DOI:
10.1039/C4RA02983F
(Paper)
RSC Adv., 2014,
4, 25675-25682
One pot synthesis of coordination polymer 2,5-dimercapto-1,3,4-thiadiazole–gold and its application in voltammetric sensing of resorcinol†
Received
3rd April 2014
, Accepted 16th May 2014
First published on 23rd May 2014
Abstract
Herein, we report a one pot synthesis of a coordination polymer using 2,5-dimercapto-1,3,4-thiadiazole (DMTD) with gold (Au) and the characterization of the polymer by FTIR, Raman spectroscopy, UV-visible spectroscopy, XPS, XRD, 13CNMR, HRTEM, gel permeation chromatography and TGA. Results show that Au ions get coordinated by the diazole and sulfydryl groups of DMTD throughout the polymeric chain. The polymeric layers are stabilized through π–π stacking and hydrophobic interaction. XRD data reveals the crystalline nature of DMTD–Au coordination polymer. The kinetic parameters, such as the energy of activation and the regression value of the DMTD–Au decomposition, have been determined by exploiting thermogravimetric data. HRTEM analysis establishes the nanostructure of the polymeric material DMTD–Au. Its voltammetric study reveals that the synthesized coordination polymer DMTD–Au exhibits excellent electroactivity towards resorcinol and facilitates fast electron transfer kinetics, which is employed for electro-sensing applications. To the best of our knowledge we are the first to report this coordination polymer DMTD–Au modified carbon paste electrode (DMTD–Au/CPE) for the electrochemical detection of resorcinol (RS). The DMTD–Au modified carbon paste electrode has been found to be highly sensitive towards resorcinol due to its strong interaction through intermolecular hydrogen bonding. Under the optimized conditions, the modified electrode exhibit resorcinol oxidation at low over potential (0.55 V) and the anodic peak current shows a linear response with sensitivity and limit of detection of 0.019 μA nM−1 and 29.77 nM, respectively, at a S/N (signal-to-noise ratio) of 3.
1. Introduction
In recent years, the study of coordination polymers (CP) has emerged as a vibrant area of research. Coordination polymers are a family of compounds, which are considered to be metal–organic frameworks with more than two physical/chemical properties in one (like hybrid materials). CPs are formed by the incorporation of metal building blocks in multi-dentate organic or inorganic bridging ligands.1,2 The design of efficient coordination polymers encapsulated with noble metal ions has received significant interest in the past two decades. Coordination polymers have been widely used for numerous applications such as sensing,3 water purification,4 hydrogen fuel storage,5 heterogeneous catalysis or catalyst-supporting energy-storage devices such as lithium ion secondary batteries6,7 and double layer capacitors.8,9 Therefore, there is an increasing demand for the synthesis of such coordination polymers for commercial purposes through facile routes without catalyst at room temperature.10–12
In this scenario, 2,5-dimercapto-1,3,4-thiadiazole (DMTD) may be of significant interest for constructing CPs with interesting properties because of its four possible bonding sites (diazole nitrogen atoms and sulfydryl sulfur donors), which provide feasibility for metal ions to be linked with the ligand and thus expanding the chain dimensions. Furthermore, azole rings are superior in terms of hydrogen bonding and π–π stacking.13
We explore the possibility of synthesizing the coordination polymer DMTD–Au under optimal conditions in the absence of catalysts or without extra control of other experimental conditions. A simple reaction design is employed, in which the concentration of Au(III) and DMTD and the reaction conditions are systematically varied with the progress of the reaction and product, which is selectivity monitored by several instrumental techniques. This compound is also compared with the Au(0)–DMTD composite to understand the difference in the coordination polymer and the composite of the two components.14 Ultimately, this reaction process leads to an enhanced practical utility of the synthesized coordination polymer DMTD–Au, thereby providing a platform for the production of such polymers with physical properties competitive with those of the existing coordination polymers.
This is the first time gold salt has been used for the synthesis of a coordination polymer; the structural features of the polymer have been established and it has been applied in the voltammetric sensing of resorcinol (RS) up to nanomolar level. Phenolic compounds, which are extensively used in tanning, cosmetics, dyes, pesticides, flavoring agents, medicines, antioxidants and photography, are highly toxic and poorly degradable in the environment and have therefore been listed as environmental pollutants by the US Environmental Protection Agency (EPA) and European Union (EU).15–18 Resorcinol is a phenolic compounds with high toxicity; it can be easily absorbed through the gastric tract and human skin, which can cause dermatitis, catarrh, convulsion and cyanopathy.19,20 In recent years, a numerous have been applied for the determination of RS such as spectrophotometry, high-performance liquid chromatography, quartz crystal microbalance, surface Plasmon resonance and fluorescence spectroscopy.21–23 However, these methods require expensive and sophisticated instrumentation or complicated sample preparation with a moderate limit of detection. Electrochemical technique has always offered simple instrumentation, low cost and portability. In this process, the concept of a modified electrode is one of the exciting fields of electroanalytical chemistry.24–26 Several existing electrochemical methods for RS sensing are based on the immobilization of an enzyme on a modified electrode. We have demonstrated a simple non-enzymatic, highly accurate and rapid voltammetric method with a DMDT–Au modified carbon paste electrode for the ultra trace level sensing of RS within a wide range of concentration.
2. Experimental
2.1. Materials
2,5-dimercapto-1,3,4-thiadiazole (DMTD) and HAuCl4 were purchased from Sigma-Aldrich (India). Ethanol, resorcinol, sodium dihydrogen phosphate and disodium hydrogen phosphate (SRL, India) were used as received unless mentioned otherwise. The glassware used in the synthesis of the polymer was cleaned with freshly prepared aqua regia (3
:
1, HCl–HNO3) and rinsed comprehensively with ultrapure water (Merck India).
2.2. Synthesis of coordination polymer (DMTD–Au)
In a typical synthesis of coordination polymer (DMTD–Au), ethanolic solution of HAuCl4 (5.95 mM) was added to 2,5-dimercapto-1,3,4-thiadiazole (17.7 mM, prepared in ethanol solution) under vigorous stirring for 18 h at 22 °C under a thin stream of nitrogen gas (shown in Scheme 1) to avoid partial oxidation of the precursor molecule. Initially, the monomeric solution was clear and transparent, which turned turbid brown during the course of the reaction, and finally a pale yellow precipitate was obtained. The pale yellow precipitate was purified by washing several times with water–ethanol mixture (30
:
70 v/v) to remove un-reacted DMTD and gold salt, and thereafter dried under vacuum at 28 °C for 15 h. The yield obtained was 64% and the product was stored below 25 °C for further use.
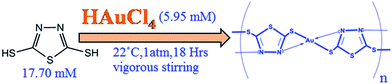 |
| Scheme 1 Reaction scheme for synthesis of co-ordination polymer DMDT–Au. | |
2.3. Instrumentation
Infrared spectra in KBr pellets were recorded with a Fourier transform infrared (FTIR) spectrometer (Nicolet-6700, USA) scanning from 2500 to 400 cm−1. Raman spectra were measured with a Micro Raman Spectrometer at 180° scattering geometry (Renishaw, Germany) with a 514.5 nm line of an Ar+ laser at 50 mW at room temperature. TEM measurements were performed with a Jeol Jem 2010 electron microscope operating at 200 kV on a carbon-coated copper grid modified with 5 μL of the polymer solution in ethanol. 13C NMR spectra of the resulting polymer were recorded on a JEOL Al300 NMR (300 MHz) in DMSO-d6 solvent and reported in parts per million (δ) from residual solvent peak. Thermogravimetric analysis (TGA) was performed on a NETZSCH, STA 409 PC analyzer at a heating rate of 20 °C min−1 under a flow of nitrogen. X-ray diffraction patterns for powder samples of the coordination polymer DMTD–Au and DMTD were recorded with 18 kW anode powder X-ray diffractometer rotating from 10° to 80°, Rigaku, Japan with Cu-Kα radiation operating in Bragg–Brentano geometry and fitted with a graphite monochromator in diffracted beam with a 3° min−1 scan rate. UV-vis absorption spectra were recorded using a Perkin Elmer Lambda-25 spectrophotometer using a quartz cuvette with an optical path length of 1 cm. Elemental analysis was recorded with X-ray photoelectron (Kratos Analytical Instrument, Shimadzu group company, Amicus XPS UK). The number-average molecular weight (Mn) and polydispersity index (Mw/Mn) were determined by Youglin ACME 9000 gel permeation chromatography in DMF at 40 °C with a flow rate of 0.5 mL min−1 on two polystyrene gel columns [PL gel 5 μm 10E 4 Å columns (300 7.5 mm)], which were connected in series to a gradient pump and a RI detector. The columns were calibrated against seven poly(methyl methacrylate) (PMMA) standard samples (Polymer Lab, PMMA Calibration Kit, M-M-10). Electrochemical workstation (model-CHI7041C, CH-Instrument Inc., USA) was used for electrochemical measurement using a three-electrode assembly (as shown in Fig. 9) consisting of a DMTD–Au carbon paste modified electrode as the working electrode, Pt foil as the counter electrode and Ag/AgCl as the reference electrode for all the electrochemical measurements. Neutral phosphate buffer (pH 7.0) was used as a supporting electrolyte and the scan rate was maintained at 50 mV s−1 for each measurement.
2.4. Modified carbon paste electrode preparation for RS sensing
The active carbon paste was prepared by mixing a known amount of DMTD–Au (2.5% w/w) with graphite powder (67.5% w/w) in a pestle and mortar followed by the addition of Nujol oil (30% w/w). The absence and presence of DMTD–Au in the active paste resulted in the formation of unmodified CPE and modified CPE/DMTD–Au systems. The mixture was thoroughly homogenized and filled manually into the cavity (1 mm diameter) of the electrode body. The electrode surface was smoothed on clean butter paper applying pressure to achieve better electrical contact.
3. Results and discussion
3.1. Structural, morphological and molecular weight studies of the synthesized coordination polymer DMDT–Au
The FTIR spectra of DMTD–Au and DMTD were recorded in 400–2500 cm−1 region, as shown in Fig. 1. In the case of DMTD, the region from 2450–2350 cm−1 contains several weak bands, which are derived from C–H stretching overtones and Fermi resonances. Peaks appearing in the region 1300–1205 cm−1 are assigned to ring modes coupled to (exocyclic) C–C stretch and a ring stretching mode. The most prominent of these are the asymmetric stretches (νas) at 1500 cm−1 and the symmetric stretch (νs) at 1407 cm−1 of C
N; the νas and νs modes of C–S–C stretches have been assigned to peaks at 718 and 658 cm−1, respectively. The peak at 1075 cm−1 is assigned due to an out of phase combination of N–N and symmetric C–S–C stretches which get reduced to 1049 cm−1 in DMDT–Au. DMTD–Au shows a C
N band at 1384 cm−1. Reduction in the intensity of the C
N bond and the stretching frequency of C
N in DMDT–Au indicates the interaction of the nitrogen atom with gold, which is also confirmed by Raman spectroscopy.
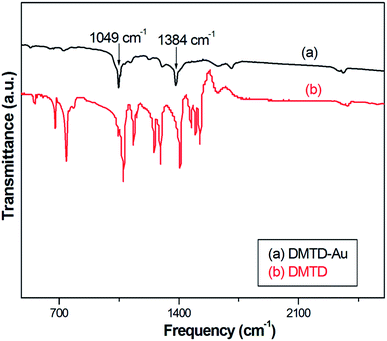 |
| Fig. 1 FT-IR spectra of DMTD–Au (a) and DMTD (b). | |
The Raman spectrum of DMTD–Au, shown in Fig. 2, exhibits bands at 380 and 546 cm−1, which arise due to Au–S and Au–N linkage, respectively, ensuring the metal–sulphur and metal–nitrogen linkage.27 Further, a band at 660 cm−1 in the Raman spectrum is due to the symmetric C–S–C stretch, while the absence of an absorption peak near 522 cm−1 clearly indicates the absence of an S–S bond in the coordination polymer DMTD–Au. The results from FTIR and Raman spectroscopy reveal that gold ions get coordinated between the DMTD molecules throughout the polymer chain.
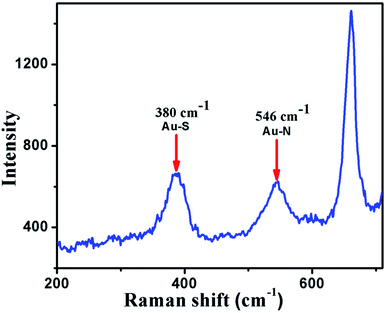 |
| Fig. 2 Raman spectrum of coordination polymer DMTD–Au. | |
The crystal structure of DMTD–Au investigated by powder X-ray diffraction (XRD) is shown in Fig. 3. Fig. 3(a) and (b) show the diffraction pattern of DMTD–Au and DMTD, respectively, while Fig. 3(c) corresponds to the diffraction pattern of fully reduced gold obtained from JCPDS file CAS number 7440-57-5. Indexing for the diffraction peaks of DMTD–Au was performed by Dicvol software, which showed that the diffraction features appearing at 2θ values of 28.28°, 40.44°, 50.10°, 58.58°, 66.30° and 73.56° corresponded to the (100), (110), (112), (200), (202) and (114) planes, respectively, for the tetragonal system. Sharp diffraction peaks show the crystalline characteristics of the polymer DMTD–Au. The present study reveals not only the crystalline nature of the coordination polymer but it also demonstrates the absence of fully reduced gold within the coordination sphere, supporting the XPS results.
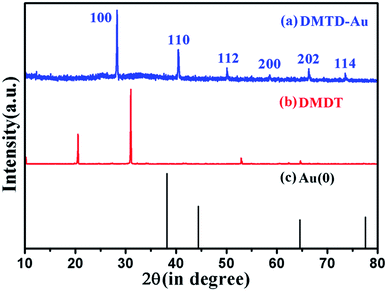 |
| Fig. 3 XRD of DMTD–Au (a), DMTD (b) and gold (c) obtained from JCPDS file CAS number 7440-57-5. | |
The XPS study of DMTD–Au (Fig. 4) revealed binding energy values of 85.41 eV and 89.11 eV, as shown in Fig. 4(a), corresponding to Au(I) 4f7/2 and Au 4f5/2, respectively.28,29 The Au 4f7/2 binding energy is shifted in DMTD–Au. The direction of this shift clearly corresponds to Au being in an oxidized state in DMDT–Au, and its magnitude is similar to that observed for Au(I). The experimental photoemission curve of S 2p signal in Fig. 4(b) can be fitted in two doublets, i.e., two different atomic environments of sulfur with 2p3/2 components peaking at 161 and 162.5 eV. The first one can be assigned to aromatic sulfur while the second one is related to sulfur attached to a gold atom.30–32 The nitrogen 1s binding energy (BE) of 400.0 eV in Fig. 4(c) corresponds to the N–N binding energy.33–35 The BE is referenced to C(1s) of C
N binding energy near 287 eV (ref. 36) in Fig. 4(d) because electronegative substituents decrease the electron density of carbon atoms, causing a small increase in C (1s) binding energy.
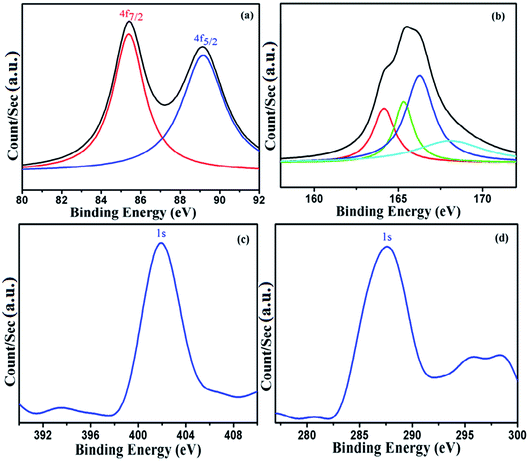 |
| Fig. 4 XPS spectra of DMTD–Au for Au 4f (a), S 2p (b), N 1s (c) and C 1s (d) regions. | |
The HRTEM image in Fig. SI-1† shows that the rigid molecules of the co-ordination polymer DMTD–Au are arrayed in the coordination polymer sphere with an average diameter of about 170 nm as nanospheres. It may be assumed that the rigid coordination polymer DMTD–Au molecules are arrayed in parallel due to the π–π stacking of adjacent molecular chains in the same plane. The corresponding SAED pattern clearly shows that the synthesized coordination polymer has crystallinity, which supports the XRD result, as shown in Fig. 3. The synthesized coordination polymer DMTD–Au has a prominent peak at a chemical shift value of 206.95 ppm (Fig. 5), showing a strong deshielding of the aromatic carbon as a chemical shift value of 177 ppm is reported for DMTD.37 Down field shielding in chemical shift value for DMDT–Au signifies the interaction of the metal with the DMDT molecule. It shows that the aromatic carbon atoms are in a strong electronegative environment as in nature.
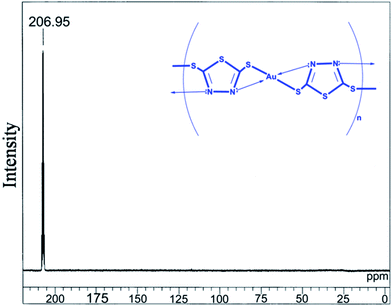 |
| Fig. 5 13CNMR spectra of DMTD–Au in DMSO-d6 at room temperature. | |
Considering various characterization techniques, a possible chemical structure of DMDT–Au has been proposed, as shown in Fig. 6, which is in accordance with 13CNMR, FTIR, RAMAN, HRTEM and XPS results.
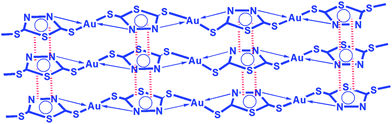 |
| Fig. 6 Proposed structure of synthesized coordination polymer DMDT–Au. | |
The average molecular weight (approximate) and distribution of coordination polymer DMTD–Au was determined by gel permeation chromatography using PMMA as a standard. Fig. SI-2† shows the chromatogram, which clearly indicates that the average molecular weight of the dominant fraction is located at around Mn = 370
000 g mol−1 (PDI 2.32). The minuscule humps are because of the fragmentation of polymeric chains and traces of lower molecular weight polymeric fragments. The UV-vis spectrum of coordination polymer DMDT–Au is shown in Fig. 7. The maximum absorption peak at around 433 nm and the shoulder peak at 323 nm are attributed to π–π* and MLCT (metal to ligand charge transfer transition) (Au → π*) transitions, respectively.38 Thermal analysis of the polymeric sample was performed using thermal gravimetric analysis (TGA) (Fig. 10) under a N2 atmosphere by varying the temperature of the instrument from room temperature to 800 °C at a heating rate of 20 °C min−1. The first weight loss (2%), which occurred in the temperature range 40–140 °C, accounts for the uncoordinated water molecules, which includes the lattice and adsorbed water molecules.
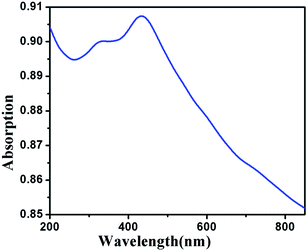 |
| Fig. 7 UV-visible spectrum of coordination polymer DMDT–Au. | |
It can be seen from Fig. 8 that the degradation of DMTD–Au starts at 260 °C, which may be due to the cleavage of the metal–sulphur and metal–nitrogen linkages, indicating good stability of the synthesized coordination polymer. The corresponding DTA plot exhibits four peaks at 280 °C, 600 °C, 510 °C and 730 °C.
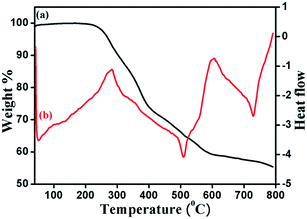 |
| Fig. 8 TGA (a) and DTA (b) plot of DMTD–Au. | |
Broido et al.39 reported a method for the evaluation of the activation energy related to decomposition. The equation developed for the calculation of activation energy (Ea) is as follows:
lnln(1/Y) = −(Ea/R)1/T + constant |
where
Y =
Wt −
W∞/
W0 −
W∞;
Y is the fraction of the initial molecules not yet decomposed;
Wt is the weight at time
t;
W∞ is weight at infinite time (= zero) and
W0 is the initial weight. A plot of lnln(1/
Y)
vs. 1/
T gives an excellent approximation to a straight line. The slope is related to activation energy. Activation energy using Broido model, as shown in Fig. SI-3,
† is measured as 25.20 kJ mol
−1 with an
R2 value of 0.984.
3.2. Electroanalysis of CPE/DMTD–Au modified electrode
The cyclic voltammetry of the DMTD–Au modified carbon paste electrode was examined in the presence of 0.02 M Fe(II)/Fe(III) redox couple in 0.1 M phosphate buffer solution (PBS) at pH 7. Three-electrode configuration consisting of the carbon paste electrode as the working electrode, Pt foil as the counter electrode and Ag/AgCl as the reference electrode was used for RS sensing, as shown in Fig. 9.
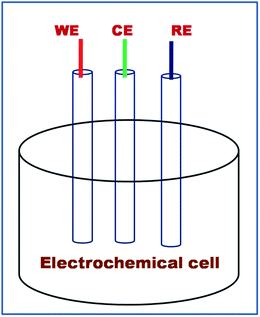 |
| Fig. 9 Schematic diagram of the three-electrode system. | |
A significant increase in the peak current of the Fe(II)/Fe(III) redox couple was observed with the DMTD–Au modified carbon paste electrode as compared to the unmodified carbon paste electrode for the same amount of Fe(II)/Fe(III) redox couple, as shown in Fig. 10, which reveals that the DMTD–Au polymeric material facilitates electron transfer and can be used as an excellent electrocatalytic material.
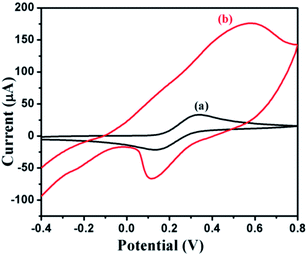 |
| Fig. 10 CV for 0.02 M Fe(II)/Fe(III) in 0.1 M PBS (pH 7) at the unmodified carbon paste electrode (a) and (DMTD–Au) modified carbon paste electrode (b). | |
3.3. Voltammetric sensing of resorcinol
The synthesized coordination polymer was further utilized for the voltammetric sensing of resorcinol using the DMTD–Au modified carbon paste electrode in phosphate buffer solution. For the voltammetric sensing of resorcinol, we selected a potential window region of 0 to 0.9 V because the oxidation of resorcinol is expected in this region. Resorcinol in PBS did not show any peak at the bare carbon paste electrode at trace concentration, and no peak of modified carbon paste electrode was observed, as shown in Fig. 11. Resorcinol addition shows an anodic peak at a potential of 0.55 V vs. Ag/AgCl over the modified electrode, as shown in Fig. 11. The electrochemistry responsible for this electro-sensing of resorcinol is probably because of the catalytic effect of DMTD–Au, which exhibits high electron transfer kinetics via the interaction of diazole nitrogen with the hydroxyl group of resorcinol through hydrogen bonding, as shown in the schematic representation in Fig. 12. The potential utility of the present modified electrode was further examined by recording the voltammetric response on the successive addition of resorcinol in PBS (pH 7.0). A systematic change in current was observed after each addition of resorcinol into the supporting electrolyte solution, as shown in the voltammogram of Fig. 13(a). This DMTD–Au modified carbon paste electrode does not suffer from electrode fouling and poisoning during resorcinol detection, which is a major limitation of other existing modified electrodes. The stability of the electrode is also very important for biosensing applications and was tested repeatedly for more than five times by the same modified carbon paste electrode. The calibration plot of resorcinol concentration vs. peak current is shown in Fig. 13(b) with the sensitivity and limit of detection of 0.019 μA nM−1 and 29.77 nM, respectively, at an R2 value of 0.999 and a S/N (signal-to-noise ratio) of 3.
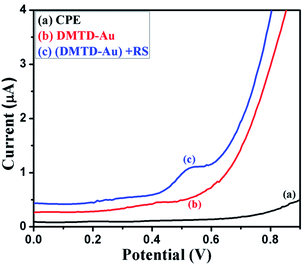 |
| Fig. 11 Differential pulse voltammetric response of unmodified carbon paste electrode (a), (DMTD–Au) modified carbon paste (b) and DMTD–Au modified carbon paste + RS (c) in phosphate buffer solution. | |
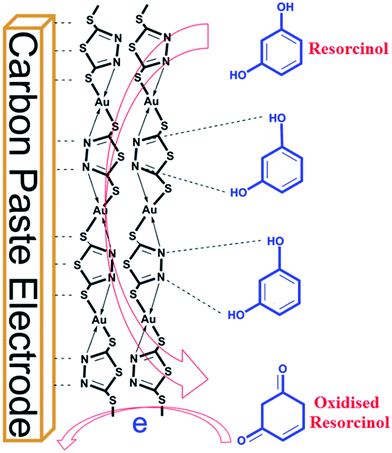 |
| Fig. 12 Schematic representation of the voltammetric sensing mechanism of resorcinol. | |
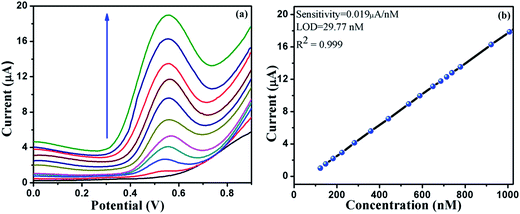 |
| Fig. 13 Differential pulse voltammogram of RS at various concentrations (112.60 × 10−9 to 1.09 × 10−6 M) in 0.1 M phosphate buffer (pH 7.0) at DMTD–Au modified carbon paste electrode (a) and the corresponding standard addition calibration plot of current vs. resorcinol concentration (b). | |
Conclusions
This work presents a one pot facile synthesis of coordination polymer DMDT–Au at room temperature. The synthetic method described here is very simple, does not require a catalyst to initiate polymerization, and can have commercial applications in drug delivery, metal–organic frameworks (MOFs) for gas storage, catalysis and sensing applications. The average molecular weight of DMDT–Au was found to be about 370
000 g mol−1 (PDI-2.32) and the activation energy of the synthesized material was evaluated using the Broido model to be 25.21 kJ mol−1 at an R2 value of 0.984. The DMDT–Au molecules are aligned parallel and polymeric layers are stacked due to π–π stacking and hydrophobic interaction. Furthermore, the redox activity of DMDT–Au was exploited for the electrochemical determination of resorcinol up to nanomolar concentration with modified carbon paste electrode. Under the optimized conditions, DMDT–Au modified carbon paste electrode (DMDT–Au/CPE) shows an anodic peak current at lower positive potential (0.55 V) than other existing methods and exhibits linear response towards resorcinol concentration with a sensitivity and limit of detection 0.019 μA nM−1 and 29.77 nM, respectively, at S/N (signal-to-noise ratio) of 3. The developed coordination polymer based modified electrode possesses advantageous properties, such as a high active surface area, stability and rapid electron transfer rate, which cumulatively demonstrate high performance towards the electrocatalytic oxidation and detection of resorcinol. Notable conclusions of the modified electrode surface demonstrate that DMDT–Au can be used to assemble a selective and sensitive sensor for resorcinol through the formation of intermolecular hydrogen bonding via a hetero atom. With respect to future research, we hope to broaden the scope for the synthesis of such thiadiazole–gold coordination polymers encapsulated with other valuable metal ions, and find their potential application towards chemical sensors, biomedical applications, biosensors and other electrochemical applications.
Acknowledgements
Madhu Tiwari and Sandeep Gupta are thankful to CSIR and UGC, respectively, for research fellowship. We are thankful to Prof. D. Pandey and Dr A. K. Singh, School of Materials Science &Technology IIT (BHU) for XRD facility and discussions, Prof. B. Ray, Chemistry, BHU for GPC of polymer, Prof. Ranjan Kumar, Physics, BHU for Raman, Prof. A. S. K. Sinha Chemical Engineering, IIT BHU for XPS measurements and Ashish Kumar, PhD, SMST for fruitful discussions and help.
References
- X. Huab and D. Yu, RSC Adv., 2012, 2, 6570–6575 Search PubMed.
- J. J. Wu, M. L. Cao, J. Y. Zhang and B. H. Ye, RSC Adv., 2012, 2, 12718–12723 RSC.
- M. Fang, L. Chang, X. Liu, B. Zhao, Y. Zuo and Z. Chen, Cryst. Growth Des., 2009, 9, 4006–4016 CAS.
- S. K. Ghosh, J. Ribas and P. K. Bharadwaj, CrystEngComm, 2004, 6, 250–256 RSC.
- D. R. Xiao, E. B. Wang, H. Y. An, Y. G. Li, Z. M. Su and C. Y. Sun, Chem.–Eur. J., 2006, 12, 6528–6541 CrossRef CAS PubMed.
- X. L. Wang, C. Qin and E. B. Wang, Cryst. Growth Des., 2006, 6, 439–443 CAS.
- E. J. Gao, M. Su, M. Zhang, Y. Huang, L. Wang, M. C. Zhu, L. Liu, Y. X. Zhang, M. J. Guo and F. Guan, Z. Anorg. Allg. Chem., 2010, 636, 1565–1569 CrossRef CAS.
- C. C. Wang, P. Wang and G. S. Guo, Transition Met. Chem., 2010, 35, 721–729 CrossRef CAS PubMed.
- L. X. Sun, Y. Qi, Y. M. Wang, Y. X. Che and J. M. Zheng, CrystEngComm, 2010, 12, 1540–1547 RSC.
- K. K. Bisht, A. C. Kathalikkattilab and E. Suresh, RSC Adv., 2012, 2, 8421–8428 RSC.
- L. F. Ma, J. H. Qin, L. Y. Wang and D. S. Li, RSC Adv., 2011, 1, 180–183 RSC.
- A. Carne, C. Carbonell, I. Imaz and D. Maspoch, Chem. Soc. Rev., 2011, 40, 291–305 RSC.
- H. Z. Xie and D. Y. Wei, Russ. J. Coord. Chem., 2011, 37, 600–605 CrossRef CAS.
- P. Kannan and S. A. John, Nanotechnology, 2008, 19, 1–10 CrossRef PubMed.
- J. Wang, J. N. Park, X. Y. Wei and C. W. Lee, Chem. Commun., 2003, 628–629 RSC.
- J. Peng and Z. N. Gao, Anal. Bioanal. Chem., 2006, 384, 1525–1532 CrossRef CAS PubMed.
- X. Hu, J. Li and J. Wang, Electrochem. Commun., 2012, 21, 73–76 CrossRef CAS PubMed.
- S. M. Mobin, B. J. Sanghavi, A. K. Srivastava, P. Mathur and G. K. Lahiri, Anal. Chem., 2010, 82, 5983–5992 CrossRef CAS PubMed.
- C. Kang, Y. Wang, R. Li, Y. Du, J. Li, B. Zhang, L. Zhou and Y. Du, Microchem. J., 2000, 64, 161–165 CrossRef CAS.
- L. Yang, Z. Wang and L. Xu, J. Chromatogr. A, 2006, 1104, 230–237 CrossRef CAS PubMed.
- A. Mirmohseni and A. Oladegaragoze, Sens. Actuators, B, 2004, 98, 28–36 CrossRef CAS PubMed.
- J. D. Wright, J. V. Oliver, R. J. M. Nolte, S. J. Holder, N. A. J. M. Sommerdijk and P. I. Nikitin, Sens. Actuators, B, 1998, 51, 305–310 CrossRef CAS.
- M. D. Olmo, A. Zafra, A. B. Jurado and J. L. Vilchez, Talanta, 2000, 50, 1141–1148 CrossRef.
- S. Gupta and R. Prakash, RSC Adv., 2014, 4, 7521–7527 RSC.
- S. Gupta, A. K. Singh, R. K. Jain, R. Chandra and R. Prakash, ChemElectroChem, 2014, 1, 793–798 CrossRef CAS.
- S. Gupta, M. Tiwari and R. Prakash, J. Nanosci. Nanotechnol., 2014, 14, 2786–2791 CrossRef CAS PubMed.
- S. A. A. Zaidi, A. S. Farooqi, D. K. Varshney, V. Islam and K. S. Siddiqi, J. Inorg. Nucl. Chem., 1977, 39, 581–583 CrossRef CAS.
- M. C. Bourg, A. Badia and R. B. Lennox, J. Phys. Chem. B, 2000, 104, 6562–6567 CrossRef CAS.
- Q. Li, C. Han, M. F. Cabrera, H. Terrones, B. G. Sumpter, W. Lu, J. Bernholc, J. Yi, Z. Gai, A. P. Baddorf, P. Maksymovych and M. Pan, ACS Nano, 2012, 6, 9267–9275 CrossRef CAS PubMed.
- N. G. Bastus, E. S.Tillo, S. Pujals, C. Farrera, C. Lopez, E. Giralt, A. Celada, J. Lloberas and V. Puntes, ACS Nano, 2009, 3, 1335–1344 CrossRef CAS PubMed.
- P. Cui, S. Seo, J. Lee, L. Wang, E. Lee, M. Min and H. Lee, ACS Nano, 2011, 5, 6826–6833 CrossRef CAS PubMed.
- T. Cai, M. Li, K. G. Neohand and E. T. Kang, J. Mater. Chem., 2012, 22, 16248–16258 RSC.
- N. Xu and B. H. Bo, Semicond. Sci. Technol., 2003, 18, 300–302 CrossRef CAS.
- X. Yang, M. Shi, L. Dong, Y. Ma, G. Ye and J. Xu, Polym. Degrad. Stab., 2010, 95, 2467–2473 CrossRef CAS PubMed.
- S. Soylemez, F. E. Kanik, A. Goyc, E. Nurioglu, H. Akpinarc and L. Toppare, Sens. Actuators, B, 2013, 182, 322–329 CrossRef CAS PubMed.
- X. Yang, M. Shi, L. Dong, Y. Maa, G. Ye and J. Xu, Polym. Degrad. Stab., 2010, 95, 2467–2473 CrossRef CAS PubMed.
- S. Yamaguchi, S. Nakagawa, S. Mitsul and S. Ohno, US Patent6340539 B1, 1999, pp. 1–6.
- R. J. Roberts, X. Li, T. F. Lacey, Z. Pan, H. H. Patterson and D. B. Leznoff, Dalton Trans., 2012, 41, 6992–6997 RSC.
- A. Broido, J. Polym. Sci., Part B: Polym. Phys., 1969, 7, 1761–1773 CrossRef CAS.
Footnote |
† Electronic supplementary information (ESI) available. See DOI: 10.1039/c4ra02983f |
|
This journal is © The Royal Society of Chemistry 2014 |
Click here to see how this site uses Cookies. View our privacy policy here.