DOI:
10.1039/C4RA06793B
(Paper)
RSC Adv., 2014,
4, 43921-43930
Use of hybrid materials in the decontamination of bisphenol A from aqueous solutions
Received
8th July 2014
, Accepted 2nd September 2014
First published on 2nd September 2014
Abstract
Hybrid materials were obtained by modifying natural sericite. The sericite was modified with hexadecyltrimethylammonium bromide (HS) and alkyldimethylbenzylammonium chloride (AS) to obtain organo-modified sericites, together with aluminum-H-sericite (AHS) and aluminum-A-sericite (AAS) as inorgano–organo-modified sericite hybrid materials. The hybrid materials were characterized by FT-IR spectrometry, XRD and SEM. The pHPZC values of the solid hybrid materials were obtained by acid–base titration. The Brunauer–Emmett–Teller surface area and pore size of the hybrid materials were also determined. The resulting materials were used in the remediation of waste waters contaminated with bisphenol A (BPA) using batch and column reactor operations. The batch experiments showed that an increase in pH from 2.0 to 10.0 did not affect the percentage uptake of BPA by these hybrid materials, but at pH > 10.0 the percentage uptake of BPA decreased significantly for the AHS and AAS hybrid materials. An increase in the concentration of BPA (1.0–20 mg L−1) and the background electrolyte concentration (0.0001–0.1 mol L−1 NaCl) did not affect the percentage removal of BPA by these hybrid materials. The time dependence adsorption data showed that BPA was quickly taken up onto these hybrid materials as most of the BPA was trapped within the first few minutes of contact. The kinetic data fitted well to a pseudo-second-order and fractal-like pseudo-second-order model rather a pseudo-first-order model. The results of pseudo-second-order fitting showed that the removal capacities of the AS, HS, AAS and AHS hybrid materials for BPA were 4.816, 5.047, 4.874 and 4.856 mg g−1, respectively. In addition, AAS and AHS were used for dynamic studies in column experiments. The breakthrough curves were then used for the non-linear fitting of the Thomas equation. The loading capacity of the column for BPA using the AHS and AAS hybrid materials was estimated.
1. Introduction
Endocrine-disrupting chemicals can disturb the human nervous and endocrine systems and weaken the immune and reproductive systems by mimicking biological activity and reproductive hormones.1,2 Bisphenol A (BPA) is an important micro-pollutant and a potential endocrine disrupter. BPA mimics the female estrogen 17-β-estradiol. BPA can alter the normal hormonal system of animals and has various biochemical effects, e.g. morphological alterations and interference in sex differentiation; it can also reduce fertility.3,4 BPA has been shown to cause the proliferation of MCF-7 human breast cancer cells5 and exert a mutagenic action on human RSa cells.6 Several other in vitro and in vivo assays have confirmed that BPA may increase infertility, genital tract abnormalities and even breast cancer.7,8
BPA is widely used as a monomer in the synthesis of polycarbonate plastics and the epoxy resins used as dental sealants and liners in food packaging, flame retardants and other speciality products.9–11 These products contain minute amounts of unreacted BPA, which may leach from the materials and enter the aquatic environment and biosphere.12,13 The maximum concentration of BPA detected in leachates from landfill sites is 17.2 mg L−1.14 Significant levels of BPA have been reported in urine samples.11,15–17 The European Commission Scientific Committee on Food recommended a tolerable daily intake of 0.05 mg kg−1 body weight per day for BPA in the context of food stored in contact with plastics.18 Similarly, the United States Environmental Protection Agency recommended a safety level of a maximum acceptable or reference dose of 0.05 mg kg−1 body weight/day.19,20
Various methods, including physical,21 chemical22 and biological23 techniques, have been used to treat waters contaminated with BPA. An effective way to remove BPA from waste water is via adsorption onto various adsorptive materials. However, the moderately hydrophobic nature of BPA (octanol–water partition coefficient Kow = 103.4), which has a low polarity due to the presence of only two hydroxyl groups,24 has restricted the use of several adsorbent materials in the treatment of waters contaminated with BPA. Carbonaceous materials have been found to be effective for the removal of BPA.25 However, the uptake of BPA is reduced with an increase in temperature or ionic strength. Various minerals, activated carbon precursors and even coconut-based biomass have been studied and the results of these experiments suggest that activated carbon has a significantly higher capacity than minerals to remove BPA from aqueous solutions.26 Waters from various sources contaminated with endocrine-disrupting chemicals were treated with single- and multi-walled carbon nanotubes in addition to powdered activated carbon.27 A mesoporous carbon sample with a high specific surface area (920 m2 g−1) was obtained with hexagonal SBA-15 mesoporous silica and was used in the rapid and efficient removal of BPA. The sorption followed a pseudo-second-order kinetic model and was unaffected in the pH range 3–9.28
The green algae Monoraphidium braunii has been studied for the remediation of BPA in the presence and absence of natural organic matter (NOM) and the results showed that NOM did not affect the active uptake of BPA by this algae.3 However, when single-walled carbon nanotube ultra-filtration membranes were assessed for the attenuation of BSA and 17-β-estradiol in the presence of NOM, it was shown that NOM fouls the membrane by blocking the pores and forming a cake or gel, which ultimately decreases the transport of the endocrine-disrupting chemicals.29 Multi-walled carbon nanotubes showed a very high uptake capacity for BPA in aqueous wastes and hydrogen bonding and hydrophobic action are the leading mechanisms involved in the attenuation of BPA by this solid.30 Natural dead biomass (e.g. peat, rice husks, bagasse and sawdust) and hexadecyltrimethylammonium bromide (HDTMA)-modified peat have been used to remove BPA from aqueous solutions by passive adsorption. The results indicate that modified peat is an effective adsorbent material in the removal of BPA and a hydrophobic interaction between the solid/solution interface was proposed.31,32
BPA immobilized on granular activated carbon was exposed to a dielectric barrier discharge plasma to decompose the BPA in an integrated treatment process.33 A molecular level simulation was performed using the molecular mechanics Poisson–Boltzmann surface area approach for the removal of BPA and 17-α-ethinyl estradiol by adsorption onto graphene, single-walled carbon nanotubes and multi-walled carbon nanotubes. The simulation results were consistent with the experimental findings and an increase in ionic strength did not significantly affect the immobilization of BPA and 17-α-ethinyl estradiol.34
Clay minerals (phyllosilicates) are composed, with a few exceptions, of crystal structures consisting of sheets packed firmly in structural layers. The individual structural layers consist of two, three or four sheets. The sheets are formed from tetrahedral [SiO4]4− (T) and octahedral [AlO3(OH)3]6− (O). The interiors of the tetrahedrons and octahedrons contain smaller metal cations and their apices are occupied by oxygen atoms, some of which are connected to protons to form –OH. These fundamental structural elements are arranged to form a hexagonal network within each sheet. Depending on the number and ratio of the sheets in the fundamental structural unit, the substitution of cations within the octahedrons and tetrahedrons determines the charge in these layers. Silicate layers have a tendency to organize themselves to form stacks with a regular van der Waals gap between them: an ‘interlayer’. The electrostatic negativity of the structural layer is naturally compensated by cations within this interlayer space. The most common exchangeable cations are K+, Na+, Ca2+, Mg2+ and H+. A simple treatment of the clay minerals allows any of these cations to be exchanged with another cation. The removal of ions from the octahedral or tetrahedral sheets is energetically more demanding and produces a material with a new microstructure and larger pore volumes.35–37 The electrical charge, the micro- to meso-level porosity, the available surface functional groups and the presence of exchangeable cations within the interlayer space make clay minerals useful natural adsorbents that can be used for the removal/attenuation of several toxic heavy metal ions from aqueous solutions. However, these materials have a low selectivity towards, and low removal capacity for, several non-polar or less polar organic contaminants. Clay minerals can, however, be modified with an appropriate organic surfactant molecule or even a pillared inorgano–organo-clay to make them effective materials for the attenuation of organic impurities.38–40 This modification makes the clay structure more organophilic and enhances the hydrophobicity.35,41,42 Dodecyltrimethylammonium bromide and HDTMA surfactants have been used in a sequential treatment to modify montmorillonite or Ca-montmorillonite for use in the removal of BPA from aqueous solutions. The hydrophobicity introduced by loading the surfactant molecules contributes a partitioning phase, which strongly interacts with the BPA molecule through hydrophobic interactions.43,44 Polyethersulfone was introduced to HDTMA-modified montmorillonite by a liquid–liquid phase separation technique. The resultant hybrid material was then used as a molecular sieve to remove BPA from aqueous solutions. Moreover, the BPA-loaded polyethersulfone–HDTMA-modified montmorillonite could be regenerated by washing with ethanol.45
Sericite is a natural clay mineral found widely in Korea, China and Japan. The crystal structure of sericite has a very compact layer structure and is generally known as ‘white powders of muscovite’. The chemical formula of muscovite is KSi3Al3O10(OH)2 and it has a layer charge density close to ≤2 e− per unit cell. The unit cell is composed of 2
:
1 T
:
O phyllosilcate layers.35,37,46 The high unit charge of sericite results in a strong electrostatic attractive force that holds the aluminosilicate layers and the cations in the interlayer space tightly together. Muscovite therefore does not swell in water and the cation-exchange capacity (CEC) is low compared with other common clays. However, muscovite has very varied properties, such as a molecularly smooth surface and resistivity to acids, and is used in fireproofing and electrical insulation.46 The interlayer spacing of the (002) plane is 10 Å. Sericite has been used for the removal of Cu(II), Pb(II), Ni(II) and Cs(I) from aqueous solution.47–49 Sericite can also be modified with HDTMA and alkyldimethylbenzylammonium chloride (AMBA) to obtain organo-modified sericite; this has been used in the removal of arsenic and phenol from aqueous solution.50,51 Similarly, aluminum-pillared sericite subsequently modified with HDTMA or AMBA has been synthesized and used in the attenuation of As(III) and As(V) from aqueous solution.52 The work reported here extends the use of organo- or inorgano–organo-modified sericite hybrid materials in the remediation of waters contaminated with BPA using batch and column reactor procedures.
2. Materials and methods
2.1 Materials
Sericite was obtained from the Keumnam deposit, Gagokmyun, Samcheok City, Kangwon Province, Korea. The sample was crushed in a mortar and sieved to obtain 200–300 British Standard Sieve particles. The powdered sericite was washed with purified water and dried at 90 °C in a drying oven. The CEC of sericite was determined using the standard United States Environmental Protection Agency method 9080 (http://www.epa.gov/osw/hazard/testmethods/sw846/pdfs/9080.pdf) and was 8.85 mEquiv./100 g of sericite. Sericite contained various metal oxides, as reported previously.47 HDTMA [CH3(CH2)15N(CH3)3Br)] was obtained from Sigma-Aldrich (St Loius, MO, USA) and AMBA (total assay C22H40NCl) 50% solution and aluminum(III) chloride were obtained from Junsei Chemical Co. Ltd (Japan). BPA (C15H16O2) was obtained from Sigma-Aldrich and was used without further purification (Scheme 1). Sodium chloride (Extrapure) was obtained from HiMedia, India. The other chemicals used were of analytical-reagent or equivalent grade. The deionized water was further purified (18 MΩ cm) using a Millipore water purification system (Milli-Q+).
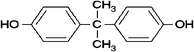 |
| Scheme 1 Chemical structure of bisphenol A. | |
2.2 Methodology
2.2.1 Preparation of HDTMA sericite or AMBA sericite. HDTMA- or AMBA-modified sericite was obtained by the wet cation-exchange method reported previously.50 In brief, 20 g of sericite was taken into 1 L of HDTMA or AMBA solution to give a CEC of 1 for sericite. The mixture was refluxed for 48 h at 60 °C with constant stirring. The slurries were filtered and the solid washed with purified water. Any excess or free surfactant was cleaned by Soxhlet extraction. The organo-modified sericite samples were then dried at 90 °C and stored in an airtight polyethylene bottle until further use. The HDTMA sericite and AMBA sericite are referred to as the HS and AS samples, respectively.
2.2.2 Preparation of inorgano–organo-sericite. Aluminum-pillared and HDTMA- or AMBA-modified sericites were obtained by the wet cation-exchange method reported previously.52 In brief, the pillaring aluminum solution was prepared by mixing 100 mL of 0.4 mol L−1 NaOH solution with 100 mL of 0.2 mol L−1 AlCl3 solution with vigorous and constant stirring. The mixture was stored for 7 days at room temperature to age. A known amount of HDTMA or AMBA solution was then added to this solution with continuous stirring; the HDTMA or AMBA was taken as equivalent to a 1
:
1 CEC of sericite. Sericite powder (4 g) was taken separately into 300 mL of water. The HDTMA or AMBA mixed aluminum solution was added to this dispersed mixture with vigorous stirring. The mixture was stirred for about 5 h at room temperature and then stored at room temperature for another 2 days. The dispersion was separated carefully and the solid was washed with purified water. The obtained solids [Al-HDTMA-sericite (AHS) and Al-AMBA-sericite (AAS)] were dried at 50 °C and gently ground in a mortar.
2.2.3 Characterization and surface morphology of hybrid materials. The surface morphologies of these hybrid materials and sericite were obtained by scanning electron microscopy using a Model FE-SEM SU-70 scanning electron microscope (Hitachi, Japan). X-ray diffraction (XRD) data were collected by a Model X'Pert PRO MPD X-ray diffractometer (PANalytical, the Netherlands). The diffraction data were recorded at a scan rate of 0.034 of 2θ illumination at an applied voltage of 45 kV with a measured current of 35 mA. The Cu Kα radiation used had a wavelength of 1.5418 Å. The solid materials were characterized by FT-IR spectrometry using the KBr disk method (Tensor 27, Bruker, USA).
2.2.4 pHPZC measurements. The pHPZC (point of zero charge) of the materials was obtained by the previously reported method.53,54 The pHPZC value is taken as the point at which the curve crossed the line at which pHfinal = pHinitial. The Brunauer–Emmett–Teller (BET) surface area was obtained using the N2 adsorption method with a BET surface area analyzer (Model-1201, Macsorb HM, Japan).
2.2.5 Batch reactor experiments. A stock solution of 100 mg L−1 BPA was prepared by dissolving an appropriate amount of BPA in purified water. The solubility is greatly enhanced by sonicating the solution for 5 min. The required BPA concentrations were then obtained by the successive dilution of stock solutions. A 50 mL aliquot of 10.0 mg L−1 of BPA solution was taken into polyethylene bottles and the pH was adjusted by adding concentrated HCl/NaOH solution dropwise. The pH was measured using an Orion 2 Star pH Benchtop pH meter (Thermoscientific, USA). AS, HS, AHS, or AAS solid (0.1 g) was then added into these bottles. The bottles were kept in an automatic incubator shaker (TM Weiber, ACMAS Technologies Pvt Ltd, India) for 24 h at 25 ± 1 °C. The samples were then removed from the shaker and filtered with a 0.45 μm syringe filter; the pH was then checked again and recorded. The filtrates are subjected to measure the absorbance of solutions using a UV-visible spectrophotometer (Model UV1, Thermo Electron Corporation, USA) at 276 nm. A calibration graph was obtained using standard BPA solutions with concentrations of 1.0, 5.0, 10.0, 20.0 and 25.0 mg L−1.The effect of the BPA concentration on adsorption was studied by varying the BPA concentration from 1.0 to 20.0 mg L−1 at a constant pH of about 6.5 and a constant temperature of 25 ± 1 °C. The sorption process was carried out as reported previously. The results are reported in terms of the initial BPA concentration (mg L−1) against the percentage of BPA removed.
The dependence of the adsorption of BPA on the background electrolyte concentration was determined by varying the background electrolyte concentration from 0.0001 to 0.1 mol L−1 NaCl in the sorptive solution. The solution pH (about 6.5) and temperature (25 ± 1 °C) were kept constant throughout. The results are presented as the percentage of BPA removed against the background electrolyte concentration.
The time dependence of the adsorption of BPA by these hybrid materials was also determined. The initial BPA concentration was held at 10.0 mg L−1 with the hybrid materials at a constant dose of 2.0 g L−1 at a pH of about 6.5 and a temperature of 25 ± 1 °C. The results are reported as the amount of BPA removed (mg g−1) as a function of time (min). The time dependent adsorption data were also used to fit various known kinetic models, e.g. the pseudo-first-order (PFO), pseudo-second-order (PSO) and fractal-like pseudo-second-order (FL-PSO) models.
2.2.6 Column experiments. A glass column (1 cm inner diameter) packed with 0.5 g of hybrid materials (in the middle of the column) was used for the column experiments. About 1.5 g of 14–16 British Standard Sieve size clean sand particles were placed above and below the hybrid materials and the column was packed with glass beads. A BPA 10.0 mg L−1 solution with a constant pH of 6.5 was pumped up from the bottom of the column using a peristaltic pump (KrosFlo Research I Peristaltic Pump, Spectrum Laboratories Inc., CA, USA) at a constant flow-rate of 1.0 mL min−1. The effluent samples were collected using a fraction collector (Spectra/Chrom CF-2 Fraction Collector, Spectrum Laboratories). The liquid samples collected were filtered using a 0.45 μm syringe filter and the total bulk sorptive concentration was measured using UV-vis spectrophotometry.The breakthrough data were used to optimize the loading capacity of the solids under dynamic conditions using the Thomas equation (eqn (1)):55
|
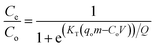 | (1) |
where
Ce and
Co are the concentrations (mg L
−1) of the effluent and influent BPA solutions, respectively,
KT is the Thomas rate constant (L min
−1 mg
−1),
qo is the maximum amount of BPA loaded (mg g
−1) under the specified column conditions,
m is the mass (g) of hybrid materials used,
V is the throughput volume (L) and
Q is the flow-rate of the pumped sorptive solution (L min
−1). A non-linear regression calculation was performed using the column data by the least-squares fitting method for two unknown parameters (
KT and
qo).
3. Results and discussion
3.1 Characterization of hybrid materials
The hybrid materials (AS, HS, AHS and AAS) were characterized by FT-IR spectrometry and XRD and their surface morphology was studied using SEM. The results have been reported previously.50,52 The pHPZC values were 6.89, 5.10, 6.20, 6.20 and 6.30 for sericite, HS, AS, AAS and AHS, respectively. The specific BET surface areas were 2.06, 5.72, 6.60, 0.93 and 1.71 m2 g−1 for sericite, AS, HS, AAS and AHS, respectively. The BET results show that the modified samples HS and AS have a significantly higher specific surface area than unmodified sericite. This may be a result of the introduction of the HDTMA or AMBA molecules within the interlayer space, which acts to prop up the interlayer space. In contrast, the AAS and AHS hybrid materials have a decreased specific surface area, perhaps because the pores are occupied by small aluminum oxide particles.
3.2 Batch reactor operations
3.2.1 Effect of pH. The mechanism involved at the solid–solution interface was investigated by studying the dependence of the adsorption of BPA on pH. This was obtained by varying the solution pH from about pH 2.0 to 12.0. The percentage uptake of BPA is presented as a function of pH in Fig. 1. Fig. 1 clearly shows that the very high percentage uptake of BPA (90%) by these hybrid materials is almost unaffected in the pH range 2.0–10.0. BPA has two hydrogens that can be dissociated. The values of pKa1 and pKa2 have been reported to be 9.9 and 10.2, respectively.56 The anionic species BPA− and BPA2− are therefore dominant at pH > 9.9 (Reaction (1)). However, in the pH range 2.0–9.0, BPA predominantly exists as a neutral molecule. All these solids have a net negative charge above about pH 6, depending on their pHPZC values (Reaction (2)). It is interesting to note that the uptake of BPA is not affected by the variation in the charge carried by these solids. Therefore the high uptake of BPA can be attributed to the fact that the HDTMA- or AMBA-modified sericite has a high hydrophobicity and strong organophilic behavior, which effectively partitions the BPA molecule. This indicates that partitioning is a driving force in this pH range for the attenuation of BPA by AS, HS, AAS and AHS hybrid materials. These hybrid materials are therefore effective molecular sieves for the removal of BPA from aqueous solutions.
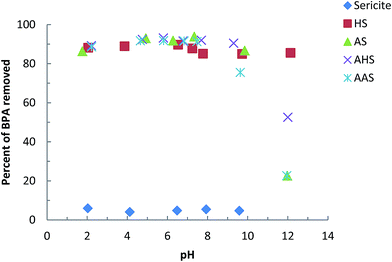 |
| Fig. 1 pH dependence of the adsorption of BPA by sericite, AS, HS, AHS and AAS. | |
Chiou et al.57,58 previously reported that non-ionic organic compounds are adsorbed onto a soil phase by partitioning between water and the soil organic phase and that this largely depends on the solubility of the organic component. Benzene, toluene and phenol were sequentially partitioned by organo-modified zeolites.59 An enhanced π–π dispersion interaction was suggested in the pH range in which the surface and BPA carry no net charge.25 Similarly, a strong π–π interaction between the benzene ring of BPA and the hydrophobic benzene ring of the solid surface can be demonstrated. This leads to hydrogen bonding of the phenolic group of BPA with the Al(OH)4 on the surface of the sorbent.1
|
 | (2) |
|
 | (3) |
With a further increase in pH beyond about pH 10.0, a sharp decrease in the percentage uptake of BPA occurred with the AS and AAS hybrid materials. This may be because the surface of these solids and BPA both carry a net negative charge, which greatly enhances the repulsive electrostatic forces. The solute is therefore prevented from entering the interlayer space, hence restricting the partitioning or sorption of BPA by these solids. Similar findings have been reported previously for the adsorption of BPA by ordered mesoporous carbon28 or multi-walled carbon nanotubes.30 However, the removal of BPA by the HS or AHS hybrid materials was either not affected, or insignificantly affected, at pH 12.0, which shows the high affinity of BPA towards the solid surfaces of HS and AHS. This high affinity means that there is little effect on the immobilization of BPA. This may be attributed to the fact that HDTMA is an aliphatic organic cation, whereas AMBA is an aromatic cation ring. Aliphatic chain compounds are flat and form a stable monolayer within the interlayer space of sericite, whereas aromatic compounds have a less stable structure. Similar results have been reported previously for the adsorption of As(III) and As(V) from organo-modified sericite50 and aluminum-HDTMA- or aluminum-AMBA-modified sericites.52
Fig. 1 also compares the properties of the modified sericite with unmodified sericite. Unmodified sericite removes an almost negligible percentage of BPA. This again indicates that the introduction of an organic molecule within the sericite network greatly enhances its hydrophobicity and organophilic nature and hence favors the partitioning of BPA between the solid and aqueous medium, providing useful sieving of BPA from aqueous solutions at the molecular level.
3.2.2 Effect of BPA concentration. The dependence of adsorption by AS, HS, AAS and AHS on the concentration of BPA was determined by varying the concentration of BPA from about 1.0 to 20.0 mg L−1 at pH 6.5 and 25 ± 1 °C (Fig. 2). Fig. 2 clearly shows that increasing the initial concentration of BPA from 1.53 to 19.51 mg L−1 did not significantly affect the percentage of BPA removed from aqueous solutions. These results suggest that these materials have an enhanced affinity towards BPA and that they may be useful molecular sieves that could be used to remove BPA from aqueous solutions. As the high uptake of BPA onto these solids was unaffected by the initial concentration of BPA, adsorption isotherm studies were not carried out.
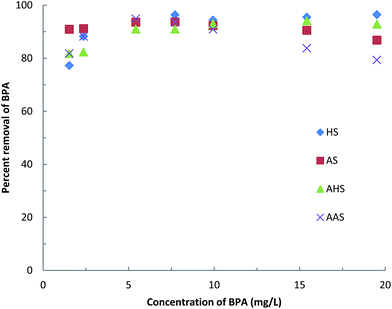 |
| Fig. 2 Effect of concentration of BPA on its removal from aqueous solution by AS, HS, AHS and AAS. | |
3.2.3 Effect of background electrolyte concentrations. The effect of the background electrolyte concentration on the adsorption of BPA by the hybrid materials could help to explain the mechanism taking place at the solid/solution interfaces.60 The specific adsorption is not affected by changes in the background electrolyte concentrations, but the non-specific adsorption is greatly affected by such changes. The adsorption of BPA was therefore investigated at various background electrolyte concentrations (NaCl from 0.0001 to 0.1 mol L−1). The initial BPA concentration was kept constant at 10.0 mg L−1 at pH 6.5. Fig. 3 shows the results as a function of the percentage removal of BPA against the background electrolyte concentrations. Increasing the background electrolyte concentration from 0.0001 to 0.1 mol L−1 NaCl caused a decrease in the removal of BPA as follows: AS, a 3.21% decrease (90.54–87.33%); HS, a 3.38% decrease (95.95–92.57%); AAS, 2.59% decrease (93.92–91.33%); and AHS, a 4.62% decrease (96.62–92.00%). These results indicate that a 1000-fold increase in the background electrolyte concentration of NaCl causes an insignificant decrease in the removal of BPA by these hybrid materials. Therefore it is inferred that BPA is immobilized by strong forces and is likely to bind specifically on these hybrid materials. It has been reported previously that As(V) was adsorbed specifically onto γ-Al2O3 in the pH range 3.0–9.2, whereas As(III) was not adsorbed specifically in the pH range 4.5–9.0.61,62 Similarly, when using an anion-exchange polymer resin the uptake of diclofenac from aqueous solutions was not significantly affected in the presence of citrate or phosphate.63 Other studies have suggested that the adsorption of BPA onto activated carbon is slightly enhanced at very high ionic strengths (0.5 mol L−1 NaCl), possibly because screening of the surface charge and the favoring of π–π interactions enhanced the adsorption of BPA along with the salting-out effect.25,64,65
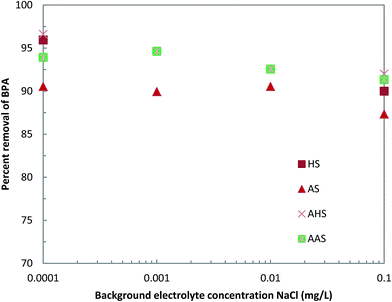 |
| Fig. 3 Effect of background electrolyte concentration (NaCl) on the removal of BPA by AS, HS, AAS and AHS. | |
3.2.4 Kinetic studies. The time dependence of the adsorption of BPA by the hybrid materials was investigated by varying the time of contact from 5 to 180 min. The initial BPA concentration was kept constant at 10.0 mg L−1 (pH 6.5). Fig. 4 shows that the immobilization of BPA on these hybrid materials is fairly fast. Within the initial few minutes of contact most of the BPA is aggregated by the solids and an apparent equilibrium is achieved within 60 min of contact. The fast initial uptake of BPA results in a higher affinity of these hybrid materials towards BPA.
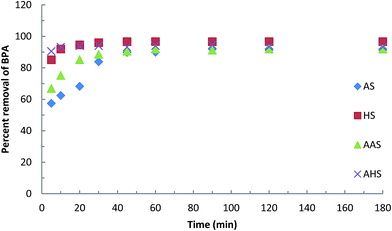 |
| Fig. 4 Time dependence of the adsorption of BPA by AS, HS, AHS and AAS. | |
The time dependence data was used in kinetic studies to determine the rate constant and the capacity of these solids to remove BPA. The following non-linear PFO,66 pseudo-second-order (PSO)67 and fractal-like pseudo-second-order (FL-PSO)68 kinetic models were used:
|
qt = qe(1 − exp(−k1t))
| (4) |
|
 | (5) |
|
 | (6) |
where
qt and
qe are the amounts of BPA adsorbed at time
t and the adsorption capacity at equilibrium, respectively.
k1 and
k2 are the PFO and PSO rate constants, respectively. The constants
k and
α are the rate constant and the fractal constant, respectively. A non-linear least-squares fitting was carried out to estimate the unknown parameters and the results are shown in
Fig. 5a–c for the PFO, PSO and FL-PSO kinetic models, respectively.
Table 1 gives the estimated values of the unknown parameters and the least-squares sum. The results are better fitted to the PSO and FL-PSO models than to the PFO model. The adsorption capacity obtained by these models was comparable, except for AS using the FL-PSO model. The theoretical studies indicate that the rate coefficient of PSO model (
k2) is a complex function of the initial concentration of the adsorbate species.
66 However, the FL-PSO model is based on the fact that different paths of adsorption take place and the adsorption rate coefficient is a function of time.
69 The PFO kinetic model, which was derived by Lagergren method, could show the properties of the Langmuir rate at initial times of adsorption or close to equilibrium.
66,70 The applicability of the PSO model indicates that the adsorbate species are bound onto the surface of the solids by strong forces. It has been reported previously that the adsorption of divalent metal cations onto
sphagnum moss peat follows second-order rate laws.
71,72 They further indicated that the metal cations are forming strong chemical forces with the surface active groups of peat.
71,72
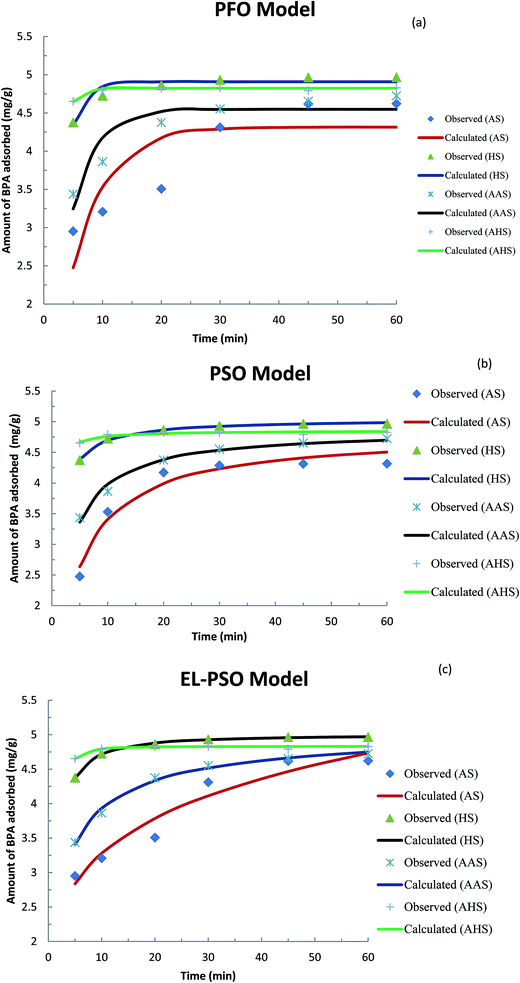 |
| Fig. 5 Fitting of different models for the adsorption of BPA by AS, HS, AAS and AHS hybrid materials: (a) PFO model; (b) PSO model; and (c) FL-PSO model. | |
Table 1 Estimated kinetic parameters for the PFO, PSO and FL-PSO kinetic models in the sorption of BPA by AS, HS, AAS and AHS hybrid materialsa
Model |
Constant evaluated |
System |
AS |
HS |
AAS |
AHS |
AS = AMBA-sericite; HS = HDTMA-sericite; AAS = aluminum-HDTMA-sericite; and AHS = aluminum-HDTMA-sericite; s2 = least-squares sum. |
PFO |
qe (mg g−1) |
4.315 |
4.908 |
4.547 |
4.823 |
k1 (min−1) |
0.170 |
0.434 |
0.250 |
0.667 |
s2 |
0.9614 |
0.0242 |
0.1968 |
0.0030 |
PSO |
qe (mg g−1) |
4.816 |
5.047 |
4.874 |
4.856 |
k2 |
0.050 |
0.265 |
0.091 |
1.037 |
s2 |
0.4360 |
0.0015 |
0.0204 |
0.0039 |
FL-PSO |
qe |
7.851 |
5.003 |
5.166 |
4.830 |
k |
0.037 |
0.190 |
0.120 |
0.132 |
α |
0.396 |
1.237 |
0.710 |
2.309 |
S2 |
0.1964 |
0.0005 |
0.0089 |
0.0023 |
3.3 Column studies
Fixed-bed column studies were carried out using the column conditions described earlier. Breakthrough curves were obtained for AHS and AAS (as a reference material) for the removal of BPA (Fig. 6). A relatively high breakthrough volume was obtained for BPA using AAS and AHS. Quantitatively, complete breakthrough was obtained at a BPA throughput of 0.12 and 0.20 L for AAS and AHS, respectively. The relatively high breakthrough volume obtained for BPA indicates the strong affinity of these solids towards BPA under dynamic conditions.73,74 It was reported previously that fixed-bed processes have the additional advantage of continuous contact of the adsorbing species with the solid surface, which results in better exhaustion of adsorbent solids.75
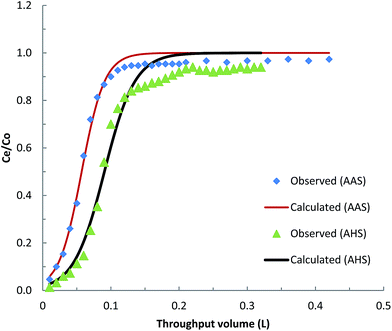 |
| Fig. 6 Breakthrough curves for the removal of BPA by AAS and AHS. | |
A non-linear least-squares analysis was performed using the breakthrough column data to simulate the two unknown constants KT and qo (Fig. 6). Table 2 gives the values of the Thomas constants and the least-squares sum obtained for these systems. The data clearly show that a relatively high loading capacity is achieved for BPA by these solids, even under dynamic conditions. This demonstrates the useful practical application of the solids in the removal of BPA from aqueous solutions. These results are similar to the findings of batch reactor experiments in which the Thomas equation was fitted to demonstrate the loading capacity of different sorbents.52,76 The lower removal capacity obtained with column reactor operation compared with the corresponding batch reactor operation is a result of the insufficient time of contact available for the adsorption of BPA by these solids.47
Table 2 Thomas constants estimated for the removal of BPA by AAS and AHS solidsa
System |
Thomas constant |
Least-square sum (s2) |
qo |
KT |
AAS = aluminum-HDTMA-sericite; AHS: aluminum-HDTMA-sericite. |
BPA-AAS |
1.285 |
5.27 × 10−3 |
3.2 × 10−2 |
BPA-AHS |
2.035 |
3.95 × 10−3 |
1.2 × 10−1 |
4. Conclusions
Sericite, a natural mica-type clay, was modified with HDTMA (H) and AMBA (A) to obtain organo-modified sericites (HS and AS) and aluminum-H-sericite (AHS) and aluminum-A-sericite (AAS) inorgano–organo-modified sericite hybrid materials. These materials were characterized by FT-IR spectrometry and XRD and the surface morphology was studied using SEM. The pHPZC values for these solids were 6.89, 5.10, 6.20, 6.20 and 6.30 for sericite, HS, AS, AAS and AHS, respectively. These hybrid materials were used as effective and efficient molecular sieves for the removal of BPA from aqueous solutions. Batch experiments were performed to determine various physico-chemical parameters. Data for the effect of pH (2.0–12.0) on the uptake of BPA showed that the adsorption of BPA is almost independent of pH in the range 2.0–10.0, but above this pH it is significantly decreased, at least for AS and AAS. The concentration of BPA (1.0–20 mg L−1) and the background electrolyte concentration (NaCl 0.0001–0.1 mol L−1) do not significantly affect the percentage uptake of BPA by these hybrid materials. The kinetic data show that BPA is rapidly immobilized by the hybrid materials and the kinetic data fits well to the PSO and FL-PSO models. The overall capacity for the removal of BPA was 4.816, 5.047, 4.874 and 4.856 mg g−1 for the AS, HS, AAS and AHS hybrid materials, respectively. The materials were used under dynamic conditions in column reactor operations. The breakthrough curves could be used for non-linear fitting to the Thomas equation. The breakthrough data fits the Thomas equation well. The loading capacity for BPA was 1.285 and 2.035 for the AAS and HAS hybrid materials, respectively. These results indicate that these hybrid materials have the potential to be used as effective molecular sieves for the removal of BPA from aqueous solutions.
Acknowledgements
One of the authors (D.T.) acknowledges the CSIR, New Delhi for financial support in the form of Research Project no. 01 (2567)/12/EMR-II.
References
- M. Zhou, Y. Wu, J. Qiao, J. Zhang, A. McDonald, G. Li and F. Li, J. Colloid Interface Sci., 2013, 405, 157–163 CrossRef CAS PubMed.
- R. H. Waring and R. M. Harris, Mol. Cell. Endocrinol., 2005, 244, 2–9 CrossRef CAS PubMed.
- C. E. Gattullo, H. Bährs, C. E. W. Steinberg and E. Loffredo, Sci. Total Environ., 2012, 416, 501–506 CrossRef CAS PubMed.
- D. A. Crain, M. Eriksen, T. Iguchi, S. Jobling, H. Laufer and G. A. LeBlanc, Reprod. Toxicol., 2007, 24, 225–239 CrossRef CAS PubMed.
- A. V. Krishnan, P. Stathis, S. F. Permuth, L. Tokes and D. Feldman, Endocrinology, 1993, 132, 2279 CAS.
- S. Takahashi, X. J. Chi, Y. Yamaguchi, H. Suzuki, S. Sugaya and K. Kita, Mutat. Res., 2001, 490, 199–207 CAS.
- M. M. Munoz de Toro, C. M. Markey, P. R. Wadia, E. H. Luque, B. S. Rubin, C. Sonnenschein and A. M. Soto, Endocrinology, 2005, 146, 4138–4147 CrossRef CAS PubMed.
- K. L. Howdeshell, A. K. Hotchkiss, K. A. Thayer, J. G. Vandenbergh and F. S. vom Saal, Nature, 1999, 401, 763–764 CrossRef CAS PubMed.
- J. Chen, X. Huang and D. Lee, Process Biochem., 2008, 43, 451–456 CrossRef CAS PubMed.
- C. A. Staples, P. B. Dorn, G. M. Klecka, S. T. O'Block and L. R. Harris, Chemosphere, 1998, 36, 2149–2173 CrossRef CAS.
- J. G. Teeguarden and S. Hanson-Drury, Food Chem. Toxicol., 2013, 62, 935–948 CrossRef CAS PubMed.
- T. Geens, T. G. Apelbaum, L. Goeyens, H. Neels and A. Covaci, ntake of bisphenol A from canned beverages and foods on the Belgian market, Food Addit. Contam., Part A, 2010, 27, 1627–1637 CrossRef CAS PubMed.
- J. Yonekubo, K. Hayakawa and J. Sajiki, J. Agric. Food Chem., 2008, 56, 2041–2047 CrossRef CAS PubMed.
- T. Yamamoto, A. Yasuhara, H. Shiraishi and O. Nakasugi, Chemosphere, 2001, 42, 415–418 CrossRef CAS.
- T. Bushnik, D. Haines, P. Levallois, J. Levesque, J. Van Oostdam and C. Viau, Health Reports, 2010, 21, 7–18 Search PubMed.
- A. M. Calafat, X. Ye, L. Y. Wong, J. A. Reidy and L. L. Needham, Environ. Health Perspect., 2008, 116, 39–44 CrossRef CAS PubMed.
- J. S. Lakind, J. Levesque, P. Dumas, S. Bryan, J. Clarke and D. Q. Naiman, J. Exposure Sci. Environ. Epidemiol., 2012, 22, 219–226 CrossRef CAS PubMed.
- Commission of the European Communities, Opinion of the Scientific Committeeon Food on Bisphenol SCF/CS/PM/3936, (2002).
- A WWF European Toxics Programme Report, Bisphenol A: A Known Endocrine Disruptor, Registered Charity No. 20170 (2000).
- S. Yüksel, N. Kabay and M. Yüksel, J. Hazard. Mater., 2013, 263, 307–310 CrossRef PubMed.
- Y. H. Kim, B. Lee, K. H. Choo and S. J. Choi, Microporous Mesoporous Mater., 2011, 138, 184–190 CrossRef CAS PubMed.
- C. Y. Wang, H. Zhang, F. Li and L. Y. Zhu, Environ. Sci. Technol., 2010, 44, 6843–6848 CrossRef CAS PubMed.
- A. Svenson, A. S. Allard and M. Ek, Water. Res., 2003, 37, 4433–4443 CrossRef CAS.
- Chemistry and Analytical Methods for Determination of Bisphenol A in Food and Biological Samples FAO/WHO Expert Meeting on Bisphenol A (BPA) Ottawa, Canada, 2–5 November (2010). Report Prepared by Xu-Liang Cao, (E-mail: http://www.who.int/foodsafety/chem/chemicals/1_chemistry_analytical_methods.pdf).
- G. Liua, J. Mab, X. Li and Q. Qin, J. Hazard. Mater., 2009, 164, 1275–1280 CrossRef PubMed.
- W.-T. Tsai, C.-W. Lai and T.-Y. Su, J. Hazard. Mater., 2006, B134, 169–175 CrossRef PubMed.
- L. Joseph, L. K. Boateng, J. R. V. Flora, Y.-G. Park, A. Son, M. Badawy and Y. Yoon, Sep. Purif. Technol., 2013, 107, 37–47 CrossRef CAS PubMed.
- Q. Sui, J. Huang, Y. Liu, X. Chang, G. Ji, S. Deng, T. Xie and G. Yu, J. Environ. Sci., 2011, 23, 177–182 CrossRef CAS.
- J. Heo, J. R. V. Flora, N. Her, Y.-G. Park, J. Cho, A. Son and Y. Yoon, Sep. Purif. Technol., 2012, 90, 39–52 CrossRef CAS PubMed.
- L. Zhang, F. Pan, X. Liu, L. Yang, X. Jiang, J. Yang and W. Shi, Chem. Eng. J., 2013, 218, 238–246 CrossRef CAS PubMed.
- Y. Zhou, P. Lu and J. Lu, Carbohydr. Polym., 2012, 88, 502–508 CrossRef CAS PubMed.
- Y. Zhou, L. Chen, P. Lu, X. Tang and J. Lu, Sep. Purif. Technol., 2011, 81, 184–190 CrossRef CAS PubMed.
- S. Tang, N. Lu, J. Li and Y. Wu, Thin Solid Films, 2012, 521, 257–260 CrossRef CAS PubMed.
- L. K. Boateng, J. Heo, J. R. V. Flora, Y.-G. Park and Y. Yoon, Sep. Purif. Technol., 2013, 116, 471 CrossRef CAS PubMed.
- S. M. Lee and D. Tiwari, Appl. Clay Sci., 2012, 59–60, 84 CrossRef CAS PubMed.
- S. W. Bailey, in Crystal Structure of Clay Minerals and Their X-Ray Identification, ed. G. W. Brndley and G. Brown, Mineralogical Society London, 1980, pp. 1–113 Search PubMed.
- J. Konta, Appl. Clay Sci., 1995, 10, 275–335 CrossRef CAS.
- G. R. Alther, Waste Manag., 2002, 22, 507–513 CrossRef CAS.
- J. Q. Jiang, C. Cooper and S. Ouki, Chemosphere, 2002, 47, 711–716 CrossRef CAS.
- J. Smith, S. Bartlett-Hunt and S. Burns, J. Hazard. Mater., 2003, B96, 91–97 CrossRef.
- R. S. Bowman, Z. Li, S. J. Roy, T. Burt, T. L. Johnson and R. L. Johnson, in Physical and Chemical Remediation of Contaminated Aquifers, ed. J. A. Smith and S. Burns, Kluwer Academic Pulishers, 2002, pp. 161–163 Search PubMed.
- Z. Li and R. S. Bowman, Water. Res., 2001, 35, 3771–3776 CrossRef CAS.
- Y. Park, Z. Sun, G. A. Ayoko and R. L. Frost, Chemosphere, 2014, 107, 249–256 CrossRef CAS PubMed.
- S. Zheng, Z. Sun, Y. Park, G. A. Ayoko and R. L. Frost, Chem. Eng. J., 2013, 234, 416–422 CrossRef CAS PubMed.
- F. Cao, P. Bai, H. Li, Y. Ma, X. Deng and C. Zhao, J. Hazard. Mater., 2009, 162, 791–798 CrossRef CAS PubMed.
- Y. J. Shih and Y. H. Shen, Appl. Clay Sci., 2009, 43, 282–288 CrossRef CAS PubMed.
- D. Tiwari, H. U. Kim and S. M. Lee, Sep. Purif. Technol., 2007, 57, 11–16 CrossRef CAS PubMed.
- C. Jeon and T. N. Kwon, J. Ind. Eng. Chem., 2013, 19, 68–72 CrossRef PubMed.
- J. O. Kim, S. M. Lee and C. Jeon, Chem. Eng. Res. Des., 2014, 92, 368–374 CrossRef CAS PubMed.
- S. M. Lee and D. Tiwari, Environ. Sci. Pollut. Res., 2014, 21, 407–418 CrossRef CAS PubMed.
- D. Tiwari, W. Kim, M. Kim, S. K. Prasad and S.-M. Lee, Desalin. Water Treat., 2013 DOI:10.1080/19443994.2013.846562.
- D. Tiwari and S. M. Lee, Chem. Eng. J., 2012, 204–206, 23–31 CrossRef CAS PubMed.
- J. Rivera-Utrilla, I. Bautista-Toledo, M. A. Ferro-Garcia and C. Moreno-Castilla, J. Chem. Technol. Biotechnol., 2001, 76, 1209–1215 CrossRef CAS PubMed.
- L. S. M. Lee and D. Tiwari, Chem. Eng. J., 2013, 225, 128–137 CrossRef PubMed.
- H. C. Thomas, J. Am. Chem. Soc., 1944, 66, 1664–1666 CrossRef CAS.
- C. Tian, J. T. Wang and X. L. Song, Water, Air, Soil Pollut., 2009, 199, 301–310 CrossRef CAS.
- C. T. Chiou, L. J. Peters and V. H. Freed, Science, 1979, 206, 831–832 CAS.
- C. T. Chiou, P. E. Porter and D. W. Schmedding, Environ. Sci. Technol., 1983, 17, 227–231 CrossRef CAS PubMed.
- M. Ghiaci, A. Abbaspur, R. Kia and F. Seyedeyn-Azad, Sep. Purif. Technol., 2004, 40, 217–229 CrossRef CAS PubMed.
- K. F. Hayes, C. Papelis and J. O. Leckie, J. Colloid Interface Sci., 1988, 125, 717–726 CrossRef CAS.
- Y. Arai, E. J. Elzinga and D. L. Sparks, J. Colloid Interface Sci., 2001, 235, 80–88 CrossRef CAS PubMed.
- Z. Li, T. Burt and R. S. Bowman, Environ. Sci. Technol., 2000, 34, 3756–3760 CrossRef CAS.
- K. A. Landry and T. H. Boyer, Water. Res., 2013, 47, 6432–6444 CrossRef CAS PubMed.
- V. Lopez-Ramon, C. Moreno-Castilla, J. Rivera-Utrilla and L. R. Radovic, Carbon, 2002, 41, 2009–2025 Search PubMed.
- G. Newcombe and M. Drikas, Carbon, 1997, 35, 1239–1250 CrossRef CAS.
- S. Azizian, J. Colloid Interface Sci., 2004, 276, 47–52 CrossRef CAS PubMed.
- Y. S. Ho and G. Mckay, Process Saf. Environ. Prot., 1998, 76, 332–340 CrossRef CAS.
- M. Haerifar and S. Azizian, Chem. Eng. J., 2013, 215–216, 65–71 CrossRef CAS PubMed.
- M. Haerifar and S. Azizian, J. Phys. Chem. C, 2012, 116, 13111–13119 CAS.
- Y. Liu and L. Shen, Langmuir, 2008, 24, 11625–11630 CrossRef CAS PubMed.
- Y. S. Ho, J. Hazard. Mater., 2006, B136, 681–689 CrossRef PubMed.
- Y. S. Ho and G. McKay, Water. Res., 2000, 34, 735–742 CrossRef CAS.
- J. T. Matheickal and Q. Yu, Bioresour. Technol., 1999, 69, 223–229 CrossRef CAS.
- M. A. Stylianou, M. P. Hadjiconstantinou, V. J. Inglezakis, K. G. Moustakas and M. D. Loizidou, J. Hazard. Mater., 2007, 143, 575–581 CrossRef CAS PubMed.
- A. Adak, M. Bandyopadhyay and A. Pal, Dyes Pigm., 2006, 69, 245–251 CrossRef CAS PubMed.
- A. Singh, D. Kumar and J. P. Gaur, Water. Res., 2012, 46, 779–788 CrossRef CAS PubMed.
|
This journal is © The Royal Society of Chemistry 2014 |
Click here to see how this site uses Cookies. View our privacy policy here.