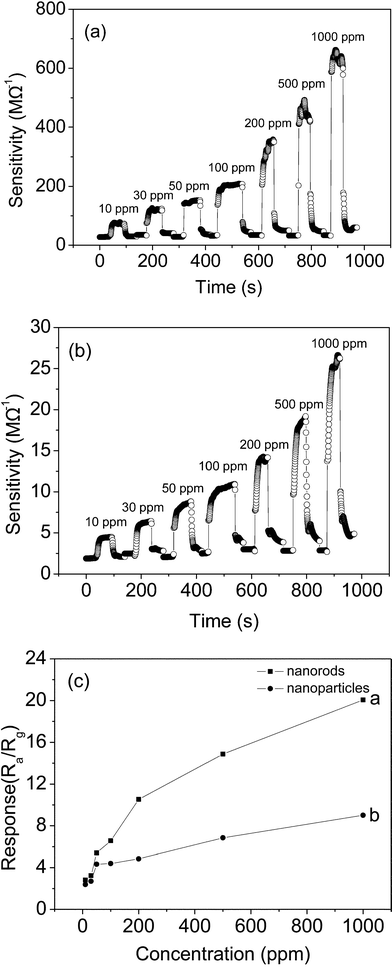 |
| Fig. 7 The real-time response curve of the In2O3 sensor to chlorobenzene with increasing concentration at a working temperature of 430 °C, using nanorods (a) and nanoparticles (b) as sensing materials; (c) a comparison of sensor sensitivities to different chlorobenzene concentrations for nanorods and nanoparticles, respectively. | |
The mechanism of In2O3 sensitivity to o-dichlorobenzene and chlorobenzene is further explained. According to the classical electron depletion theory, the change of resistance is dependent on species of chemisorbed oxygen (O2−, O2− and O−) on the surface,6 which would cause electron depletion, and consequently increase the resistance of the sensor. When chlorobenzene or o-dichlorobenzene gases are present at a certain temperature, they would be oxidized by these chemisorbed oxygen species on the surface of the sensor. This will then lead to an increase in the number of electrons in the conduction band, and an increase in sensor conductivity. The equations involved in the response process are as follows:
|
C6H4Cl2 → C6H4Cl2+ + e−
| (d) |
Moreover, owing to higher reaction activity, the In2O3 sensor has a higher sensitivity to chlorobenzene. These results also further confirm that our proposed mechanism is reasonable. The electron transfer on the In2O3 sensor surface is clearly shown in Scheme 1.
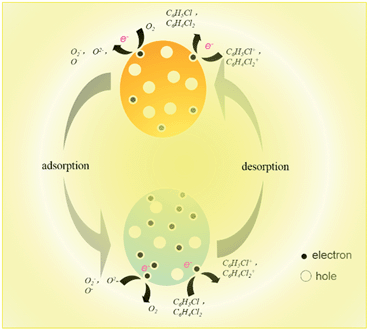 |
| Scheme 1 The electron transfer process on the In2O3 sensor surface. | |
Conclusions
In summary, a new solvothermal route has been developed to synthesize ultrathin InOOH nanorods, which could be further converted into In2O3 nanorods through calcination at a high temperature. Furthermore, it was found that the gas sensing performance of the In2O3 nanorods for o-dichlorobenzene could be improved in comparison to nanoparticles, as a result of their high sensitivity and rapid response to o-dichlorobenzene due to the advantages of the effective adsorption and rapid diffusion of the o-dichlorobenzene molecules. The as-synthesized ultrathin In2O3 nanorods could be applied to the on-line monitoring of dioxins in the future.
Acknowledgements
This work was supported by the National Natural Science Foundation of China (Grant no. 21103046, 21373081, 51302079 and 61376073) and the Young Teachers' Growth Plan of Hunan University (Grant no. 2012-118).
Notes and references
- N. Yamazoe, Sens. Actuators, B, 1991, 5, 7–19 CrossRef CAS.
- N. Liu, M. L. Tang, M. Hentschel, H. Giessen and A. P. Alivisatos, Nat. Mater., 2011, 10, 631–636 CrossRef CAS PubMed.
- W. Guo, J. M. Ma, G. S. Pang, C. Y. Wei and W. J. Zheng, J. Mater. Chem. A, 2014, 2, 1032–1038 CAS.
- M. Bao, Y. J. Chen, F. Li, J. M. Ma, T. Lv, Y. J. Tang, L. B. Chen, Z. Xu and T. H. Wang, Nanoscale, 2014, 6, 4063–4066 RSC.
- J. W. Deng, J. M. Ma, L. Mei, Y. J. Tang, Y. J. Chen, T. Lv, Z. Xu and T. H. Wang, J. Mater. Chem. A, 2013, 1, 12400–12403 CAS.
- J. M. Ma, L. Mei, Y. J. Chen, Q. H. Li, T. H. Wang, Z. Xu, X. C. Duan and W. J. Zheng, Nanoscale, 2013, 5, 895–898 RSC.
- X. H. Liu, J. Zhang, S. H. Wu, D. J. Yang, P. R. Liu, H. M. Zhang, S. R. Wang, X. D. Yao, G. S. Zhu and H. J. Zhao, RSC Adv., 2012, 2, 6178–6184 RSC.
- J. M. Ma, J. Teo, L. Mei, Z. Y. Zhong, Q. H. Li, T. H. Wang, X. H. Duan, J. B. Lian and W. J. Zheng, J. Mater. Chem., 2012, 22, 11694–11700 RSC.
- B. Miao, W. Zeng, L. Y. Lin and S. Xu, Physica E, 2013, 52, 40–45 CrossRef CAS PubMed.
- F. Li, Y. J. Chen and J. M. Ma, RSC Adv., 2014, 4, 14201–14205 RSC.
- H. Steinebach, S. Kannan, L. Rieth and F. Solzbacher, Sens. Actuators, B, 2010, 151, 162–168 CrossRef CAS PubMed.
- J. C. Fu, C. H. Zhao, J. L. Zhang, Y. Peng and E. Q. Xie, ACS Appl. Mater. Interfaces, 2013, 5, 7410–7416 CAS.
- J. M. Ma, J. Zhang, S. R. Wang, Q. H. Wang, L. F. Jiao, J. Q. Yang, X. C. Duan, Z. F. Liu, J. B. Lian and W. J. Zheng, CrystEngComm, 2011, 13, 6077–6081 RSC.
- F. Li, Y. J. Chen and J. M. Ma, J. Mater. Chem. A, 2014, 2, 7175–7178 CAS.
- Y. L. Wang, X. C. Jiang and Y. N. Xia, J. Am. Chem. Soc., 2003, 125, 16176–16177 CrossRef CAS PubMed.
- J. Zhang, S. R. Wang, Y. M. Wang, Y. Wang, B. L. Zhu, H. J. Xia, X. Z. Guo, S. M. Zhang, W. P. Huang and S. H. Wu, Sens. Actuators, B, 2009, 135, 610–617 CrossRef CAS PubMed.
- A. Aslani and V. Oroojpour, Physica B, 2011, 406, 144–149 CrossRef CAS PubMed.
- M. Mashock, K. H. Yu, S. M. Cui, S. Mao, G. H. Lu and J. H. Chen, ACS Appl. Mater. Interfaces, 2012, 4, 4192–4199 CAS.
- D. P. Volanti, A. A. Felix, M. O. Orlandi, G. Whitfield, D. J. Yang, E. Longo, H. L. Tuller and J. A. Varela, Adv. Funct. Mater., 2013, 23, 1759–1766 CrossRef CAS.
- J. Nisar, Z. Topalian, A. De Sarkar, L. Österlund and R. Ahuja, ACS Appl. Mater. Interfaces, 2013, 5, 8516–8522 CAS.
- C. X. Wang, L. W. Yin, L. Y. Zhang, Y. X. Qi, N. Lun and N. N. Liu, Langmuir, 2010, 26, 12841–12848 CrossRef CAS PubMed.
- C. C. Li, X. M. Yin, T. H. Wang and H. C. Zeng, Chem. Mater., 2009, 21, 4984–4992 CrossRef CAS.
- A. M. Cao, J. S. Hu, H. P. Liang, W. G. Song, L. J. Wan, X. L. He, X. G. Gao and S. H. Xia, J. Phys. Chem. B, 2006, 110, 15858–15863 CrossRef CAS PubMed.
- J. M. Ma, J. Zhang, S. R. Wang, T. H. Wang, J. B. Lian, X. C. Duan and W. J. Zheng, J. Phys. Chem. C, 2011, 115, 18157–18163 CAS.
- Z. H. Li, J. C. Li, L. L. Song, H. Q. Gong and Q. Niu, J. Mater. Chem. A, 2013, 1, 15377–15382 CAS.
- J. Yu, H. Wen, M. Shafiei, M. R. Field, Z. F. Liu, W. Wlodarski, N. Motta, Y. X. Li, K. Kalantar-zadeh and P. T. Lai, Sens. Actuators, B, 2013, 184, 118–129 CrossRef CAS PubMed.
- C. Cantalini, W. Wlodarski, H. T. Sun, M. Z. Atashbar, M. Passacantando and S. Santucci, Sens. Actuators, B, 2000, 65, 101–104 CrossRef CAS.
- T. Waitz, T. Wagner, T. Sauerwald, C. D. Kohl and M. Tiemann, Adv. Funct. Mater., 2009, 19, 653–661 CrossRef CAS.
- P. S. Khiabani, A. Hosseinmardi, E. Marzbanrad, S. Ghashghaie, C. Zamani, M. Keyanpour-Rad and B. Raissi, Sens. Actuators, B, 2012, 162, 463–470 Search PubMed.
- C. S. Lee, I. D. Kim and J. H. Lee, Sens. Actuators, B, 2013, 181, 102–107 CrossRef PubMed.
- S. Park, S. An, H. Ko, C. Jin and C. Lee, Bull. Korean Chem. Soc., 2012, 33, 3368–3372 CrossRef CAS.
- H. Y. Du, J. Wang, M. Y. Su, P. J. Yao, Y. G. Zheng and N. S. Yu, Sens. Actuators, B, 2012, 166, 746–752 CrossRef PubMed.
- H. Y. Lai and C. H. Chen, J. Mater. Chem., 2012, 22, 13204–13208 RSC.
- J. Q. Xu, Y. P. Chen, Q. Y. Pan, Q. Xiang, Z. X. Cheng and X. W. Dong, Nanotechnology, 2007, 18, 115615 CrossRef.
- X. Y. Lai, D. Wang, N. Han, J. Du, J. Li, C. J. Xing, Y. F. Chen and X. T. Li, Chem. Mater., 2010, 22, 3033–3042 CrossRef CAS.
- L. Xu, B. A. Dong, Y. Wang, X. Bai, Q. Liu and H. W. Song, Sens. Actuators, B, 2010, 147, 531–538 CrossRef CAS PubMed.
- P. C. Xu, Z. X. Cheng, Q. Y. Pan, J. Q. Xu, Q. Xiang, W. J. Yu and Y. L. Chu, Sens. Actuators, B, 2008, 130, 802–808 CrossRef CAS PubMed.
- A. Vomiero, S. Bianchi, E. Comini, G. Faglia, M. Ferroni and G. Sberveglieri, Cryst. Growth Des., 2007, 7, 2500–2504 CAS.
- T. Zhang, F. B. Gu, D. M. Han, Z. H. Wang and G. S. Guo, Sens. Actuators, B, 2013, 177, 1180–1188 CrossRef CAS PubMed.
- S. J. Kim, I. S. Hwang, J. K. Choi, Y. C. Kang and J. H. Lee, Sens. Actuators, B, 2011, 155, 512–518 CrossRef CAS PubMed.
- B. X. Li, Y. Xie, M. Jing, G. X. Rong, Y. C. Tang and G. Z. Zhang, Langmuir, 2006, 22, 9380–9385 CrossRef CAS PubMed.
- Z. P. Li, H. Yan, S. L. Yuan, Y. J. Fan and J. H. Zhan, J. Colloid Interface Sci., 2011, 354, 89–93 CrossRef CAS PubMed.
- K. W. Park, S. B. Kang, J. A. Jeong, S. W. Choi, J. Kim, I. K. You, Y. S. Yang and H. K. Kim, J. Phys. D: Appl. Phys., 2013, 46, 145301 CrossRef.
- I. A. Tambasov, V. G. Myagkov, A. A. Ivanenko, I. V. Nemtsev, L. E. Bykova, G. N. Bondarenko, J. L. Mihlin, I. A. Maksimov, V. V. Ivanov, S. V. Balashov and D. S. Karpenko, Semiconductors, 2013, 47, 569–573 CrossRef CAS.
- B. P. Bastakoti, H. Oveisi, C. C. Hu, K. C. W. Wu, N. Suzuki, K. Takai, Y. Kamachi, M. Imura and Y. Yamauchi, Eur. J. Inorg. Chem., 2013, 1109–1112 CrossRef CAS.
- M. Osiak, W. Khunsin, E. Armstrong, T. Kennedy, C. M. S. Torres, K. M. Ryan and C. O'Dwyer, Nanotechnology, 2013, 24, 065401 CrossRef CAS PubMed.
- G. Z. Shen, B. Liang, X. F. Wang, H. T. Huang, D. Chen and Z. L. Wang, ACS Nano, 2011, 5, 6148–6155 CrossRef CAS PubMed.
- Y. G. Yan, Y. Zhang, H. B. Zeng, J. X. Zhang, X. L. Cao and L. D. Zhang, Nanotechnology, 2007, 18, 175601 CrossRef.
- Y. F. Hao, G. W. Meng, C. H. Ye and L. D. Zhang, Cryst. Growth Des., 2005, 5, 1617–1621 CAS.
- W. Y. Yin, M. H. Cao, S. J. Luo, C. W. Hu and B. Q. Wei, Cryst. Growth Des., 2009, 9, 2173–2178 CAS.
- W. C. Chang, C. H. Kuo, P. J. Lee, Y. L. Chueh and S. J. Lin, Phys. Chem. Chem. Phys., 2012, 14, 13041–13045 RSC.
- M. Hafeez, T. Y. Zhai, A. S. Bhatti, Y. Bando and D. Golberg, Cryst. Growth Des., 2012, 12, 4935–4943 CAS.
- W. H. Zhang, F. Wang and W. D. Zhang, Dalton Trans., 2013, 42, 4361–4364 RSC.
- C. J. Chen, W. L. Xu and M. Y. Chern, Adv. Mater., 2007, 19, 3012–3015 CrossRef CAS.
- Z. H. Jing and J. H. Zhan, Adv. Mater., 2008, 20, 4547–4551 CrossRef CAS.
- L. Y. Chen, Y. Liang and Z. D. Zhang, Eur. J. Inorg. Chem., 2009, 903–909 CrossRef CAS.
- X. X. Xu and X. Wang, Inorg. Chem., 2009, 48, 3890–3895 CrossRef CAS PubMed.
- X. M. Xu, P. L. Zhao, D. W. Wang, P. Sun, L. You, Y. F. Sun, X. S. Liang, F. G. Liu, H. Chen and G. Y. Lu, Sens. Actuators, B, 2013, 176, 405–412 CrossRef CAS PubMed.
- P. S. Khiabani, A. Hosseinmardi, E. Marzbanrad, S. Ghashghaie, C. Zamani, M. Keyanpour-Rad and B. Raissi, Sens. Actuators, B, 2012, 162, 102–107 CrossRef PubMed.
|
This journal is © The Royal Society of Chemistry 2014 |
Click here to see how this site uses Cookies. View our privacy policy here.