DOI:
10.1039/C4RA04925J
(Paper)
RSC Adv., 2014,
4, 45939-45945
Synthesis and self-assembly of hierarchical SiO2–QDs@SiO2 nanostructures and their photoluminescence applications for fingerprint detection and cell imaging
Received
25th May 2014
, Accepted 18th August 2014
First published on 18th August 2014
Abstract
Hierarchical SiO2–QDs@SiO2 (QDs = CdTe, PbTe or PbS) nanostructures have been prepared by an integrated synthesis and self-assembly strategy using 3-mercaptopropionic acid (MPA) to stabilize the QDs and NaOH to control the self-assembly of QDs on the SiO2 nanobead carriers. 3–10 nm QDs covered with a 1–2 nm SiO2 shells were separately embedded on the surface of 50 nm SiO2 nanobead carriers, resulting in hierarchical SiO2–QDs@SiO2 nanostructures. MPA and NaOH played important roles in the nucleation and growth of QDs, etching the SiO2 nanobead carriers to the colloidal surface, and the self-assembly of the QDs on viscous SiO2 nanobead carriers. The hierarchical SiO2–QDs@SiO2 nanostructures possess various advantages: (I) the growth of QDs and their self-assembly on SiO2 nanobead carriers were performed in a one-pot reaction by a facile aqueous phase refluxing approach; (II) the SiO2 shell with 1–2 nm in thickness can prevent the leakage of toxic Cd2+ or Pb2+ and avoid photobleaching or environmental pollution; (III) the surface of hierarchical SiO2–QDs@SiO2 nanostructures can be easily functionalized through silane-coupling chemistry. Because of the excellent photoluminescence and biocompatibility, the hierarchical SiO2–QDs@SiO2 nanostructures modified by carboxyl (–COOH) active group can be available for covalent coupling to various biomolecules, which have shown potential applications in fingerprint detection and in vivo cell imaging.
1. Introduction
Because of the unique size-tunable, narrow emission and high photostability properties, semiconductor quantum dots (QDs) have been attracting extensive attention1–3 as light-emitting diode,4,5 vivo imaging,6,7 optoelectronic and photovoltaic devices.8–10 However, QDs are susceptible to external environment and easily lose their fluorescence due to optical and chemical instability in harsh environment. On the other hand, QDs generally contain highly toxic heavy metal ions, such as Cd2+, Pb2+, which cause environmental pollution.11,12 Up to now, a series of stable coatings, such as polymer and silica, have been developed to improve the chemical stability and biocompatibility of the QDs. Silica coating, as a popular inert material, can provide chemical/physical shielding from the direct environment and has good water dispensability.13–15 Moreover, the surface of QDs with silica coating can be easily modified by various functional groups through a silane-coupling method.16 For example, Rogach et al. encapsulated luminescent QDs into SiO2 particles by the Stöber method, and the QDs@SiO2 core–shell nanocomposites showed good biocompatibility and photostability for fluorescence imaging.17 Ma et al. encapsulated QDs in SiO2 nanoparticles via the reverse microemulsion methodology, and the obtained multiplexed colored silica–QDs nanohybrids could avoid the quenching of the photoluminescence (PL) of QDs.18 These coating methods for preparing QDs involved complicated steps and environmental polluting solvents, so exploring a simple green method to prepare QDs with high photostability still remains a challenge.
In this paper, an integrated synthesis and self-assembly strategy for preparing hierarchical SiO2–QDs@SiO2 (QDs = CdTe, PbTe or PbS) nanostructures in aqueous solution has been developed. The growth of QDs and self-assembly process were involved in this one-pot synthesis. The fabrication of hierarchical SiO2–QDs@SiO2 nanostructures, which includes the synthesis of 3–10 nm QDs and their self-assembly on the SiO2 nanobead carriers in a one-pot reaction. Fortunately, the hierarchical SiO2–QDs@SiO2 nanostructures can be successfully modified by –COOH or –NH2 active group by the silane-coupling agent, and then the functionalized hierarchical nanostructures (SiO2–QDs@SiO2–COOH or SiO2–QDs@SiO2–NH2) can be obtained. The as-prepared multicolored hierarchical SiO2–CdTe@SiO2–COOH nanostructures have strong binding affinity for the amino acids of the fingerprint by cross-coupling reaction, which can be available for visualizing the fingerprint images without background influence.19 On the other hand, the as-obtained multicolored hierarchical SiO2–CdTe@SiO2–COOH nanostructures show high PL fluorescence and good biocompatibility, which could be effective for the enhancement of cell bioimaging. The synthetic process of the SiO2–QDs@SiO2 nanostructure is schematic presented in Scheme 1.
 |
| Scheme 1 Synthetic procedures of the hierarchical SiO2–QDs@SiO2 nanostructure. | |
2. Experimental details
All the chemicals used in this work were of analytical grade and were used without further purification. The reactions were carried out under open-air conditions. Tetrabutyl titanate, cadmium chloride hydrate (CdCl2·2.5H2O, 99%), tetraethoxysilane (TEOS), triton X-100, succinic anhydride (95%), 1-hexanol (98%), ammonia, sodium tellurite (97%), hydrazine hydrate, toluene, N,N-dimethylformamide (DMF), (3-aminopropyl)-triethoxysilane AMEO (KH550), 3-mercaptopropionic acid (MPA, C3H6O2S, 99%), sodium sulphide, lead acetate and cyclohexane (98%) were purchased from Aladdin Chemistry Co., Shanghai, China.
The morphology of the hierarchical SiO2–QDs@SiO2 nanostructures were investigated by field emission scanning electron microscopy (FESEM, Hitachi S-4800, Japan) and transmission electron microscopy (TEM, JEM-2100, Japan). The elemental compositions of the SiO2–QDs@SiO2 were confirmed by spatially energy dispersive X-ray spectroscopy (EDS, Falcon, EDAX). The X-ray diffraction (XRD) pattern of the SiO2–CdTe@SiO2 was recorded on a Bruker AXS D8 Discover diffraction system with a Cu Kα-radiation source (λ = 1.5406 Å) at a scanning rate of 0.02° per second in the 2θ range of 10–60°. The FT-IR spectra of the surface functionalization of SiO2–CdTe@SiO2 were carried out using KBr tablets (1% w/w of product in KBr) at a resolution of 4 cm−1 and 100 scans per sample.
2.1 Synthesis of hierarchical SiO2–QDs@SiO2 (QDs = CdTe, PbTe or PbS) nanostructures
1. (i) Synthesis of hierarchical SiO2–CdTe@SiO2 nanostructures with PL emission from yellow to red. In a typical procedure, 0.1 g of the SiO2 nanobead carriers (prepared as the ref. 20) were added to a flask containing 48.0 mL of deionized water, 40 μL of MPA and 1.6 mL of 0.1 M CdCl2 solution. The pH of the solution was adjusted to 10.0 by 0.1 M of NaOH solution, and 8.0 mg of Na2TeO3 were added to the solution and stirred for 6 h at 80 °C. Subsequently 1.0 mL of 80% hydrazine hydrate was added to the solution. A series of hierarchical SiO2–CdTe@SiO2 nanostructures with different colors from yellow to dark red could be obtained by adjusting the period of the refluxing time.
(ii) Synthesis of hierarchical SiO2–CdTe@SiO2 nanostructures with green PL emission. The NaHTe, an intermediate product for preparation of CdTe QDs, was first synthesized by the reduction of tellurium powder with NaBH4.21 In a typical procedure, 45 mg of NaBH4, 16 mg of Te powder and 2 mL of deionized water were added to a flask, and then kept in the flask in 60 °C water bath for several minutes until the black Te powder disappeared. Then, the white sodium tetraborate (Na2B4O7) precipitate appeared on the bottom of the flask, and the flasket was then cooled by ice. The synthesis of hierarchical SiO2–CdTe@SiO2 nanostructures was as following: 30 mL of solution containing 84.5 mg of CdCl2, 60 μL of MPA and 0.1 g of SiO2 nanobead carriers were transferred into a flask, and the pH of the solution was adjusted to 10.0 by 0.1 M of NaOH solution and purged with N2 for 1 h. Subsequently, 1.2 mL of oxygen-free NaHTe aqueous solution was injected into the solution, and refluxed at 100 °C for 1 h. The orange products were centrifuged at 5000 rpm for 5 min and washed with ethanol and deionized water three times. Then, the hierarchical SiO2–CdTe@SiO2 nanostructures were obtained.
2. Synthesis of hierarchical SiO2–PbTe (PbS)@SiO2 nanostructures with the near-IR PL emission. The hierarchical SiO2–PbTe@SiO2 or SiO2–PbS@SiO2 nanostructures could be synthesized with a similar method by using (CH3COO)2Pb as the Pb source and NaTeO3 or Na2SO3 as the Te or S source. 0.1 g of the SiO2 nanobead carriers were added to a flask containing 48.0 mL of deionized water, 40 μL of MPA and 1.6 mL of 0.1 M (CH3COO)2Pb solution. The pH of the solution was adjusted to 10.0 by 0.1 M of NaOH solution, and 8.0 mg of Na2TeO3 was added to the solution and stirred for 6 h at 80 °C. Subsequently, 1.0 mL of 80% hydrazine hydrate was added to the solution. A series of hierarchical SiO2–PbTe (PbS)@SiO2 nanostructures with different sizes with the near-IR PL emission could be obtained by adjusting the period of the refluxing time.
2.2 Modification of the hierarchical SiO2–CdTe@SiO2 nanostructures by –COOH or –NH2 groups
As we know, the –OH group of Si–OH on the surface of SiO2–CdTe@SiO2 nanostructures can conjugate to other active groups through the silane-coupling reaction, and then –COOH or –NH2 functional group modified SiO2–CdTe@SiO2 nanostructures can be obtained.
(i) Modification of the hierarchical SiO2–CdTe@SiO2 nanostructures by –COOH group: typically, 0.1 g of succinic anhydride, 25 mL of N,N-dimethylformamide (DMF) and 235 μL of KH550 were transferred to a flask, and kept at 70 °C for 3 h to obtain the –COOH group conjugated KH550 (KH550-COOH). Subsequently, 0.1 g of the hierarchical SiO2–CdTe@SiO2 nanostructures was added into the KH550-COOH solution to obtain the hierarchical SiO2–CdTe@SiO2–COOH nanostructures. Finally, the resulting products were centrifuged at 5000 rpm for 5 min and washed with ethanol and water three times. The samples were redispersed in deionized water as a stock solution.
(ii) Modification of the hierarchical SiO2–CdTe@SiO2 nanostructures by –NH2 group: typically, 0.1 g of the hierarchical SiO2–CdTe@SiO2 nanostructures, 10.0 mL of toluene and 700 μL of KH550 were transferred to a flask, and refluxed at 80 °C for 12 h to obtain the hierarchical SiO2–CdTe@SiO2–NH2 nanostructures. Finally, the resulting products were centrifuged at 5000 rpm for 5 min and washed with ethanol and deionized water for three times. The samples were redispersed in deionized water as a stock solution.
2.3 Fingerprint detecting test
Fingerprints were deposited by pressing a finger onto a clean glass surface, and the solution of SiO2–CdTe@SiO2 that containing carboxyl was painted on the glass uniformly and air drying for two hours. After washing with deionized water, the glass substrate was placed under the UV light.
2.4 Cell imaging experiment
The potential bioimaging application of hierarchical SiO2–CdTe@SiO2–COOH nanostructures were carried out on HeLa cells. First, the HeLa cells were grown in Dulbecco's Modified Eagle Medium supplemented with 5% fetal bovine serum and 1% antibiotics at 37 °C and 5% CO2, seeded in 96-well tissue culture plates and allowed to adhere for 5 h. Subsequently, after washing with phosphate-buffered saline solution, HeLa cells were incubated in a serum-free medium containing hierarchical SiO2–CdTe@SiO2–COOH nanostructures at 37 °C for 3 h under 5% CO2.
2.5 MTT assay
HeLa cells were cultured in Dulbecco's Modified Eagles Medium (DMEM) supplemented with 10% fetal bovine serum (FBS) and antibiotics (100 U mL−1 penicillin, 100 mg mL−1 streptomycin). The SiO2, SiO2–QDs@SiO2 and QDs solutions with culture medium containing HeLa cells were cultured in an humidified incubator with 5% CO2 at 37 °C. Cell viability was evaluated by 3-(4,5-dimethylthiazol-2-yl)-2,5-diphenyl tetrazolium bromide (MTT; Sigma) reduction conversion assay and cell survival was expressed as absorbance at 570 nm in a spectrophotometric microplate reader (Bio-RAD Model 680, USA).
3. Results and discussion
3.1 Morphology and structures
Fig. 1 shows the SEM images of the as-prepared SiO2 nanobead carriers, hierarchical SiO2–CdTe@SiO2, SiO2–PbTe@SiO2, SiO2–PbS@SiO2, SiO2–CdTe@SiO2–COOH and SiO2–CdTe@SiO2–NH2 nanostructures. The as-prepared SiO2 nanobead carriers are generally spherical in shape and uniform (as shown in Fig. 1a). The morphology of hierarchical SiO2–CdTe@SiO2 nanostructures shows that the small CdTe QDs are coated onto the surface of SiO2 nanobead carriers (as shown in Fig. 1b). The corresponding EDAX spectrum demonstrates the presence of Cd, Te, Si and O element in the final products (as shown in the inset one of Fig. 1b). Hierarchical SiO2–PbTe@SiO2 and SiO2–PbS@SiO2 nanostructures can be successfully synthesized when (CH3COO)2Pb is used as the Pb source and NaTeO3 (Na2SO3) is used as the Te (S) source. The hierarchical SiO2–PbTe@SiO2 and SiO2–PbS@SiO2 nanostructures show similar morphology as that of SiO2–CdTe@SiO2 nanostructures (as shown in Fig. 1c and d). The chemical properties of the surface of the nanostructures can be modified through the conjugation of active groups, and the SEM images confirmed that the morphology of hierarchical SiO2–CdTe@SiO2–COOH and SiO2–CdTe@SiO2–NH2 still remains as the original (as shown in Fig. 1e and f).
 |
| Fig. 1 SEM images of (a) SiO2, (b) SiO2–CdTe@SiO2, (c) SiO2–PbTe@SiO2, (d) SiO2–PbS@SiO2, (e) SiO2–CdTe@SiO2–COOH, and (f) SiO2–CdTe@SiO2–NH2. Insets of Fig. 1b–d show the corresponding EDAX of hierarchical SiO2–CdTe@SiO2, SiO2–PbTe@SiO2 and SiO2–PbS@SiO2 nanostructures. | |
HRTEM characterization was applied to investigate the structure and morphology of the QDs of the hierarchical SiO2–QDs@SiO2 nanostructures. TEM images of QDs depict that 3–10 nm QDs covered by a 1–2 nm SiO2 shells were embedded onto the surface of the SiO2 nanobead carriers (as shown in Fig. 2). The uniform CdTe QDs of about 5 nm in diameter deposited onto the surface of SiO2 nanobead carriers with approximately 50 nm in diameter were separated well (as shown in Fig. 2a). The HRTEM image of a CdTe QDs shows that the QDs are well-crystallized, and the lattice fringe with a spacing of approximately 0.34 nm corresponds to the interplanar distance of the (200) plane in the cubic CdTe (JCPDS Card no. 65-1046) (as shown in Fig. 2b). The PbTe QDs with 7 nm in diameter were separated well onto the surface of SiO2 nanobead carriers (as shown in Fig. 2c). The PbTe QDs covered by a 2 nm SiO2 shell were embedded on the surface of SiO2 nanobead carriers (as shown in Fig. 2d). In addition, the HRTEM image of PbTe QDs shows that the d value of a PbTe QDs is about 0.33 nm, which can be assigned to the (200) reflection of cubic PbTe (as shown in Fig. 2d). Similarly, the PbS QDs with a diameter of about 6 nm are deposited separately onto the surface of SiO2 nanobead carriers (as shown in Fig. 2e). HRTEM image of the hierarchical SiO2–PbS@SiO2 nanostructures shows that d value of the PbS QDs is approximately 0.32 nm, which corresponds to the (111) plane of cubic PbS (as shown in Fig. 2f).
 |
| Fig. 2 TEM images of hierarchical SiO2–CdTe@SiO2 (a and b), SiO2–PbTe@SiO2 (c and d), and SiO2–PbS@SiO2 (e and f) nanostructures. The inserts of Fig. 2b, d and f show the representative HRTEM image. | |
The growth of QDs and the self-assembly of QDs and SiO2 nanobead carriers are two important steps toward generating the advanced hierarchical SiO2–QDs@SiO2 nanostructures. In the synthesis process, MPA and NaOH play important roles in the synthesis and self-assembly strategy and the formation of the hierarchical SiO2–QDs@SiO2 nanostructures. MPA, acting as the stabilizer, is a superior capping molecule for producing stable QDs, and the carboxylic groups of MPA may coordinate with the Cd or Pb site of monomers or clusters for the fast growth of QDs nanocrystal. On the other hand, NaOH is another agent influencing the formation of hierarchical SiO2–QDs@SiO2 nanostructures.22 NaOH can favor PL enhancement in the colloid solution by decreasing the defects attributed to the formation of Cd–thiol (Cd(SR)) complexes on CdTe QDs surfaces. Furthermore, NaOH can assist the self-assembly of QDs on the surface of SiO2 nanobead carriers, which include the following sequence: from (i) etching the surface of SiO2 nanobead carriers by NaOH solution to obtain the colloidal surface to; (ii) adhering QDs on the viscous surface of SiO2 nanobead carriers to; (iii) covering the QDs by a colloidal SiO2 shell and then (iv) forming the hierarchical SiO2–QDs@SiO2 nanostructures.
X-ray diffraction analysis was applied to investigate the CdTe crystalline of hierarchical SiO2–CdTe@SiO2 nanostructures. Three broad peaks at about 24.5°, 40.5° and 48° correspond to the (111), (220), and (311) planes, respectively, which are matched with the cubic zinc blende structure of CdTe crystal (as shown in Fig. 3). According to the XRD data, the average QDs size can be quantitatively evaluated using the Debye–Scherrer formula, which gives the relationship between peak broadening in the XRD and particle size:
D = 0.89λ/(Bsize cos θB) |
where 0.89 is the Debye–Scherrer constant,
λ represents the X-ray wavelength (
λ = 1.5406 Å),
Bsize is the full width at half-maximum and
θB is the Bragg diffraction angle.
23 The nanoparticle size of CdTe QDs determined by the Debye–Scherrer equation is about 4.5 nm, which corresponded to the information obtained from the HRTEM image of CdTe QDs (as shown in
Fig. 2a).
 |
| Fig. 3 XRD patterns of the hierarchical SiO2–CdTe@SiO2 nanostructures. | |
3.2 Characterization of surface functionalization
As we know, most applications of QDs are directly dependent on the chemistries that make them hydrophilic and biofunctional. The hierarchical SiO2–CdTe@SiO2–COOH or SiO2–CdTe@SiO2–NH2 nanostructures provide a chemical link with various functional molecules for promising applications. In this paper, the hierarchical SiO2–CdTe@SiO2–COOH nanostructures can pave the way for applying them in the fields of imaging in vivo cells and detecting fingerprints. FT-IR spectra are applied to confirm the surface binding of the capping groups. The IR spectra of the samples confirmed that –COOH or –NH2 groups were successfully connected to the hierarchical SiO2–CdTe@SiO2 nanostructures. The FT-IR spectra of hierarchical SiO2–CdTe@SiO2–COOH confirm the successful functionalization of hierarchical SiO2–CdTe@SiO2 in the presence of carbonyl group (–CO–, νC
O ≈ 1565 cm−1) and hydroxyl group (–OH, νO–H ≈ 3448 cm−1),24 as shown in Fig. 4c. In addition, FT-IR spectra confirm that the amino group (–NH2) is also successfully connected to the hierarchical SiO2–CdTe@SiO2 in the presence of peaks at about 1470, 2580 and 2924 cm−1 (ref. 25) (as shown in Fig. 4d). Two distinct peaks for the SiO2 nanobead carriers at about 800 and 1100 cm−1 are attributed to the symmetric contraction vibration peak, horizontal and vertical to the Si–O–Si bond. The presence of a peak at 1385 cm−1 is due to the interaction of the samples with KBr in the tablets, and the band around 1635 cm−1 is assigned to the bending mode of the interlayer water molecule.26
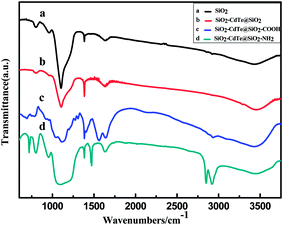 |
| Fig. 4 FT-IR spectra of (a) SiO2, (b) SiO2–CdTe@SiO2, (c) SiO2–CdTe@SiO2–COOH and (d) SiO2–CdTe@SiO2–NH2 (samples a–d). | |
3.3 Optical properties of the hierarchical SiO2–CdTe@SiO2 nanostructures
It is well-known that the PL wavelength range of QDs can be finely tuned by changing the size of the QDs particles.27,28 In this paper, hierarchical SiO2–CdTe@SiO2 nanostructures show excellent luminescence properties, and the PL colors can be tuned from green to red in the visible spectrum. Fig. 5 shows the PL spectra, normalized UV-vis absorption spectra and corresponding photographs of the solution of hierarchical SiO2–CdTe@SiO2 nanostructures. The PL emission peaks of CdTe QDs range from 535 nm to 640 nm, which reveals that the tunable emission spectrum is in the visible range (as shown in Fig. 5a). The size-dependent quantum confinement effects are apparent during the CdTe QDs growth process because solutions in vials shift across the visible spectrum from green to red emission (as shown in inset of Fig. 5b). The corresponding peak position in the UV-vis spectra ranges from 460 nm to 610 nm, attributed to the increase in the non-radiative recombination of electrons and holes on the surface of the CdTe QDs as the size increases.29 During the growth of CdTe QDs, smaller particles dissolve and become the constituents of larger QDs, a process known as Ostwald ripening. The tunable colors of the hierarchical SiO2–CdTe@SiO2 nanostructures implies that our facile method can control the size of CdTe QDs from 2.6 nm to 5.0 nm, and thus the PL emission changes from the green to the red region of the visible spectrum. Interestingly, the hierarchical SiO2–CdTe@SiO2 nanostructures still remain their PL properties after the modification by –COOH or –NH2 group. The SiO2 coating prevents the oxidative and photothermal degradation of the QDs and increases the stability of QDs@SiO2 nanostructures. The spectra of SiO2–CdTe@SiO2 nanostructures exhibit only a slight red shift (∼25 meV) over 30 days of water soaking and almost zero peak broadening for 60 days in air.
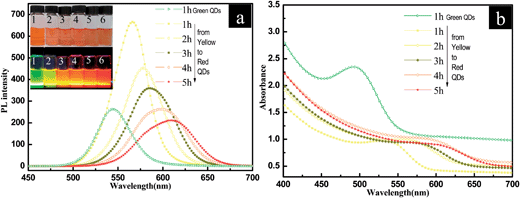 |
| Fig. 5 Emission (a) and absorption (b) spectra of hierarchical SiO2–CdTe@SiO2 nanostructures in aqueous solution (excitation wave length: 380 nm), colloidal solutions of hierarchical SiO2–CdTe@SiO2 nanostructures (left to right, inserts of Fig. 4b) consisting of samples 1–6 obtained by the aforementioned methods under normal and UV light (352 nm, 6 W). | |
4. Fingerprint detecting
The pattern of friction ridge skin on the finger of the hands is unique to each individual, which can provide significant information about one person.30–32 Usually, ninhydrin solution, iodine/benzoflavone spray33 and vacuum metal deposition34 techniques have been developed to detect fingerprint. However, these methods suffer from influence by complex background. QDs can luminesce with greater intensity and higher photostability than commercially available organic fluorescence dyes, and so the QDs are the potentially ideal reagents for detecting the latent fingerprint deposited on polychrome substance. For instance, Sametband et al. have shown that CdSe/ZnS QDs stabilized with n-alkaneamines in organic solution can be used for the direct visualization of latent fingerprint on wet nonporous surfaces.35 CdS/dendrimer in organic solvent has also been used for the detection of fingerprint.36,37 However, the toxicity of QDs without protecting shell may hinder their applications in the fields of fingerprint detection. Fortunately, the hierarchical SiO2–CdTe@SiO2–COOH nanostructures demonstrate forensic work on fingerprint detection.
Hierarchical SiO2–CdTe@SiO2–COOH nanostructures can interact with the amino acid of a fingerprint, especially the sebum-rich fingerprint, and acts as a dusting powder to locate and visualize fingerprints under UV light.38 Because the emission of the multicolored CdTe QDs in aqueous solution under UV light covers almost the visible region from green to red, the fingerprint deposited on objects can be readily detected without being influenced by background colors. A fingerprint on glass was detected by the hierarchical SiO2–CdTe@SiO2–COOH nanostructures, and the fluorescence photographs are shown in the Fig. 6. The –COOH groups modified on the hierarchical SiO2–CdTe@SiO2–COOH nanostructures has a strong affinity for amino acid or grease in the substances of the fingerprint, which enables the visualization of the fingerprint, even if the prints are faint.
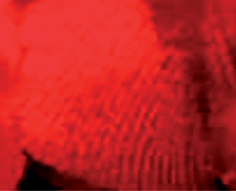 |
| Fig. 6 Photographs of the detection of a fingerprint deposited on a glass under UV light (352 nm, 6 W). | |
5. Cells imaging
The free carboxyl (–COOH) group conjugated on the surface of hierarchical SiO2–CdTe@SiO2–COOH nanostructures is available for covalent coupling to various biomolecules (such as proteins, peptides and nucleic acids) by cross-linking to reactive amine (–NH2) groups of the vivo cells. The luminescence images of HeLa cells are shown in Fig. 7. Bright spots are attributed to the CdTe QDs of the hierarchical SiO2–CdTe@SiO2–COOH nanostructures, which can illuminate the entire image of the cell (as shown in Fig. 7a and c). The cell image could also be demonstrated by the dark frame (Fig. 7b). These bright spots are mainly distributed in the membrane of HeLa cells. The shape and position of the HeLa cells (as shown in Fig. 7a and c) indicate a strong interaction between HeLa cells and hierarchical SiO2–CdTe@SiO2–COOH nanostructures by forming covalent bonds. It is obvious that the hierarchical SiO2–CdTe@SiO2–COOH nanostructures did not permeate the cytomembrane, rendering the hierarchical SiO2–CdTe@SiO2–COOH nanostructures suitable for cell-labeling markers.
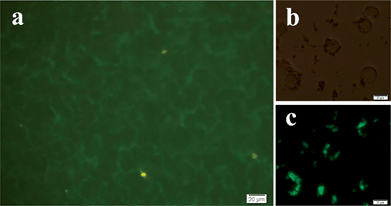 |
| Fig. 7 Fluorescence imaging of HeLa cells after incubation with hierarchical SiO2–CdTe@SiO2–COOH nanostructures (green-emitting nanocrystals). The acquisition was performed using a continuous-wave laser source emitting at 380 nm. | |
The quantity of the living cells was reflected by MTT assays (Fig. 8). The MTT results reveal that SiO2 shell increased cellular proliferation without any evidence of cytotoxicity and the viability of HeLa cells were similar to the results of the SiO2 control test, while the QDs without SiO2 shell had obvious cytotoxicity. After 7 days of culturing, the number of living cells declined dramatically.
 |
| Fig. 8 Comparison of the proliferation of Hela cells cultured with SiO2, SiO2–QDs@SiO2 and QDs solutions after 1, 3, 5 and 7 days of culture, respectively. | |
6. Conclusion
In general, an integrated synthesis and self-assembly strategy for preparing hierarchical SiO2–QDs@SiO2 nanostructures (QDs = CdTe, PbTe or PbS) has been developed. In the synthesis, 3–10 nm QDs covered by 1–2 nm SiO2 shells were deposited onto the surface of SiO2 nanobead carriers, resulting in the hierarchical SiO2–QDs@SiO2 nanostructures. The color-tunable hierarchical SiO2–QDs@SiO2 nanostructures exhibit excellent optical property with PL emission from green (CdTe) to the near-IR (PbS and PbTe) of the region. Moreover, the hierarchical SiO2–QDs@SiO2 nanostructures can be readily introduced to –COOH or –NH2 active group. The hierarchical SiO2–QDs@SiO2–COOH nanostructures are available for covalent coupling to various bimolecular by the cross-linking reaction, with the result that the SiO2–QDs@SiO2 nanostructures show promising potential applications in detecting fingerprint and imaging cells. This work sheds new light on the preparation of hierarchical SiO2–QDs@SiO2 nanostructures (QDs = CdTe, PbTe or PbS) and their applications in cell imaging and fingerprint detection without being influenced by the multicolored environment.
Acknowledgements
This work was supported by the National Natural Science Foundation of China (nos 51272235, 21301155); Zhejiang Provincial Natural Science Foundation of China (LR12E02001); Program for New Century Excellent Talents in University (NCET-13-0998); Program for Changjiang Scholars and Innovative Research Team in University (PCSIRT-13097) and Open Project Program of State Key Laboratory of Inorganic Synthesis and Preparative Chemistry, Jilin University.
References
- M. Bruchez, M. Moronne, P. Cin, S. Weiss and A. P. Alivisatos, Science, 1998, 281, 2013–2016 CrossRef CAS.
- B. Dubertret, P. Skourides, D. J. Norris, V. Noireaux, A. H. Brivanlou and A. Libchaber, Science, 2002, 298, 1759–1762 CrossRef CAS PubMed.
- H. B. Li, Y. L. Li and J. Cheng, Chem. Mater., 2010, 22, 2451–2457 CrossRef CAS.
- J. L. Zhao, J. Y. Zhang, C. Y. Jiang, J. Bohnenberger, T. Basché and A. J. Mews, J. Appl. Phys., 2004, 96, 3206–3210 CrossRef CAS PubMed.
- S. Nizamoglu, T. Ozel, E. Sari and H. V. Demir, Nanotechnology, 2007, 18, 065709 CrossRef.
- H. Mattoussi, J. M. Mauro, E. R. Goldman, G. P. Anderson, V. C. Sundar, F. V. Mikulec and M. G. Bawendi, J. Am. Chem. Soc., 2000, 122, 12142–12150 CrossRef CAS.
- P. Alivisatos, Nat. Biotechnol., 2004, 22, 47–52 CrossRef CAS PubMed.
- Y. Jun, J. H. Lee and J. Cheon, Angew. Chem., Int. Ed., 2008, 47, 5122–5135 CrossRef CAS PubMed.
- M. Rosso, A. Arafat, K. Schron, M. Giesbers and H. Zuilhof, Langmuir, 2008, 24, 4007–4012 CrossRef CAS PubMed.
- E. M. Barea, M. Shalom, S. Giménez, I. Hod, I. Mora-Seró, A. Zaban and J. Bisquert, J. Am. Chem. Soc., 2010, 132, 6834–6389 CrossRef CAS PubMed.
- M. Darbandi, R. Thomann and T. Nann, Chem. Mater., 2005, 17, 5720–5725 CrossRef CAS.
- R. Koole, M. M. Schooneveld, J. Hilhorst, K. Castermans, D. P. Cormode, G. J. Strijkers, C. M. Donega, D. Vanmaekelbergh and A. W. Grifioen, Bioconjugate Chem., 2008, 19, 2471–2479 CrossRef CAS PubMed.
- D. K. Yi, T. Selvan, S. S. Lee, G. C. Papaefthymiou, D. Kundaliya and J. Y. Ying, J. Am. Chem. Soc., 2005, 127, 4990–4991 CrossRef CAS PubMed.
- T. T. Tan, S. Selvan, T. L. Zhao, S. J. Gao and J. Y. Ying, Chem. Mater., 2007, 19, 3112–3117 CrossRef CAS.
- M. A. Correa-Duarte, M. Giersig and L. M. Liz-Marzan, Chem. Phys. Lett., 1998, 286, 497–501 CrossRef CAS.
- Y. Yang and M. Y. Gao, Adv. Mater., 2005, 17, 2354–2357 CrossRef CAS.
- A. L. Rogach, D. Nagesha, J. W. Ostrander, M. Giersig and N. A. Kotov, Chem. Mater., 2000, 12, 2676–2685 CrossRef CAS.
- Q. Ma, I. C. Serrano and E. Palomares, Chem. Commun., 2011, 47, 7071–7073 RSC.
- G. Konstantatos, I. Howard, A. Fischer, S. Hoogland, J. Clifford, E. Klem, L. Levina and E. H. Sargent, Nature, 2006, 442, 180–183 CrossRef CAS PubMed.
- F. Rowell, K. Hudson and J. Seviour, Analyst, 2009, 134, 701–707 RSC.
- R. N. Sanoj, P. C. Krishna, T. Hiroshi, V. N. Shantikumar and J. Rangasamy, ACS Appl. Mater. Interfaces, 2011, 3, 3654–3665 Search PubMed.
- J. L. Duan, L. X. Song and J. H. Zhan, Nano Res., 2009, 1, 61–68 CrossRef.
- H. P. Klug and L. E. Alexander, X-ray diffraction procedures for polycrystalline and amorphous materials, John Wiley & Sons New York, 2nd edition, 1974, p. 716 Search PubMed.
- K. Liu and L. Jiang, Nano Today, 2011, 2, 155–175 CrossRef PubMed.
- L. Li, H. F. Qian, N. H. Fang and J. C. Ren, J. Lumin., 2006, 116, 59–66 CrossRef CAS PubMed.
- W. R. Algar and K. Susumu, Anal. Chem., 2011, 83, 8826–8837 CrossRef CAS PubMed.
- X. G. Peng and A. P. Alivisatos, Nature., 2000, 404, 59–61 CrossRef CAS PubMed.
- R. G. Xie, X. H. Zhong and T. Basché, Adv. Mater., 2005, 12, 2741–2745 CrossRef.
- R. G. Xie, U. Kolb, J. X. Li, T. Basché and A. Mews, J. Am. Chem. Soc., 2005, 127, 7480–7488 CrossRef CAS PubMed.
- M. Rajamathi and P. V. Kamath, J. Power Sources, 1998, 70, 118–121 CrossRef CAS.
- J. R. Millette, R. S. Brown and W. B. Hill, Nature, 2008, 18, 20–30 CAS.
- S. Oden and B. V. Hofsten, Nature, 1954, 173, 449–450 CrossRef CAS.
- K. Karu and A. K. Jain, Pattern Recognit., 1996, 29, 389–404 CrossRef.
- P. Theys, Y. Turgis, A. Lepareux, G. Chevet and P. F. Ceccaldi, Int. Crim. Police Rev., 1968, 217, 106–108 Search PubMed.
- M. Sametband, I. Shweky, U. Banin, D. Mandler and J. Almog, Chem. Commun., 2007, 0, 1142–1144 RSC.
- G. Carrot, D. Rutot-Houze, A. Pottier, P. Degee, J. Hilborn and P. Dubois, Macromolecules, 2002, 35, 8400–8404 CrossRef CAS.
- L. Zhou, C. Gao, W. J. Xu, X. Wang and Y. H. Xu, Biomacromolecules, 2009, 10, 1865–1874 CrossRef CAS PubMed.
- B. J. Theaker, K. E. Hudson and F. J. Rowell, Forensic Sci. Int., 2008, 174, 26–34 CrossRef CAS PubMed.
|
This journal is © The Royal Society of Chemistry 2014 |
Click here to see how this site uses Cookies. View our privacy policy here.