DOI:
10.1039/C3RA47861K
(Paper)
RSC Adv., 2014,
4, 17768-17779
Pseudo-graft polymer based on adamantyl-terminated poly(oligo(ethylene glycol) methacrylate) and homopolymer with cyclodextrin as pendant: its thermoresponsivity through polymeric self-assembly and host–guest inclusion complexation
Received
21st December 2013
, Accepted 14th March 2014
First published on 14th March 2014
Abstract
A series of well-defined adamantyl-terminated thermally responsive copolymers (Ad-POEGMAs) were synthesized by atom transfer radical polymerization (ATRP), in which 2-(2-methoxyethoxy) ethyl methacrylate (MEO2MA) and oligo(ethylene glycol) methacrylate (OEGMA) served as the thermosensitive building blocks. Moreover, cyclodextrins (CDs) as bulky pendant grafted polymers (PGCD) were synthesized by homopolymerization of aminoethyl methacrylate β-cyclodextrin (GCD). The thermal-responsive behaviors were investigated by a combination of 1H NMR, UV-vis spectroscopy, dynamic light scattering (DLS), and transmission electron microscopy (TEM). In comparison to other thermal-responsive copolymers based on POEGMAs, Ad-POEGMAs exhibited unusual thermally induced aggregation processes. The Ad group assembled and POEGMA chains associated to produce stable water-soluble nano-aggregates, followed by a rearrangement process at the second thermal transition. Moreover, it was found that a noncovalently connected supramolecular pseudo-graft polymer was formed via inclusion complexation in aqueous solution. This pseudo-graft polymer underwent a reversible temperature-induced transition from solution to micelle under suitable conditions. The cyclodextrin (CD) moiety attached to the main chain played two roles. As supramolecular host moieties, CDs formed inclusion complexes with guest-ended polymers, leading to graft-like polymers. As bulky hydrophilic moieties, CDs stabilized the micelles induced by the coil-to-globule transition of POEGMA segments.
Introduction
Polymers that respond with a property alteration towards environmental changes are often referred to as “smart” polymeric systems because they exhibit reversible property changes in response to changes in external conditions such as pH, temperature, ionic strength, light irradiation, mechanical force, electric and magnetic fields, and analytes of interest (e.g., ions and bioactive molecules) or an integration of these factors.1–7 For cases in which the external trigger is temperature, polymers are believed to exhibit thermoresponsive properties. Poly(N-isopropylacrylamide) (PNIPAM), one of the most popular thermally responsive polymers, possesses dramatic and reversible phase transition behavior in aqueous solutions and a lower critical solution temperature (LCST).8–11 Progress in the design and application of PNIPAM-based thermoresponsive polymers during the past decades has been covered in a range of review articles.12,13 Recently, a series of methacrylate-based polyethylene glycol(PEG) polymers (POEGMAs) substituted with oligo(ethylene glycol) (OEG) units as side chains were reported to possess a thermosensitivity similar to or even superior than PNIPAMs, and these POEGMAs have attracted a great deal of attention.14–20 POEGMAs have good phase transition reversibilities and tunable lower critical solution temperatures (LCSTs) in aqueous media and have excellent biocompatibility. Moreover, by the copolymerization of various OEGMA monomers with different side chain lengths and by varying the feed molar ratio of the comonomers, the LCSTs of POEGMAs can be conveniently adjusted. To date, substantial attention has been given for the design of POEGMA-based polymers, which have been widely incorporated into dendrimers, microgels, block or comb-like copolymers, polymeric brushes and gold surfaces which provide these materials with fascinating properties. Furthermore, although studies have indicated that the thermal-responsive mechanism of POEGMAs can also be regarded as a consequence of the competition between hydrophilic polymer–water interactions and hydrophobic polymer–polymer interactions, the role of POEGMAs in a thermally induced self-assembly process has not been sufficiently studied, especially when POEGMAs serve as thermosensitive components for composite systems that include various components.21–23
Compared with traditional chemistry based on covalent bonds, supramolecular chemistry based on non-covalent bonds has become a powerful method of constructing supramolecular systems in a facile and dynamic manner. A variety of subtle non-covalent interactions such as hydrogen bonding, metal–ligand coordination interaction, electrostatic attraction, and host–guest inclusion complexation have been widely used as driving forces for constructing supramolecular systems.24–34 Therefore, on the basis of these results, well-defined Ad-POEGMAs are synthesized in this study for the first time and are used to fine-tune the LCSTs of PEG-based thermoresponsive polymers. Moreover, this work focuses on the self-assembly behavior of a composite system between Ad-POEGMAs as guests consisting of POEGMAs as thermal blocks and PGCD as multi-host with pendant CD cavities via inclusion complexation. The design of this special composite system is based on the following facts and considerations: the main parts of Ad-POEGMAs are thermal-sensitive POEGMA segments; thus, thermally induced self-assembly is possible. Moreover, the CD species is a bulky group that provides the PGCD with some characteristics of a comb-like polymer. Further, the hydrophobic interior cavity of β-CD can accommodate a variety of guest molecules. Among them, the β-CD–Ad pair is well-known due to its high association constant; thus, PGCD may show some peculiar characteristics in assembly. Finally, the CD cavities as hosts are available for post-polymerization modifications by POEGMA with Ad guest ends via inclusion complexation to construct a complex supramolecular system. In the current work, this supramolecular system is formed with mixtures of Ad-POEGMAs and PGCD due to the inclusion complexation between terminal CD and Ad moieties. Because it possesses thermoresponsive POEGMAs, the obtained supramolecular pseudo-graft polymer is expected to exhibit intriguing aggregation properties in aqueous solutions.
Results and discussion
Synthesis and structure analysis of β-CD based monomers and PGCD homopolymer
Herein, we systematically report an efficient and preparatively simple approach for the generation of β-CD-based monomers with high purity and excellent yield, which all were confirmed by element analysis and IR, 1H NMR, 13C NMR, and MALDI-TOF measurements. The detailed synthesis processes of β-CD based monomers are shown in our previous reports.35 The synthetic route of TCD, ECD, and GCD are shown in Scheme 1, and the 1H NMR spectra of TCD, ECD, and GCD are shown in Fig. 1. Water-soluble PGCD was prepared by homopolymerization, and its SEC polydispersity index (Mw/Mn) was low at 1.19.
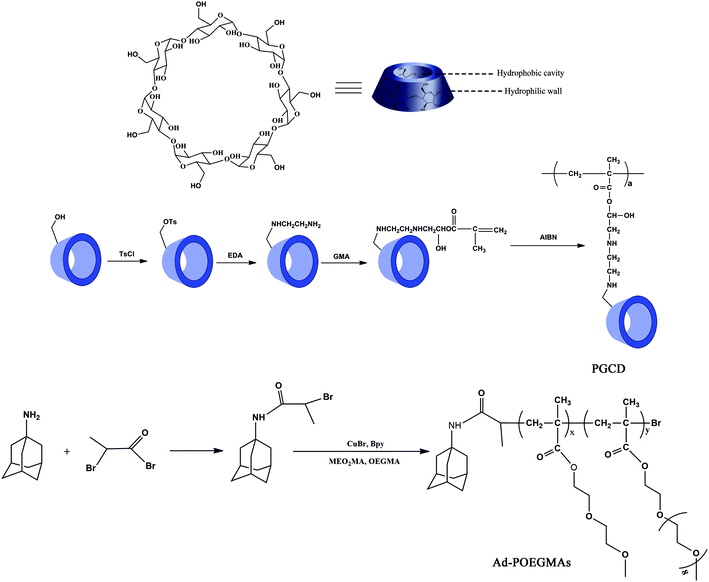 |
| Scheme 1 Synthetic routes employed for the preparation of CD based monomers and PGCD, and ATRP initiator AdBB and Ad-POEGMAs. | |
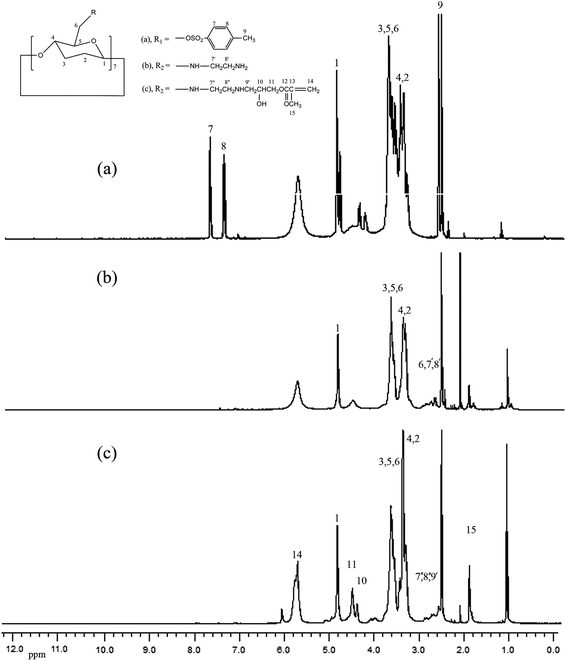 |
| Fig. 1 1H NMR spectra of β-CD based monomers (TCD and ECD) and GCD. | |
Synthesis and structure analysis of AdBB and Ad-terminated P(MEO2MA-co-OEGMA) copolymers (Ad-POEGMAs)
The AdBB initiator was synthesized by 2-bromopropiomyl bromide and 1-adamantanamine with high purity and yield. Using AdBB as the initiator and MEO2MA and OEGMA as comonomers, Ad-POEGMAs were synthesized by atom transfer radical polymerization (ATRP). As reported by Lutz et al., increasing the feed molar ratio of MEO2MA to OEGMA leads to a decrease in the LCST of POEGMAs. Therefore, three Ad-POEGMAs named A1, A2, and A3 with molar ratios of MEO2MA to OEGMA of 95
:
5, 90
:
10, and 85
:
15, respectively, were used in this study. The polymerization conditions and results are summarized in Table 1. Fig. 2 shows the 1H NMR spectra of AdBB and A1. In addition to the resonance peaks of the POEGMAs segment in the range of δ = 0.8–4.1 ppm, the characteristic signals of the Ad segment, were also observed at δ = 1.6–2.1 ppm, indicating that Ad-POEGMAs were successfully synthesized, although the signals of Ad moiety were partly overlapped by the relative signals of the main-chain methylene protons of the main chains at δ = 1.3–2.4 ppm.
Table 1 Summary of structural parameters of PGCD and Ad-terminated POEGMA copolymers (A1, A2 and A3)
Samples |
Initiator |
nMEO2MA : nOEGMAc |
Mn,SECd |
Mw,SECd |
PDId |
Synthesized via the homopolymerization of aminoethyl methacrylate β-cyclodextrin. Synthesized by the ATRP of MEO2MA and OEGMA using AdBB as initiator. Mole ratio (%) of MEO2MA and OEGMA in feed. Molecular weights and molecular distributions (Mw/Mn, PDI) were determined by SEC using THF as the eluent relative to polystyrene standards. |
PGCDa |
— |
— |
11 950 |
14 220 |
1.19 |
A1b |
AdBB |
95 : 5 |
23 163 |
30 427 |
1.32 |
A2b |
AdBB |
90 : 10 |
29 610 |
39 604 |
1.35 |
A3b |
AdBB |
85 : 15 |
34 667 |
46 535 |
1.34 |
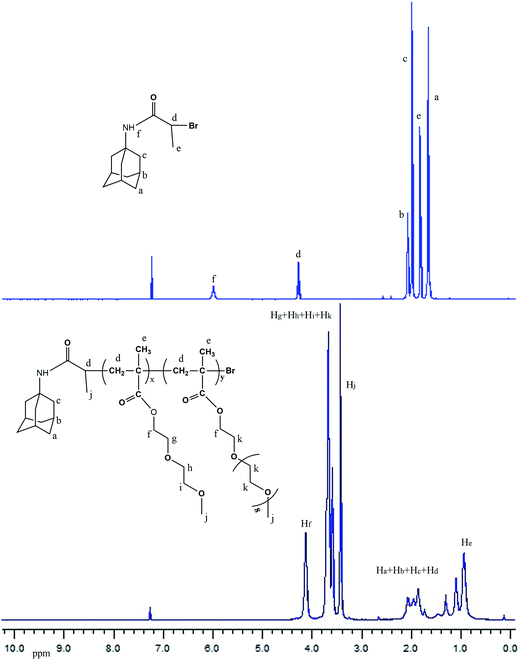 |
| Fig. 2 1H NMR spectra of AdBB and a Ad-terminated P(MEO2MA-co-OEGMA) copolymer (A1) containing 5 mol% of OEGMA per chain recorded in CDCl3. | |
Fig. 3 presents the 2D 1H NOESY spectrum of the mixture of Ad-POEGMA (A1) guest and PGCD host in DMSO-d6, which provides direct evidence for the formation of the pseudo-graft PGCD–Ad-POEGMA polymer. The NOESY cross-peaks between the signals at 3.0–4.0 ppm ascribed to the inner protons C(3)H and C(5)H of β-CD segments and the signals at 1.6–2.1 ppm assigned to the methine protons (Hb) and the methylene protons (Ha and Hc) of adamantyl moieties were clearly observed, which indicates that Ad moieties were included in the cavity of β-CD. Therefore, the Ad-POEGMAs were successfully synthesized by atom transfer radical polymerization.
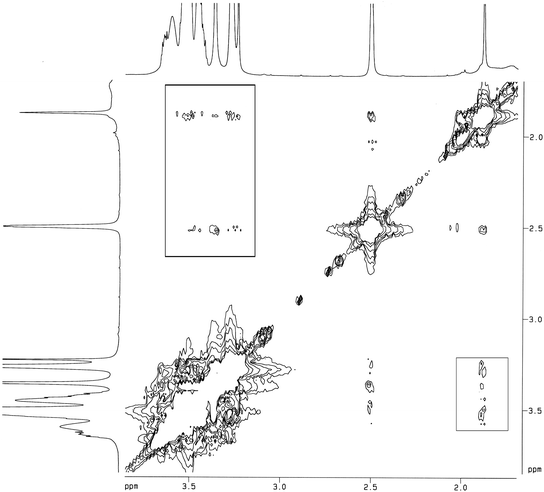 |
| Fig. 3 2D 1H NOESY spectrum of the host–guest mixture of PGCD host and Ad-POEGMA guest in DMSO-d6 at 25 °C. | |
Thermoresponsive behavior of the Ad-POEGMAs and host–guest inclusion complexation system
Polyethylene glycol (PEG) and its derivatives are well known for their highly hydrophilic, or water-soluble, nature and exhibit LCSTs in aqueous solutions.14–19 They are water-soluble below their LCSTs and water-insoluble above. The mechanism proposed for explaining the thermoresponsiveness of POEGMAs is summarized as follows: the conformationally favored formation of H-bonds between the ether oxygens of PEG and water hydrogens is one of the key factors responsible for the unusual water solubility of PEG-type polymers.21,22 Thus, Ad-POEGMAs are expected to self-assemble into nano-sized aggregates or micelles in their aqueous solutions by altering their temperatures. Moreover, the interesting thermoresponsive behavior of the host–guest system comprising a polymeric host PGCD with CD as pendant and a polymeric guest Ad segment with POEGMAs in an aqueous solution was investigated. In this study, the thermally induced self-assembly behaviors were investigated in detail by a combination of UV-vis, DLS, TEM, and 1H NMR in D2O.36
(a) Determination of the LCSTs of Ad-POEGMAs. UV-vis was first used to determine the LCSTs of the Ad-POEGMAs. The results are shown in Fig. 4. A gradual decline in solution transmittance could be observed with elevated temperature, indicating that the three Ad-POEGMAs were all thermally responsive. The LCSTs for A1, A2, and A3 were 37.94 °C, 42.07 °C, and 46.71 °C, respectively, and they increased with an increase in OEGMA content. Previous studies have indicated that the LCSTs of the copolymers of 2-(2-methoxyethoxy) ethyl methacrylate (MEO2MA) and ω-methoxy (oligoethyleneoxy) ethyl methacrylate (OEGMA) also increased significantly with an increase in OEGMA content. In this study, in contrast to that in previous reports, the Ad group was firstly introduced to the POEGMA system, although similar results were further confirmed for different POEGMA copolymers with hydrophobic Ad groups. However, the difference for Ad-POEGMAs is that the LCSTs are higher than those in the POEGMA copolymers without Ad groups.
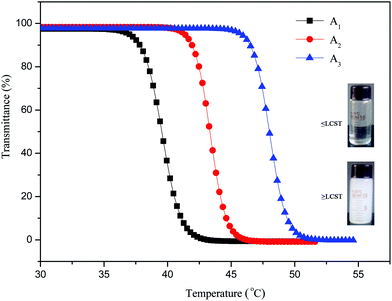 |
| Fig. 4 Plots of transmittance as a function of temperature measured for aqueous solutions (3 mg mL−1) of Ad-POEGMAs (A1, A2 and A3). | |
(b) Determination of the LCSTs of the supramolecular system of Ad-POEGMAs and PGCD. In this study, the interesting thermoresponsive behavior was further investigated for the host–guest system comprising polymeric guest Ad-POEGMAs and host PGCD in an aqueous solution. Both the host and the guest polymers used in this study were all soluble at room temperature. Fig. 5 shows information on the thermoresponsive behaviors of a series of mixtures of PGCD and Ad-POEGMAs with varied host–guest ratios. The individual aqueous pure guest exhibited a thermoresponsive profile, although the host polymer exhibited no thermoresponsive profile. When the two components were mixed, the LCSTs of the host–guest supramolecular systems decreased, and when the added amount of polymeric host PGCD increased to some extent, the LCST became almost saturated when the composite system exhibited a 1
:
1 complexation between adamantyl moiety and β-CD core. The detailed experimental results for the aqueous mixtures of A1 and PGCD are given below as a typical example.
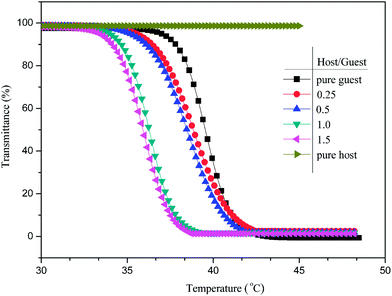 |
| Fig. 5 Turbidity variations of aqueous solutions of Ad-POEGMAs (A1) (guest) as a function of temperature upon the addition of PGCD (host). cguest = 3 mg mL−1 (pure guest); nPGCD–Ad-POEGMA = 0.25, 0.5, 1, 1.5 separately. | |
Fig. 5 shows that the LCST of the pure guest (A1) was 37.94 °C, which is somewhat higher than body temperature and promising for biomedical application, and shifted to a lower temperature upon the addition of the host (PGCD). The LCST significantly changed with LCST decreases (ΔLCST, referenced to pure guest) of 1.45 °C, 1.74 °C, 3.13 °C, and 3.65 °C with the host–guest ratio of 0.25, 0.5, 1.0, and 1.5, respectively. Finally, the LCST change with further addition of host became imperceptible, indicating that the composite system involved complexation equilibrium between the adamantyl moiety (Ad) and β-CD core. The Ad-terminated POEGMAs could be incorporated to the β-CD core via inclusion complexation to form a pseudo-graft supramolecular polymer. Through formation of this polymer, such networks stabilized not only by single CD–Ad complexes but also by pairs of CD–Ad complexes, and the hydrophilic POEGMA arms on the guest polymer contributed to an increase in water solubility of the supramolecular polymeric system. However, when the added amount of PGCD exceeded the requirement of the complexation equilibrium, the excess PGCD reduced the thermal sensitivity of this supramolecular system.
Size and morphology of the Ad-POEGMAs and host–guest inclusion complexation system
(a) Size and morphology of the assemblies formed by Ad-POEGMAs. Although researchers have demonstrated that comb-like polymers with multiple PEG side chains generally adopt a compact coil conformation in water, the existence of intermolecular associations resulting in the formation of larger aggregates such as polymer vesicles has also been suggested.37,38 Moreover, previous reports have indicated the existence of a hydrophilic propionate-terminated poly(MEO2MA–OEGMA) solution that had a coexistence of small particles with a hydrodynamic radius (Rh) less than 10 nm.21 Thus, in the present case, it is important to study the exact conformation of Ad-POEGMAs in aqueous solutions above and below the LCSTs. Fig. 6 shows the typical size distributions measured by dynamic light scattering (DLS) for an aqueous solution (3 mg mL−1) of the Ad-POEGMAs (A1) containing 5 mol% of OEGMA. This solution was optically clear at room temperature; however, the DLS intensity distribution indicated the coexistence of small particles with a hydrodynamic radius (Rh) of approximately 10–100 nm and larger aggregates with a radius of roughly 350 nm; the latter were indeed a negligible minority, as evidenced by the number distribution shown. Hence, the size distributions of the Ad-POEGMAs in aqueous solutions presented a wide poly-dispersity with a large hydrodynamic radius below the LCSTs, indicating that Ad-POEGMAs not only exist as a random coil conformation in aqueous solutions but also with pseudo aggregates. However, under lower temperature (<LCSTs), the Ad-POEGMAs were still easily soluble.
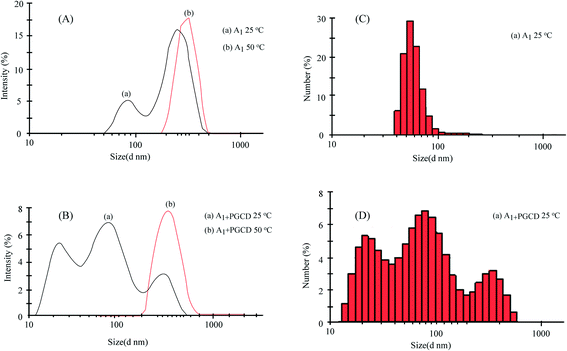 |
| Fig. 6 Size distributions of the aggregates formed by A1 (A and C), and A1 + PGCD (B and D) in aqueous solution (3 mg mL−1) at 25 and 50 °C, separately. | |
According to previous reports, the self-assembly mechanism of random copolymers of P(MEO2MA-co-OEGMA) includes four consecutive conformation changes of hydrated chains-dehydrated chains-loosely aggregated micelles-densely aggregated micelles during the self-aggregation process.39,40 Therefore, a reasonable explanation for Ad-POEGMAs can be summed as follows: as shown in Scheme 2, the special hydrophobic Ad groups connected to POEGMAs covered in PEG chains or those self-assembled partly resulted in different aggregates sizes in their aqueous solutions. A plausible structure of aggregates should be that with hydrophobic adamantyl (Ad) chains towards the interior, whereas hydrophilic POEGMA chains were exposed and stretched in water by the hydrophilic–hydrophilic interactions and therefore behaved as water soluble. As the temperature increased, the partial dehydration of PEG side chains collapsed first to get close to the hydrophobic backbones or Ad group, then distorted to expose hydrophilic ether oxygen groups to the outer shell of the polymer chains as much as possible. When the temperature rose above the LCSTs, the Ad-POEGMAs self-assembled into nano-sized aggregates in their aqueous solutions. The uniform aggregates formed with values ranging from 150 nm to 250 nm with a Z-average diameter (DZ = 181 nm, PDI = 0.116), revealing the occurrence of thermally induced self-assembly of the Ad-POEGMAs. However, the sizes of this nano-sized aggregates of Ad-POEGMAs were obvious larger than those in previous reports on P(MEO2MA-co-OEGMA) copolymers resulting from the Ad group.
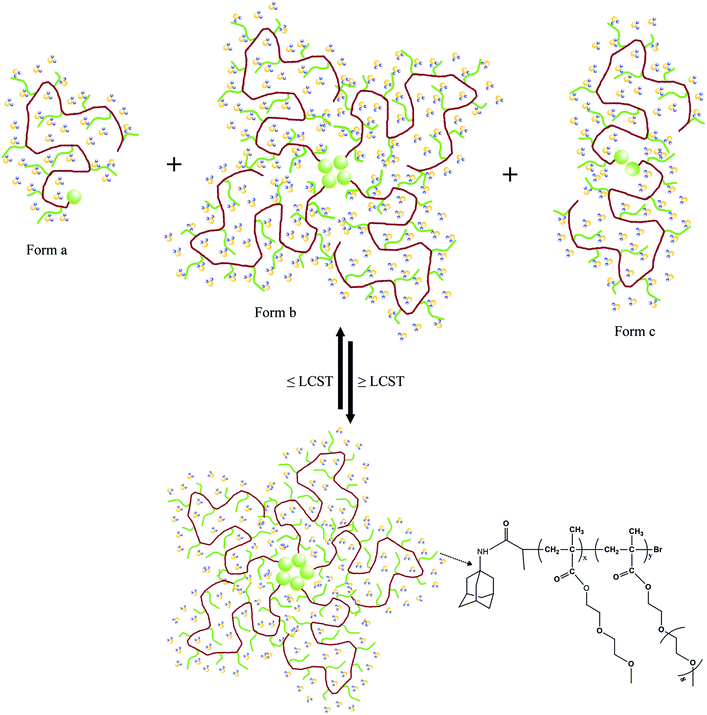 |
| Scheme 2 Schematic illustration of the dynamic self-aggregation and self-disaggregation mechanism of Ad-POEGMAs in water during heating and cooling. | |
To further confirm the self-assembly mechanism, Ad-POEGMAs (A1) were investigated by 1H NMR in various deuterated solvents. Fig. 7 compares the spectra measured for the Ad-POEGMAs (A1) in deuterated water (D2O) at 25 °C and 50 °C. As shown in Fig. 2, in deuterated chloroform, all of the protons of A1 led to sharp and intense signals, which suggests that the macromolecule was uniformly molecularly dissolved. Rather different results were observed in D2O. At 25 °C and 50 °C, the peaks corresponding to the protons of the oligo (ethylene glycol) side chains remained sharp; however, the signals of the protons belonging to the Ad group and backbone or those located in close proximity to the backbone δ = 1.6–2.1 ppm reduced considerably and broadened more than those observed in CDCl3. In D2O at 50 °C, the signals of the protons belonging to the Ad group disappeared compared to those observed in D2O at 25 °C. On the basis of the above analysis, it can be concluded that the nano-assemblies formed by the Ad-POEGMAs were stabilized by Ad segment aggregation and POEGMA chains dehydrated and aggregated jointly through hydrophobic–hydrophobic interactions.
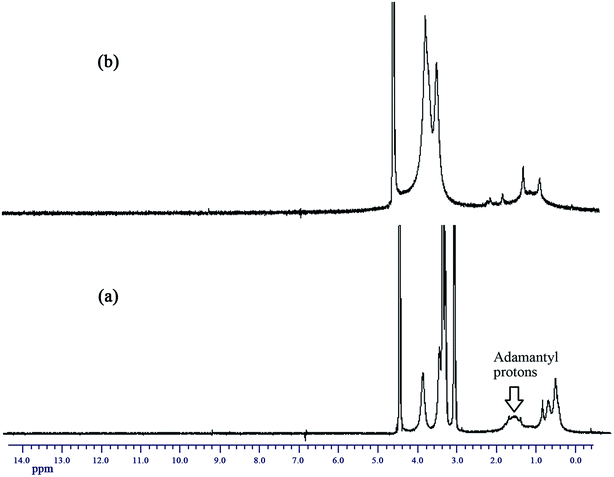 |
| Fig. 7 1H NMR spectra of a copolymer P(MEO2MA-co-OEGMA) containing 5 mol% of OEGMA per chain recorded in D2O at 25 and 50 °C. | |
(b) Size and morphology of the assemblies formed by host–guest inclusion complexation system. DLS has proven to be a sensitive method for tracing the formation of noncovalently connected nano-assemblies according to the changes in particle size and scattered light intensity.41,42 The changes in micelle size with temperature (25 °C and 50 °C) for the 1
:
1 host–guest system of Ad-POEGMAs (A1) and PGCD are shown in Fig. 6. The Rh of the nano-assemblies were approximately 10–300 nm, and they were kept unevenly under low temperature (<LCST). However, the values increased dramatically to 200–350 nm with a Z-average diameter (DZ = 273 nm, PDI = 0.121) when the temperature was increased above LCST. This increase can be attributed to changes in the refractive index of the guest molecule because its POEGMA arms underwent a phase transition from random coil to condensed globule.15,43,44 This change clearly shows that the micelles began to form near the LCST; this result from DLS study was consistent with that following TEM investigation.The self-assembly mechanism of the host–guest composite system was further confirmed by comparing the TEM images of the polymeric solutions, which were prepared at 25 °C and 50 °C. Fig. 8 shows the representative TEM micrographs of noncovalently connected micelles formed from Ad-POEGMAs (A1) and 1
:
1 host–guest mixtures of Ad-POEGMAs (A1) and PGCD at 25 °C and 50 °C, respectively. From the TEM micrographs, At 25 °C, the Ad-POEGMAs dissolved in water as uneven and loose single- or multi-molecular spherical particles with diameters ranging from 10 nm to 100 nm, whereas larger micelles 150–230 nm in diameter were observed for the sample solution prepared and dried at 50 °C, showing that the noncovalently connected micelles formed at higher temperature were larger. In contrast, spherical uneven and loose nano-assembled vesicles were observed at 25 °C for host–guest mixtures of A1 and PGCD, which agreed quite well with the results measured by DLS at the same temperature. These results demonstrate that nano-sized aggregates indeed formed via inclusion complexation of Ad-POEGMAs and PGCD. For the host–guest composite system of A1 and PGCD, dense, dark spherical particles were observed, which was especially clear for the sample prepared and dried at 50 °C. This result is due to the action of the staining agent, phosphotungstic acid, which stains the hydrophobic segments to a greater extent. However, it was found that the diameters of the micelles from the TEM micrographs were clearly smaller than those from DLS measurements. This result may be related to the fact that DLS measures the hydrodynamic diameters of the micelles in an aqueous environment, whereas the TEM micrographs show dehydrated solid states of the micelles.17
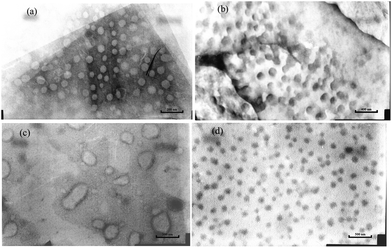 |
| Fig. 8 Typical tem images obtained by aqueous solutions of A1 (a and b) and A1 + PGCD (c and d) (3 mg mL−1) at 25 and 50 °C. | |
Furthermore, obvious differences in morphology were observed by comparing the TEM images of the nano-assemblies. Those constructed from Ad-POEGMAs showed relatively light aggregates below the LCST and dark, condensed globules above, presenting typical micellar characteristics of POEGMA-based polymers.39,45 For those that formed from host–guest mixtures of A1 and PGCD, a strong contrast between the light center and the dark, thin periphery was observed below the LCST, which is characteristic of vesicular nano-structures.46,47 When the temperature rose above the LCST, dense aggregates formed. Hence, from the results of DLS and TEM, it was found that nano-assemblies with different morphologies can be constructed from Ad-POEGMAs and the supramolecular system of Ad-POEGMAs and PGCD by simply elevating the temperature. In such cases, Ad-POEGMAs formed micelle-like nano-structures, and the supramolecular system of Ad-POEGMAs and PGCD organized into vesicle-like nano-aggregates. The procedures for inclusion complexation, thermally induced dehydration, and subsequent self-assembly of the pseudo-graft polymer based on Ad-POEGMAs and PGCD are illustrated in Scheme 3.
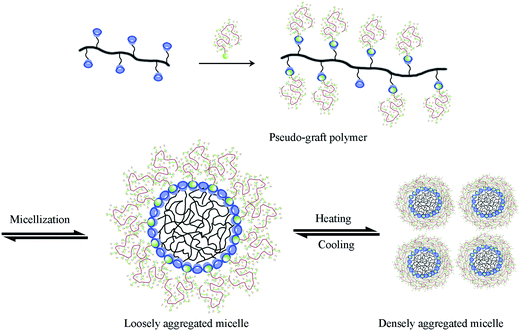 |
| Scheme 3 The procedures for inclusion complexation, the thermally induced dehydration and subsequent self-assembly of the pseudo-graft polymer based on Ad-POEGMAs and PGCD. | |
Conclusions
Thermally responsive Ad-POEGMAs were synthesized and shown to have tunable LCST behavior in aqueous solutions. These polymers were able to self-assemble into noncovalently connected micelles with the Ad segments as the cores and hydrophilic POEGMAs as the coronas. Moreover, a composite system was designed using Ad-terminated POEGMAs and PGCD, which was able to form thermoresponsive pseudo-graft polymers in aqueous solutions via host–guest complexation between the β-CD core of the host polymer and the adamantyl moiety of the guest polymer. This polymer stabilized by single CD–Ad complexes and by pairs of CD–Ad complexes. The pseudo-graft polymer was shown to self-assemble to form nano-sized aggregations above and below the LCST in aqueous solutions. As a result of the tunability of its thermoresponsive behavior, noncovalent connected micelle-forming ability, and potential biocompatibility, the pseudo-graft polymer shows potential interest for applications in biomedical science.
Materials and methods
Materials
β-Cyclodextrin was purchased from Sigma and was used after recrystallization from water and was dried at 100 °C under vacuum. Glycidyl methacrylate (GMA) was purchased from TCI, Japan. p-Toluenesulfonyl chloride (p-TsCl), ethanediamine, and azoisobutyronitrile (AIBN) were all purchased from Sigma, China. Copper(I) bromide was washed with glacial acetic acid in order to remove any soluble oxidized species, then was filtered, washed with ethanol, and dried. 2-Bromopropiomyl bromide, 1-adamantanamine and 2,2-bipyridine were obtained from Aldrich. 2-(2-Methoxyethoxy) ethyl methacrylate (MEO2MA, Mn = 188 g mol−1) and oligo (ethylene glycol) methyl ether methacrylate (OEGMA, Mn = 475 g mol−1) were acquired from Aladdin. Tetrahydrofuran (THF) was initially dried over sodium wire and refluxed over potassium for three days before use. Dichloromethane (CH2Cl2) and N,N-dimethylformamide (DMF) were refluxed over CaH2 separately before use. Ethanol was also refluxed over CaH2 before use. All other reagents were used as received without further purification.
Characterization
Fourier transform infrared (FT-IR) spectra were recorded on a Nicolet 5100 spectrometer by the KBr sample holder method in the fundamental region of 400–4000 cm−1. 1H NMR spectra were obtained by a Bruker DMX-400 spectrometer. Deuterated chloroform (CDCl3), deuterated water (D2O), and deuterated dimethyl sulfoxide (DMSO-d6) were used as solvents. The MALDI-TOF mass spectrum was recorded in the reflector mode on a Bruker Autoflex III Smartbeam mass spectrometer using a nitrogen laser, and α-cyano-4-hydroxycinnamic acid (CCA) was used as the matrix. The number-average molecular weight (Mn) and polydispersity index (Mw/Mn) of each polymer were determined at 35 °C using a Waters 1515 size exclusion chromatograph (SEC) equipped with a Waters 2414 refractive index (RI) detector. DMF and THF were used as eluants, and the columns used were the styragel HR3 and HR4 columns calibrated by narrow PS standards.
The LCSTs of the polymers were determined by UV-vis spectroscopy (U-3010 Spectrophotometer). The transmittance of the polymeric aqueous solution (3 mg mL−1) was recorded at temperatures ranging from 20 °C to 65 °C. The lower critical solution temperature (LCST) value of the aqueous polymer solution at a specific concentration was determined as the temperature corresponding to a 10% decrease in optical transmittance. The hydrodynamic diameters (Dh) of the capsules and their polydispersity indices (PDI) were determined by dynamic light scattering (DLS) on a Malven Zetasizer Nano System (Nano-zs90). The solutions were passed through 0.45 μm filters before DLS measurement. The measurements were conducted in a 3.0 mL quartz cuvette using a 670 nm diode laser, and the scattering angle used was 90°.
The micromorphology was visualized by transmission electron microscopy (TEM). The samples were prepared by placing polymer aqueous solutions on copper grids in a biochemical incubator thermostated at 25 °C or 50 °C and were stained with phosphotungstic acid before TEM observation with a JEM-100CX II microscope operated at 80 kV.
Sample synthesis
(a) Synthesis of mono-6-OTs-CD (TCD). The synthesis of TCD was conducted according to that in previous literature with a few modifications.33,34 β-CD (24 g) was suspended in 180 mL of water. NaOH (2.623 g) in 20 mL of water was added dropwise over a 30 min period and was reacted under vigorous agitation at 0 °C for a period of 1 h. Then p-toluenesulfonyl chloride (4.032 g) in 20 mL of acetonitrile was added dropwise for a period of 1 h, which causing immediate formation of a white precipitate. After 3 h of stirring at 20 °C, the unreacted toluenesulfonyl chloride was filtered off. The solution was then neutralized and refrigerated, and the precipitate was recovered by suction filtration and recrystallization in water. The sample obtained was dried at 60 °C for 48 h in a vacuum oven, and TCD was obtained as a white solid (5.14 g yield: 21.40%). IR (KBr, cm−1): 3388 (s, OH), 2928 (w, CH2), 1597 (s, Ph). 1H NMR (400 MHz, DMSO-d6): δ 7.42 (2H), 7.74 (2H), 4.75 (7H), 3.49–3.64 (28H), 3.28–3.36 (14H), 2.42 (3H). 13C NMR (DMSO): δ 144.9 (s), 132.7 (s), 129.9 (s), 127.6 (s), 102.3 (m), 81.5 (m), 71.9–73.1 (m), 69.8, 69.0, 59.5 (t), 21.3 (s) ppm. MALDI-TOF on CCA matrix (TCD + Na+) m/z calcd for C49H76NaO37S (TCD + Na+) 1311.37, measured 1311.13.
(b) Synthesis of mono (6-amino-6-deoxy)-β-CD (ECD). 5.0 g of TCD was reacted with an excess amount of EDA (30 mL) in 20 mL DMF at 80 °C for 6 h. After the reaction was completed, the mixture was allowed to cool to room temperature and was then precipitated with acetone. The sample obtained was dried at 40 °C for 72 h in a vacuum oven, and ECD was obtained as a white solid (3.96 g yield: 79.10%). IR (KBr, cm−1): 3388 (s, OH, NH2), 2928 (w, CH2). 1H NMR (400 MHz, DMSO-d6): δ 4.85 (7H), 3.54–3.64 (28H), 3.27–3.41 (14H), 2.72–2.92 (4H). 13C NMR (DMSO): δ 102.0 (s), 81.6 (s), 72.1–73.1 (m), 60.0 (s), 40.1–39.5 (m) ppm. MALDI-TOF on CCA matrix (ECD + Na+) m/z calcd for C44H76N2NaO34 (ECD + Na+) 1199.42, measured 1199.20.
(c) Synthesis of aminoethyl methacrylate β-cyclodextrin (GCD). 5.98 g ECD was dissolved in 30 mL DMF, and a small amount of inhibitor was added. Then 2.49 g GMA was added dropwise. After the reaction was completed, the mixture was allowed to cool to room temperature, then was precipitated with acetone and finally dried at room temperature for 2 days under vacuum (4.90 g yield: 82.0%). IR (KBr, cm−1): 3388 (s, OH), 2928 (w, CH2), 1720 (w, C
O). 1H NMR (400 MHz, DMSO-d6): δ 6.05 (2H), 4.81 (7H), 4.48 (1H), 3.54–3.62 (28H), 3.29–3.43 (14H), 2.72–2.92 (6H), 1.87 (3H). 13C NMR: (DMSO) δ 166.6 (s), 135.9 (s), 125.8 (s), 102.0 (s), 81.6 (s), 72.0–73.1 (m), 66.9 (s), 59.9 (s), 56.1 (s), 40.1–39.5 (m), 20.6 (t), ppm. MALDI-TOF on CCA matrix (GCD + Na+) m/z calcd for C51H86N2NaO37 (GCD + Na+) 1341.48, measured 1341.09.
(d) Homopolymerization of aminoethyl methacrylate β-cyclodextrin (PGCD). Radical copolymerizations of GCD were initiated by AIBN. A typical experiment for the polymerization procedure is as follows: in a 50 mL three-neck round-bottom flask, GCD (1.5 g) and AIBN (0.1 g) were mixed with 10 mL of DMF. The solution was bubbled under argon for 30 min at room temperature to remove free oxygen. The flask was then immersed in an oil bath thermostated at 80 °C, and the reaction was maintained for 6 h under argon. Homopolymer was directly precipitated by acetone, washed at least three times using a large amount of acetone, then dried at 50 °C under vacuum to yield a fine white powder.
(e) Synthesis of 1-adamantyl-2-bromopropionamide (AdBB). The ATRP initiator, AdBB, was prepared by the amidation reaction of 1-adamantanamine with 2-bromopropiomyl bromide. A 100 mL round-bottom flask was charged with 1-adamantanamine (3.03 g), TEA (3.04 g), and dry CH2Cl2 (30 mL). The mixture was cooled to 0 °C in an ice-water bath. 2-bromopropiomyl bromide (6.48 g) in THF (20 mL) was then added dropwise over 2 h. After the addition was completed, the reaction mixture was stirred at 0 °C for an additional 2 h and then at 30 °C for 24 h. The mixture was allowed to cool to room temperature, and the insoluble salts were removed by suction filtration. The organic solution was washed with an aqueous sodium bicarbonate solution (2 × 100 mL) and distilled water (2 × 100 mL) and was then dried with anhydrous magnesium sulfate and concentrated by a rotary evaporator. The concentrated syrup was purified by silica gel column chromatography using ethyl acetate–petroleum ether (1
:
3 v/v) as the eluent. The solvents were then removed by a rotary evaporator and dried in vacuum oven at 50 °C for 2 days. AdBB was obtained as a white solid (1.24 g, yield: 82%). 1H NMR (CDCl3): δ (ppm) 5.99 (1H, NHCO), 4.25–4.30 (1H, BrCHCH3), 2.07 (3H, –CH), 1.98 (6H, –CH2), 1.81 (3H, CH3–Br), 1.63 (6H, CH2). 13C NMR (CDCl3): δ (ppm) 168.1 (C
O), 52.3(C–O), 46.1 (BrCH), 41.1 (CH2), 36.2 (CH2), 29.3 (CH3), 23.1 (CH).
(f) Synthesis of adamantyl-terminated P(MEO2MA-co-OEGMA) (Ad-POEGMAs). Ad-POEGMAs were synthesized by the ATRP of MEO2MA and OEGMA monomer using AdBB as the initiator. A typical procedure is described as follows: a Schlenk tube was purged with dry argon for 30 minutes, and a degassed mixture of 2-(2-methoxyethoxy) ethyl methacrylate (95 eq.), oligo (ethylene glycol) methyl ether methacrylate (5 eq.), ethanol (monomers–ethanol ∼1
:
1.5 v/v), AdBB (1 eq.) initiator and copper bromide (1 eq.) was added to the Schlenk tube. The mixture was degassed via three freeze–thaw–pump cycles and was back-filled with argon. Then 2,2-bipyridyl (2 eq.) was added. The mixture was heated at 60 °C in an oil bath for 6 h. The experiment was stopped by opening the flask and exposing the catalyst to air. The final mixture was diluted in ethanol and was passed through a short neutral alumina column (200 mesh) in order to remove the copper catalyst. Then, the filtered solution was diluted with ethanol and was subsequently purified by dialysis in water (molecular weight cut-off: 3500). Finally, after freeze-drying in a vacuum, Ad-POEGMAs were obtained as a viscous solid. The synthetic routes employed for the preparation of β-CD-based monomers and PGCD, in addition to the adamantane-based initiator and adamantyl-terminated POEGMAs, are shown in Scheme 1.
Acknowledgements
The authors appreciate the Integration of Industry, Education and Research of Guangdong Province project (project no. 2011A091000007), Guangdong-Hongkong Technology Cooperation Funding (project no. 2009A091300012) and the National Natural Science Foundation of China (project no. 20974121). They also wish to thank Professor Yu Qijun (South China University of Technology) for his support and collaboration in the test involved in this study.
References
- C. Weber, R. Hoogenboom and U. S. Schubert, Prog. Polym. Sci., 2012, 3, 686 CrossRef PubMed.
- M. Y. Guo and M. Jiang, Soft Matter, 2009, 5, 495 RSC.
- G. S. Chen and M. Jiang, Chem. Soc. Rev., 2011, 40, 2254 RSC.
- Y. Y. Mai and A. Eisenberg, Chem. Soc. Rev., 2012, 41, 5969 RSC.
- M. A. Ward and T. K. Georgiou, Polymers, 2011, 3, 1215 CrossRef CAS PubMed.
- F. Liu and M. W. Urban, Prog. Polym. Sci., 2010, 35, 3 CrossRef CAS PubMed.
- C. Tsitsilianis, G. Gotzamanis and Z. Iatridi, Eur. Polym. J., 2011, 47, 497 CrossRef CAS PubMed.
- J. Zou, B. Guan, X. J. Liao, M. Jiang and F. G. Tao, Macromolecules, 2009, 42, 7465 CrossRef CAS.
- Z. X. Zhang, X. Liu, F. J. Xu, X. J. Loh, E. T. Kang, K. G. Neoh and J. Li, Macromolecules, 2008, 41, 5967 CrossRef CAS.
- F. Sakai, G. S. Chen and M. Jiang, Polym. Chem., 2012, 3, 954 RSC.
- Z. S. Ge and S. Y. Liu, Macromol. Rapid Commun., 2009, 30, 1523 CrossRef CAS PubMed.
- A. K. Bajpai, S. K. Shukla, S. Bhanu and S. Kankane, Prog. Polym. Sci., 2009, 33, 1088 CrossRef PubMed.
- D. Roy, J. N. Cambre and B. S. Sumerlin, Prog. Polym. Sci., 2010, 35, 278 CrossRef CAS PubMed.
- S. Han, M. Hagiwara and T. Ishizone, Macromolecules, 2003, 36, 8312 CrossRef CAS.
- J. F. Lutz, O. Akdemir and H. Ann, J. Am. Chem. Soc., 2006, 128, 13046 CrossRef CAS PubMed.
- Z. X. Zhang, K. L. Liu and J. Li, Macromolecules, 2011, 44, 1182 CrossRef CAS.
- C. G. Mu, X. D. Fan, W. Tian, Y. Bai and X. Zhou, Polym. Chem., 2012, 3, 1137 RSC.
- C. R. Becer, S. Hahn, M. W. M. Fijten, H. M. L. Thijs, R. Hoogenboom and U. S. Schubert, J. Polym. Sci., Part A: Polym. Chem., 2008, 46, 7138 CrossRef CAS.
- O. G. Schramm, G. M. Pavlov, H. P. Erp, M. A. R. Meier, R. Hoogenboom and U. S. Schubert, Macromolecules, 2009, 42, 1808 CrossRef CAS.
- E. W. Edwards, M. Chanana, D. Y. Wang and H. Möhwald, Angew. Chem., Int. Ed., 2008, 47, 320 CrossRef CAS PubMed.
- J. F. Lutz, K. Weichenhan, O. Akdemir and A. Hoth, Macromolecules, 2007, 40, 2503 CrossRef CAS.
- J. F. Lutz, J. Polym. Sci., Part A: Polym. Chem., 2008, 46, 3459 CrossRef CAS.
- H. Kitano, T. Hirabayashi, M. Gemmei-Ide and M. Kyogoku, Macromol. Chem. Phys., 2004, 205, 1651 CrossRef CAS.
- M. Miyauchi and A. Harada, J. Am. Chem. Soc., 2004, 126, 11418 CrossRef CAS PubMed.
- J. Wang and M. Jiang, J. Am. Chem. Soc., 2006, 128, 3703 CrossRef CAS PubMed.
- L. Z. Wang, Y. M. Yang, M. R. Zhu, G. J. Qiu, G. L. Wu and H. Gao, RSC Adv., 2014, 4, 6478 RSC.
- L. Janus, B. Carbonnier, A. Deratani, M. Bacquet, G. Crini, J. Laureynsd and M. Morcellet, New J. Chem., 2003, 27, 307 RSC.
- R. Machín, J. R. Isasi and I. Vélaz, Carbohydr. Polym., 2012, 87, 2024 CrossRef PubMed.
- Y. Ping, C. Liu, Z. Zhang, K. L. Liu, J. Chen and J. Li, Biomaterials, 2011, 32, 8328 CrossRef CAS PubMed.
- Z. S. Ge, H. Liu, Y. F. Zhang and S. Y. Liu, Macromol. Rapid Commun., 2011, 32, 68 CrossRef CAS PubMed.
- W. Tian, A. L. Lv, Y. C. Xie, X. Y. Wei, B. W. Liu and X. Y. Lv, RSC Adv., 2012, 2, 11976 RSC.
- J. Wu, P. H. Ni, M. Z. Zhang and X. L. Zhu, Soft Matter, 2010, 6, 3751 RSC.
- R. C. Petter, J. S. Salek, C. T. Sikorski, G. Kumaravel and F. T. Lin, J. Am. Chem. Soc., 1990, 112, 3860 CrossRef CAS.
- Y. Y. Liu, X. D. Fan and L. Gao, Macromol. Biosci., 2003, 3, 715 CrossRef CAS.
- Y. W. Li, H. L. Guo, Y. F. Zhang, J. Zheng, Z. X. Li, C. H. Yang and M. G. Lu, Carbohydr. Polym., 2014, 102, 278 CrossRef CAS PubMed.
- Y. L. Liu, Z. Q. Wang and X. Zhang, Chem. Soc. Rev., 2012, 41, 5922 RSC.
- T. Ishizone, A. Seki, M. Hagiwara, S. Han, H. Yokoyama, A. Oyane, A. Deffieux and S. Carlotti, Macromolecules, 2008, 41, 2963 CrossRef CAS.
- Y. Maeda, T. Kubota and H. Yamauchi, Langmuir, 2007, 23, 11259 CrossRef CAS PubMed.
- B. L. Peng, N. Grishkewich, Z. L. Yao, X. Han, H. L. Liu and K. C. Tam, ACS Macro Lett., 2012, 1, 632 CrossRef CAS.
- S. T. Sun and P. Y. Wu, Macromolecules, 2013, 46, 236 CrossRef CAS.
- H. Liu, Y. F. Zhang, J. M. Hu, C. H. Li and S. Y. Liu, Macromol. Chem. Phys., 2009, 210, 2125 CrossRef CAS.
- L. Li, X. H. Guo, J. Wang, P. Liu, R. K. Prud'homme, B. L. May and S. F. Lincoln, Macromolecules, 2008, 41, 8677 CrossRef CAS.
- J. G. Zeng, K. Y. Shi, Y. Y. Zhang, X. H. Sun and B. L. Zhang, Chem. Commun., 2008, 3753 RSC.
- L. H. He, J. Huang, Y. M. Chen and L. P. Liu, Macromolecules, 2005, 38, 3351 CrossRef CAS.
- X. Y. Huan, D. L. Wang, R. J. Dong, C. L. Tu, B. S. Zhu, D. Y. Yan and X. Y. Zhu, Macromolecules, 2012, 45, 5941 CrossRef CAS.
- Y. Chen, X. H. Pang and C. M. Dong, Adv. Funct. Mater., 2010, 20, 579 CrossRef CAS.
- O. Kretschmann, C. Steffens and H. Ritter, Angew. Chem., Int. Ed., 2007, 46, 2708 CrossRef CAS PubMed.
|
This journal is © The Royal Society of Chemistry 2014 |
Click here to see how this site uses Cookies. View our privacy policy here.