DOI:
10.1039/C4RA07649D
(Paper)
RSC Adv., 2014,
4, 49560-49568
Detection of dopamine on a poly(metanilic acid) decorated two-dimensional gold cavity array electrode†
Received
26th July 2014
, Accepted 16th September 2014
First published on 16th September 2014
Abstract
A shape-controllable, highly ordered, two-dimensional gold cavity array (GCA) electrode was prepared by electrodeposition using a closely packed monolayer of 1.2 μm-diameter polystyrene spheres as a template and was characterized by FESEM and XRD. A significant enhancement for the electrooxidation of dopamine at this nanostructured electrode was found due to the increased amount of the active surface area. By electropolymerizing a poly(metanilic acid) thin film on its surface, the enhanced electrochemical properties of the GCA electrode were maintained, and the antifouling property was improved. The SWV technique was used for the trace determination of DA, and the dependence of current vs. concentration was linear from 0.2 to 100 μM with a regression coefficient of 0.9988, and the detection limit of DA was ∼0.08 μM. Furthermore, the signals of DA and UA can be well distinguished at this poly(metanilic acid) modified GCA electrode. The proposed method was applied to the selective and precise analysis of DA in commercial injections.
1. Introduction
Dopamine (DA) is an important neurotransmitter, which plays a vital role in the mammalian central and peripheral nervous systems and is involved in the regulation of cognitive functions such as attention, stress, rewarding behavior, and reinforcing effects of certain stimuli.1,2 Moreover, abnormal levels of DA are associated with schizophrenia, senile dementia, and Parkinson's disease.1 Therefore, the rapid and accurate determination of DA in biosamples has attracted great interest among neuroscientists and chemists. Various approaches, including spectrophotometry,3,4 fluorescence,5 ultra-high performance liquid chromatography-mass spectrometry (HPLC-MS),6 and electrochemistry,7–16 have been established to perform DA determination. Although some of these methods show high sensitivity and selectivity (such as HPLC-MS), cumbersome experimental steps and reagent consumption are the stumbling blocks for high-efficiency detection.
Being an electroactive substance, DA can be easily detected by electrochemical methods through its oxidation at the surface of various electrodes. One of the primary problems is that, in real biological samples, the physiological concentration of DA is ultra-low (from 0.01 to 1 μM)8 and even in the nanomolar range for patients with Parkinson's disease, whereas the concentrations of the main detection interferences, e.g., ascorbic acid (AA) and uric acid (UA), are several orders of magnitude higher.17 A further complication is that both AA and UA are also electroactive and undergo oxidation within the same potential window as DA. To perform the DA detection with high sensitivity and selectivity, various chemically modified electrodes have been fabricated using metal or metal oxide nanoparticles,18 metal complexes,19 carbon materials,15 conductive polymers,17 and self-assembled monolayers.9 These modified electrodes show excellent properties in DA detection by decreasing the overpotential, which accelerates the electron and mass transfer rate and enlarges the electrode surface.
In recent years, porous metal electrodes with highly ordered nanostructures have attracted increasing research attention. The large active surface area and the ordered arrangement of pores of such two-dimensional or three-dimensional metal electrodes have found their important roles in many applications, such as electrocatalysis, spectroelectrochemistry, chemical and biological sensors, and microelectronic devices.20–22 For example, Kuhn's group reported the fabrication of a fluorenone-based mediator modified macroporous gold electrode to detect glucose in solution, and the currents increased by more than one order of magnitude relative to those achieved on flat electrode surfaces.23,24 Due to their uniform structures, macroporous electrodes can be also used to host enzymes and/or redox proteins at the monolayer level.25–27 Xia and co-workers25 prepared a three-dimensional macroporous gold electrode to enlarge the surface area and accelerate the direct electron transfer between the electrode and the adsorbed hemoglobin.
Although photolithography, electron beam lithography, X-ray lithography, scanning tunneling microscopy, and atomic force microscopy lithography are the most widely used methods to create defect-free nanostructured arrays, their high costs are beyond the purchasing power of most laboratories.28 Template-based methods have proven to be flexible and low-cost for preparing porous films. In this method, using highly ordered monodispersed nanoparticles (usually silica and polystyrene (PS) particles) as a template, functional materials can be filled into the interspaces of the template through vapor deposition, chemical deposition, and electrodeposition.29–31 A highly ordered macroporous film with the same shape and size as the template is obtained by removing the template through dissolution or thermal decomposition. The first example of electrochemical deposition through a colloidal template was reported by Braun and Wiltzius.32 In their excellent paper, the authors reported the electrodeposition of CdSe and CdS using 466 nm-diameter PS spheres as the template. Following this approach, the deposition materials have been extended to semiconductors, metals, metal oxides, and conducting polymers. Combined with electrodeposition, it is possible to control the morphology of the obtained macroporous film by employing different sizes of PS spheres, and by altering the electrochemical parameters during the deposition process. Furthermore, no shrinkage of the material occurs because electrodeposition ensures a high density of the deposited material within the interspaces of the template.33
Conductive polymers, such as polypyrrole, polythiophene, and polyaniline, have also been widely used to alleviate the above-mentioned problems with DA detection.34–36 Their widespread popularity arises not only because of their easier fabrication processes and good electrical conductivity, but also because it is possible to increase the functional density and facilitate electron exchange through chemical substitution on the polymer chain (doped conductive polymer). The multifunctionality of metal/conductive polymer composites is particularly useful, as they are endowed with enhanced electrochemical catalytic ability and better physical stability.37
In this work, we combine the benefits of a two-dimensional gold cavity array (GCA) electrode and poly(metanilic acid) to develop a sensitive and selective electrochemical DA sensor. The concave bowl-shaped morphology greatly increased the surface area available for the sensitive detection of the target analyte. A thin film of poly(metanilic acid) was then electropolymerized on the surface of the GCA electrode, and the electrocatalytic ability of the gold nanoparticles (AuNPs) and their strong interaction with the polyaniline backbone significantly improved the stability of the poly(metanilic acid). The conductivity and electrochemical activity in neutral solutions were greatly enhanced due to the –SO3H doped in the polymer chain. In this study, we demonstrate that by depositing this thin layer of poly(metanilic) onto the GCA electrode, DA concentrations as low as 0.08 μM with a linear range from 0.2 to 100 μM were detected with the square wave voltammetry (SWV) technique. Since the anti-fouling effect of the poly(metanilic) thin film, the problem of the adsorption of the DA oxidation product was avoided, ensuring the significant reproducibility and stability during DA detection. Moreover, DA and UA can be simultaneously detected when in binary mixtures.
2. Experimental section
2.1. Materials and apparatus
Dopamine (DA), uric acid (UA), metanilic acid, and tetrachloroauric(III) acid trihydrate were purchased from Sigma-Aldrich. Phosphate buffer solution (PBS, pH 7.0) was used as the supporting electrolyte and it was prepared with 0.1 M KH2PO4/K2HPO4. Polystyrene (PS) particles with 1.2 μm-diameter were synthesized by a dispersion polymerization of styrene in ethanol–water mixtures based on previous reports.38 All the reagents used were of analytical reagent grade. Ultrapure water used for washing and all buffer solution preparations was produced with a Millipore-Q system.
The SEM images were obtained using a Hitachi S4700 (Japan) field emission scanning electron microscope. X-ray diffraction (XRD) patterns were recorded at a Bruker AXSD8 ADVANCE X-ray diffractometer (Cu Kα radiation, λ = 0.15406 nm) at room temperature. The electropolymerization of poly(metanilic acid) and all electrochemical measurements were recorded at a CHI 660E electrochemical station (Shanghai ChenHua Instruments CO. LTD., China). A bare Au or gold cavity array electrode was used as the working electrode. In order to maintain a constant surface area of the working electrode, the surface of the electrode was sheltered by a sticker and only a circular portion of 0.5 cm diameter was exposed to the solution. Platinum and Ag|AgCl (3 M KCl) electrodes were used as the auxiliary and reference electrodes, respectively. All electrochemical measurements were conducted in an argon-purged (at least 15 min and maintained under an argon atmosphere during whole course) solution and at room temperature.
2.2. Preparation of electrodes with highly ordered gold cavities
The monolayer of PS particles was generated by self-assembly at the air/water interface in a 15 cm diameter Petri dish, and transferred onto the gold coated slides. For preparation, a silicon wafer substrate of 4 × 6 cm in size was ultrasonically cleaned in acetone, ethanol and then in distilled water for half an hour. The cleaned silicon wafer was then immersed in an isopropanol solution saturated with potassium hydroxide overnight to hydroxylate the surface and make it hydrophilic, and it was then washed again with ethanol and water. The PS template was formed on a gold coated ITO surface after self-assembly on a water surface using the following method. Several drops of sodium dodecyl sulfate (2 wt% in water) were added to the surface of 200 mL water in a 15 cm Petri dish. 1 mL of a 5 wt% PS nanosphere suspension (spheres with different diameters, 1
:
1 water–ethanol) was then spread slowly and evenly across the silicon wafer treated as described above, which was then placed into the water at a 45° angle. The nanosphere suspension ran down the silicon wafer onto the water surface, where it formed a stable film. The monolayer was transferred to a gold coated ITO by placing it under the monolayer and withdrawing it slowly from the solution. Areas up to 1 cm2 were easily modified using this method with very high quality. The gold coated slides used in this work were prepared by electromagnetic sputtering of a 10 nm thick chromium layer, followed by a 200 nm thick gold layer onto ITO glass slides.
The PS template-coated substrate was then used as the working electrode for further electrodeposition of gold in a plating bath solution containing 0.029 M HAuCl4, 0.017 M EDTA, 1.267 M Na2SO3 and 0.173 M K2HPO4 according to a method previously reported in the literature.39 The gold arrays were produced using multi-current pulse plating with the first pulse to a current density of 20 mA cm−2 for 100 ms, followed by a train of pulses of 5 mA cm−2 for 60 ms separated by a rest time of 1 s (zero current). Then, the electrode was immersed into toluene to dissolve the PS spheres, leaving an ordered array of interconnected sphere segment voids.
2.3. Electropolymerization of poly(metanilic acid)
The poly(metanilic acid) modified gold cavity array electrode was obtained by constant potential electrolysis at 0.9 V in 0.2 M sulfuric acid containing 40 mM metanilic acid. The polymerization was stopped when the charge (Q) was up to 2.0 mC, which resulted in a thin polymer film forming at this GCA electrode.40
2.4. Electrochemical Measurements
For the SWV determination of DA in this work, the initial potential and final potential were −0.20 and +0.70 V, respectively. The incremental potential was set as 4 mV, the pulse amplitude was 25 mV, and the frequency was 15 Hz.
3. Results and discussion
3.1. Preparation and characterization of the gold cavity array electrode
In our previous paper, we fabricated a 2-D polystyrene monolayer by dropping 2 μm-diameter PS suspensions in an appropriate proportion of water/alcohol to the surface of water.38 However, there were some defects in the crystal lattice, such as multilayers, dislocations, and vacancies, which largely affected the structure of the electrochemically deposited AuNPs and in turn, the electrochemical behaviors of the resulting electrode. In the present case, we first added the proper volume of surfactant SDS to the surface of water, which could markedly lower the surface tension of water. When the PS spheres ran down from the hydrophilic silicon wafer, the moderate surface tension prevented the PS spheres from spreading in a disordered manner, and thus a stable monolayer with close-packed PS spheres was formed. Fig. 1a shows the typical SEM image of the assembled PS particles. As can be seen, the highly ordered hexagonally arranged PS spheres can preserve their morphology when the monolayer of PS particles is transferred to the surface of the Au coated ITO electrode; moreover, the surface homogeneity is relatively good and no major defects are observed.
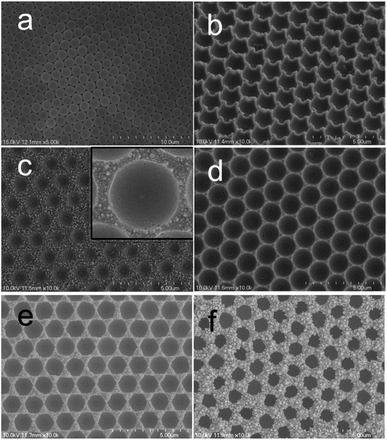 |
| Fig. 1 SEM images of (a) closely packed PS spheres with 1.2 μm-diameter, (b) tilted view of the as-prepared gold cavity array using template (a), (c–f) top view of the gold cavity arrays with increasing film thickness. The inset of (c) is a high magnification view of a single cavity. | |
Multi-current pulse plating is a preferable method for preparing uniformly sized metal nanoparticles that firmly adhere to the conducting substrate. Electrochemical deposition occurs from the electrode surface out through the overlying template, AuNPs gradually fill the interstices among the PS spheres, and the growth of AuNPs on the electrode surface is directed by the ordered structure of the assembled PS particles. As a result, the cavity arrays are obtained after removal of the template. Fig. 1b shows a tilted SEM image of a hemispheric cavity array grown through the template made up of a monolayer of 1.2 μm-diameter PS spheres (as shown in Fig. 1a). It can be clearly seen that the resulting gold cavities match the size of the PS particles and the array retains the two-dimensional close-packed ordering. The first pulse produced “gold seeds.” Fig. S1a† shows the SEM image of the “gold seeds” produced under a 20 mA cm−2 current density. As observed, these nanoparticle-like dots were evenly distributed on the electrode surface, which was the key factor to ensure good adhesion and uniform morphology. An appropriate increase in the current density of the first pulse resulted in the increased density of the gold nanoparticles (AuNPs) on the electrode and made the growth of the gold seeds more controllable. However, as shown in Fig. S1b,† the pulse current density was increased to 50 mA cm−2, the size distribution of the deposited gold seeds became uneven, thus affecting the morphology of the cavity array and further applications. The film thickness can be easily controlled by regulating the total amount of charges passed during the electrodeposition. As shown in Fig. 1c–f, the depth of the gold cavities increased with an increase in the repeat times of the second pulse. Moreover, it was found that when the interstices of the assembled PS particles were fully filled with AuNPs, the growth of AuNPs was no longer directed by the shape of the PS particles, showing up as irregular shapes of the pore mouths. Further increase in the number of the second pulses led to a continuous increase in the thickness until the PS template was completely covered.
The further magnified image (the inset of Fig. 1c) shows that the as-prepared gold cavities mainly consist of AuNPs in the size range of 10 to 20 nm and these AuNPs are in contact with each other, resulting in the final structure. The smooth inner surface of the cavities seems to indicate that the bottom site of each cavity is also uniformly covered with a thin layer of AuNPs. The UV-vis reflection spectra of the films further characterized the special morphology of the gold cavity array. As shown in Fig. S2,† the reflection spectrum of the flat Au substrate, the only dip that can be observed is at ca. 480 nm, which originates from the well-known surface plasmon resonance of AuNPs. The different dips in the reflection spectrum of the gold cavity array suggest the variation of the localized surface plasmon resonance (LSPR), as the plasmonic properties of metal nanostructures are critically dependent on their shape and size (void diameter, film thickness).41
3.2. Electrochemical behavior of DA at GCA electrodes
The typical CVs of 0.5 mM DA at the bare Au and GCA electrodes are shown in Fig. 2. A couple of definite reversible redox peaks, located at 0.132 and 0.272 V, were observed at the GCA electrode. The same redox peaks can also be obtained at the bare Au electrode. However, the peak current for DA oxidation at the GCA electrode was 74.66 μA, which was ca. 9 times larger than that recorded at the bare Au electrode (8.43 μA) under identical working conditions. This could be first attributed to the significant advantage of the nanostructured GCA electrode with a large electroactive surface area due to the cavity morphology. Previous literature has shown that three-dimensional macroporous gold films can significantly enhance the redox current due to their high surface area. However, it should be pointed out that an increase in surface area did not necessarily lead to an amplified current.42 This was demonstrated by Szamocki et al. that, for redox couples with fast charge-transfer kinetics, the molecules did not have enough time to diffuse to the inner surface, and thus using a three-dimensional macroporous structure would not cause any increase in current;23 i.e., although three-dimensional macroporous structure might have a high surface area, the surface area available was limited under such conditions. Actually, in the present work of DA detection, the two-dimensional cavity architecture might have a greater effect on current amplification than that of three-dimensional macroporous gold film electrodes, because the oxidation of DA on the surface of Au electrode is a diffusion controlled process (as discussed below). For the same reason, in this work, we chose those electrodes with semispherical gold cavity for DA detection. However, the surface area of the cavity array thin film increased two-fold compared with that of the flat one, i.e., the higher current enhancement of the GCA electrode may not be simply explained by the increase in surface area.
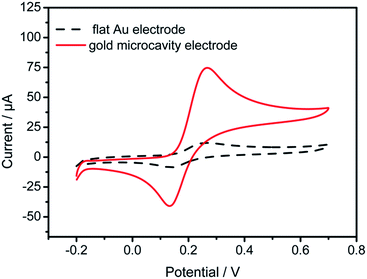 |
| Fig. 2 CVs obtained at the bare Au and GCA electrode in 0.1 M PBS (pH 7.0) containing 0.5 mM DA. Scan rate: 50 mV s−1. | |
Fig. 3 shows the X-ray diffraction (XRD) pattern of the obtained gold cavities, which exhibit characteristic diffraction peaks of metallic Au with the face-centered cubic structure (Joint Committee on Powder Diffraction Standards (JCPDS) file: 04-0784, the peaks marked with asterisks result from the bulk ITO electrode).43,44 Moreover, the intensity ratio between the [200] and [111] diffraction peaks for the GCA electrode was 0.04, which was much lower than that in the standard file (0.37). This indicates that the electrochemically deposited gold nanoparticles grew in a favorable manner on the [111] crystal facet. It was suggested that metallic Au with an enriched [111] have a specific catalytic activity to some electrochemical reactions. For example, Pineda and coworkers reported the systematical research on Au(111) electrodes, and demonstrated that metallic Au with an enriched [111] facet have some specific electrochemical properties.45,46 More recently, the same group experimentally verified that AuNPs|ODT-SAM|Au(111) ensembles could efficiently promote electron transfer (ET) across an ODT insulating monolayer, which opened up a new way of building fast and sensitive electrochemical sensors.46 Moreover, the adsorption of OH− at the (111) facet of the gold particles surface may play a critical role in mediating electrocatalytic oxidation.47 We think that, in the present work, the formation of negatively charged incipient oxide species would be more facile at the surface of the gold cavity structures, resulting in the enhanced current levels for electrooxidation of DA. However, further research must be carried out to confirm this.
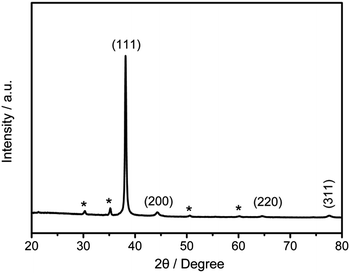 |
| Fig. 3 XRD pattern of the obtained gold cavity array, and the peaks for ITO are marked with *. | |
It has to be noted that even at the GCA electrode, the electrochemical process for the oxidation of 0.5 mM DA is still irreversible (considering that n × ΔEp = 2 × 140 mV > 200 mV; n = 2, the number of electrons transferred).48 This could be caused by the adsorption of the large quantities of producing dopaminequinone on the electrode surface, resulting in a sluggish electron transfer.49,50 When the concentration of DA decreased to 0.05 mM, the CV response showed a couple of quasi-reversible redox peaks with a peak separation of 65 mV, and the oxidation and reduction peak potentials were located at 0.227 V and 0.162 V, respectively (see Fig. S3, ESI†). The result might indicate that, at lower concentration ranges, the electron transfer between DA and Au surface was more facile at the GCA electrode.
3.3. Poly(metanilic acid) decorated GCA electrodes
As other authors have previously observed, the oxidized product of DA is apt to be adsorbed on the electrode surface, resulting in a decrease of current response during continuous measurement. The reproducibility of this GCA electrode was tested. Fig. S4 (see ESI†) displays the CVs recorded at the GCA electrode in a 0.5 mM DA solution for five cycles of consecutive detection. As can be seen, the redox currents showed an obvious decrease with increasing scan times, especially between the first and the second cycle. Moreover, the values of the oxidation and reduction peak separation, ΔEp, gradually increased. These observations indicated that the electrode surface was subjected to the contamination from the oxidation product of DA.
To alleviate the fouling of the electrode surface by the oxidation products, a poly(metanilic acid) thin film was electropolymerized on the surface of the GCA electrode. Fig. 4a shows the typical SEM image of the poly(metanilic acid) modified GCA surface (for clarity, we chose a GCA electrode with a relative small depth for visual observation of the polymer thin film). Comparing Fig. 4a with the SEM images in Fig. 1b–f, the surface of the former was covered by a dense and compact film, which is particularly clear in the inset of Fig. 4a. The obvious differences in the surface morphologies confirmed that the GCA electrode was coated by poly(metanilic acid). Because the –SO3H group is immobilized irreversibly in the chain of the conducting polymer, the electroactivity of the film was very stable over a wide pH-range, and had a reproducibility to DA detection. The reproducibility and stability of this poly(metanilic acid) modified GCA electrode were also tested. The oxidation peak currents in a 2 mM DA solution were measured for twenty cycles of consecutive detection using CV technique. As shown in Fig. 4b, the oxidation current is almost unchanged during the scanning process, and only a 4.5% decrease appears after the completion of the twentieth cycle. The good reproducibility of this electrode could be associated with closely arranged poly(metanilic acid) thin film occupying most of the Au surface, making it difficult for the adsorption of unwanted oxidized product of DA. For the five different modified electrodes, the RSD was 8.6%. The modified electrode was stored at 4 °C over 30 days, and 92% of its original peak current was maintained. These results showed excellent stability of this electrode towards the DA oxidation and its excellent antifouling property against the oxidation products.
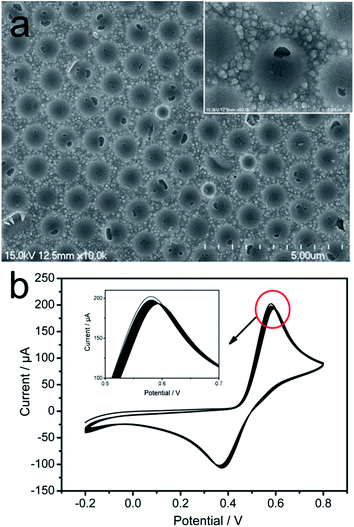 |
| Fig. 4 (a) Typical SEM image of the poly(metanilic acid) thin film modified GCA electrode. (b) CV evolutions at the poly(metanilic acid) modified GCA electrode in 2 mM DA for 20 times of consecutive scanning. | |
3.4. Effect of scan rate
In order to further investigate the electrochemical behavior of DA at this poly(metanilic acid) modified GCA electrode, CVs in 0.1 M PBS containing 50 μM DA at various scan rates ranging from 25 mV s−1 to 1 V s−1 were shown in Fig. 5a. As can be seen, the anodic and cathodic peak currents of DA increased simultaneously with increasing scan rate. Fig. 5b shows the linear dependence of the redox peak currents on the square root of scan rates. The linear regression equations are y = (33.4136 ± 0.3697)x − (0.9846 ± 0.0582) (r2 = 0.9970) for the anodic peak current and y = −(30.8892 ± 0.3178)x + (2.0642 ± 0.1038) (r2 = 0.9984) for the cathodic peak current, suggesting that the electrochemical oxidation at the modified electrode is controlled by a diffusion process. In addition, only a slight increase in the separation of the cathodic and anodic peak potentials ranging from 25 mV s−1 to 0.5 V s−1 was observed, indicating a fast electron transfer rate for the electrochemical oxidation of DA at the surface of the modified GCA electrode.
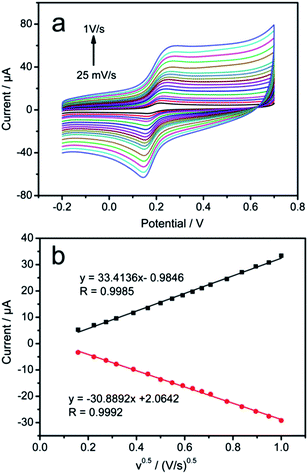 |
| Fig. 5 CV evolutions of Au/Fe6 obtained in 0.1 M PBS (pH 7.0) containing 50 μM DA at scan rates from 25 mV s−1 to 5 V s−1 (from inner to outer curves: 0.025, 0.05, 0.075, 0.1, 0.15, 0.2, 0.25, 0.3, 0.35, 0.4, 0.45, 0.5, 0.6, 0.7, 0.8, 0.9, 1.0 V s−1), (b) the plot of current (Ip) vs. square root of scan rate. | |
The diffusion coefficient of DA was calculated according to the Randles–Sevcik equation,51
where
Ip (A) is the oxidation peak current,
A is the geometric surface area of the electrode (π
r2 = 3.14(0.5/2)
2 = 0.19625 cm
2),
D is the diffusion coefficient (cm
2 s
−1),
C0 is the concentration of DA (mol mL
−1),
n represents the number of electrons transferred, and
ν (V s
−1) is the scan rate. By substituting the necessary values into the equation, the
D value was calculated to be 1.0 × 10
−5 cm
2 s
−1. This
D value agrees well with the reported value for the Nafion carbon-coated iron nanoparticles–chitosan composite film modified electrode
52 and is higher compared to some other electrodes reported in the literature,
19,53,54 suggesting a faster DA diffusion for the modified electrode in this study.
Taken together, the better electrochemical response exhibited at the poly(metanilic acid) modified GCA electrode is ascribed to the combination of the larger surface area of the GCA, excellent anti-fouling property of the poly(metanilic acid) thin film against the oxidation products, easy diffusion of dopamine through the thin film to the surface of GCA, and the possibility of the electrostatic interaction between the negatively charged sulfonic group and the positively charged DA (DA is positively charged at pH 7.4 or in a neutral environment).9 Thus, the composite film with unique structural and electrochemical properties could be a promising candidate for the construction of sensitive and selective sensors.
3.5. Electrochemical determination of DA
Although the surface area of the GCA electrode was enlarged, with a concomitant increase in faradaic current, the capacitive current also showed an increase, and thus when using cyclic voltammetry, the overall signal-to-noise ratio would not be improved. In this work, we applied the SWV technique for the quantitative determination of DA, because it can minimize the influence of the capacitive current and render a real improvement in sensitivity and LODs. Fig. 6 shows parts of the SWV responses of DA with different concentrations at the poly(metanilic acid) modified GCA electrode. The oxidation peak currents increased linearly with the concentrations of DA ranging from 0.2 to 100 μM, and the linear regression equation is expressed as y = (0.3443 ± 0.0032)x − (1.2150 ± 0.0749) (r2 = 0.9988). However, when the concentrations of DA were higher than 100 μM, the responses of the SWV currents gradually deviated from the calibration curve. The limit of detection was calculated to be 0.08 μM, using the IUPAC definition LOD = 3sb/q, with sb being the standard deviation of the blank signal, and q the slope of the calibration curve. The lower detection limit and wider linear range are due to the excellent electrochemical activity of the composite film towards DA.
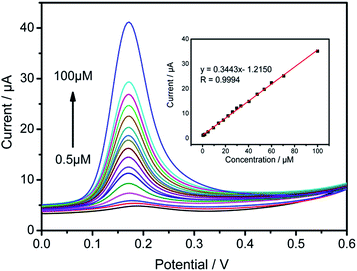 |
| Fig. 6 Typical SWVs of the poly(metanilic acid) modified GCA electrode in 0.1 M PBS (pH 7.0) solution containing increasing concentrations of DA (0.5, 1.0, 2.0, 4.0, 6.0, 8.0, 10.0, 15.0, 20.0, 25.0, 30.0, 35.0, 40.0, 45.0, 50.0, 60.0, 70.0, 80.0, 90.0, 100.0 μM). The inset is the linear relationship between DA peak current and concentration. | |
It is well known that ascorbic acid (AA) and/or uric acid (UA) usually have a higher concentration than DA in biological samples and are oxidized at a similar potential, resulting in an overlapped voltammetric response.17 The oxidation of co-existing DA and UA at the poly(metanilic acid) modified GCA electrode was carried out by CV and SWV. Fig. S5 (see ESI†) shows the CVs for the oxidation of 0.5 mM DA, 1 mM UA at the GCA electrode, and a mixture of 50 μM DA + 1 mM UA at the poly(metanilic acid) modified GCA electrode. As observed, at the GCA electrode, the oxidation peak potentials for DA and UA were located at 0.32 and 0.45 V, respectively, with a certain degree of overlap between these two peaks. Compared to the individual detection of DA and UA, the peak potential of DA in the mixture showed a 72 mV negative shift, whereas for UA, the peak potential positively shifted ∼50 mV, the peak potential difference increased to 270 mV. Therefore, at the poly(metanilic acid) modified electrode, the electrochemical signals of DA and UA can be well distinguished. This electrochemical behavior is similar with an earlier report based on single-walled carbon nanotube modified electrodes,46 and graphene/size-selected Pt nanocomposite modified GC electrodes.55 Fig. 7a shows part of the SWV curves at the modified electrode obtained for the oxidation of various concentrations of DA in the presence of 0.2 mM UA in PBS solution. As observed, the oxidation peak currents of DA at + 0.17 V increased with the increasing DA concentration from 2 to 25 μM in the presence of UA, while the oxidation peak currents of UA at + 0.46 V remained unchanged. The signal of DA can be clearly detected, even when the concentration of UA is 500 times higher than that of DA (figure not shown). Fig. 7b shows the SWV obtained at the modified electrode for the different concentrations of UA in the presence of 20 μM DA. The UA peak current increased with increasing concentration of UA from 2 to 100 μM and the DA peaks were essentially unchanged. These results verified that DA and UA could be individually and simultaneously detected at the modified electrode with high sensitivity and selectivity. The interference of AA in the electrochemical detection of DA was also investigated. The SWVs of standard additions of AA into 0.1 M pH 7.0 PBS solution containing 25 μM DA are shown in Fig. S6.† As observed, the electrochemical response of AA was less sensitive than those of DA and UA, and showed a nonlinear increase with the increasing AA concentration from 0.05 to 0.5 mM, while the peak currents of DA almost remained unchanged. This result indicates that AA did not present an obvious interference in the determination of DA.
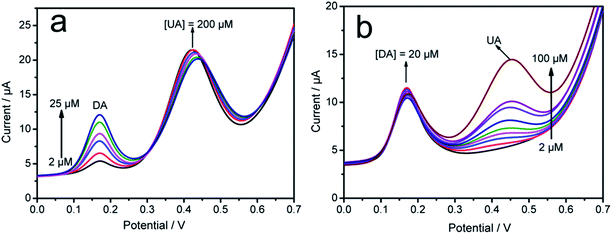 |
| Fig. 7 SWVs of the modified GCA electrode in 0.1 M PBS (pH 7.0) solution containing (a) 0.2 mM UA and increasing concentrations of DA (2.0, 5.0, 10.0, 15.0, 20.0, 25.0 μM), (b) 20.0 μM DA and increasing concentrations of UA (2.0, 5.0, 10.0, 15.0, 20.0, 30.0, 40.0, 50.0, 100.0 μM). | |
3.6. Real sample determination
In order to evaluate the analytical applicability of this poly(metanilic acid) modified GCA electrode for pharmaceutical products, it was tested to detect the concentration of DA in a dopamine hydrochloride injection (specified content of DA is 10 mg mL−1, Shaanxi Jingxi Pharmaceutical Co., Ltd). The injection sample was diluted with PBS, and then spiked with an appropriate amount of DA. The SWV and the calibration curve were used to determine the concentration of each solution; the recovery and RSD are shown in Table 1. The results suggest that the modified electrode may have a promising application in the determination of DA in real samples.
Table 1 Determination of dopamine hydrochloride injection (n = 5)
No. |
Original (μM) |
Added (μM) |
Found (μM) |
RSD (%) |
Recovery (%) |
1 |
10.55 |
0 |
10.61 |
2.87 |
100.57 |
2 |
10.55 |
4.45 |
15.37 |
3.81 |
102.47 |
3 |
10.55 |
9.90 |
20.19 |
2.65 |
98.73 |
4 |
21.10 |
14.78 |
37.26 |
4.16 |
103.85 |
5 |
21.10 |
19.61 |
39.74 |
3.35 |
97.62 |
6 |
21.10 |
24.39 |
43.46 |
5.62 |
95.53 |
4. Conclusion
In conclusion, we have reported the fabrication of a two-dimensional gold cavity array electrode via a multi-current pulse electrodeposition method using closely packed PS spheres as a template. The thickness of the as-prepared gold cavity can be conveniently modulated by controlling the charge passed during the deposition process. Next, poly(metanilic acid) has been electropolymerized on the gold cavity array surface to form the final poly(metanilic acid) thin film modified GCA electrode. Different electrochemical methods have been performed herein to investigate the possibility of utilizing this poly(metanilic acid) modified GCA electrode for the electrochemical detection of DA. From the results, we have found that the proposed electrode exhibits a faster electron transfer rate and better electrochemical oxidation abilities towards DA than the bare Au electrode. The detection limit of DA could be as low as 8.0 × 10−8 M with a linear range from 0.2 to 100 μM. Moreover, a trace amount of DA can be well distinguished from the interfering substances UA based on the respective oxidation potentials, thus realizing the simultaneous detection of DA and UA in their binary mixtures. The high sensitivity, good reproducibility and long-term stability of this poly(metanilic acid) modified GCA electrode enable itself to be a good sensor for the detection of DA in low concentration.
Acknowledgements
Financial support from the Nature Science Foundation of China (nos 21173122, 21177067), the Nature Science Foundation of Jiangsu Province (no. BK20131200), the Natural Science Foundation of the Jiangsu Higher Education Institutions of China (14KJB150018), and the Innovative Training Program for undergraduates (201410304015Z) are gratefully acknowledged.
Notes and references
- R. M. Wightman, L. J. May and A. C. Michael, Anal. Chem., 1988, 60, 769A CrossRef CAS.
- B. J. Venton and R. M. Wightman, Anal. Chem., 2003, 75, 414A CrossRef CAS.
- M. R. Moghadam, S. Dadfarnia, A. M. H. Shabani and P. Shahbazikhah, Anal. Biochem., 2011, 410, 289 CrossRef CAS PubMed.
- A. Abbaspour, A. Khajehzadeh and A. Ghaffarinejad, Analyst, 2009, 134, 1692 RSC.
- Z. Ebru Seckin and M. Volkan, Anal. Chim. Acta, 2005, 547, 104 CrossRef CAS PubMed.
- S. Md, S. Haque, M. Fazil, M. Kumar, S. Baboota, J. K. Sahni and J. Ali, Expert Opin. Drug Delivery, 2014, 11, 827 CrossRef CAS PubMed.
- R. M. Wightman, L. J. May and A. C. Michael, Anal. Chem., 1988, 60, 769A CrossRef CAS.
- F. J. Shang, L. Zhou, K. A. Mahmoud, S. Hrapovic, Y. L. Liu, H. A. Moynihan, J. D. Glennon and J. H. T. Luong, Anal. Chem., 2009, 81, 4089 CrossRef CAS PubMed.
- R. K. Shervedani, S. M. Siadat-Barzoki and M. Bagherzadeh, Electroanalysis, 2010, 22, 969 CrossRef CAS.
- O. O. Fashedemi and K. I. Ozoemena, Sens. Actuators, B, 2011, 160, 7 CrossRef CAS PubMed.
- M. Ahn and J. Kim, J. Electroanal. Chem., 2012, 683, 75 CrossRef CAS PubMed.
- K. Jackowska and P. Krysinski, Anal. Bioanal. Chem., 2013, 405, 3753 CrossRef CAS PubMed.
- M. Hasanzadeh, N. Shadjou and E. Omidinia, J. Neurosci. Methods, 2013, 219, 52 CrossRef CAS PubMed.
- L. Yang, S. Liu, Q. Zhang and F. Li, Talanta, 2012, 89, 136 CrossRef CAS PubMed.
- N. Xiao and B. J. Venton, Anal. Chem., 2012, 84, 7816 CrossRef CAS PubMed.
- C.-L. Sun, C.-T. Chang, H.-H. Lee, J. Zhou, J. Wang, T.-K. Sham and W.-F. Pong, ACS Nano, 2011, 5, 7788 CrossRef CAS PubMed.
- M. Anu Prathap and R. Srivastava, Sens. Actuators, B, 2013, 177, 239 CrossRef CAS PubMed.
- F. Gao, H. Qu, Y. Duan, J. Wang, X. Song, T. Ji, L. Cao, G. Nie and S. Sun, RSC Adv., 2014, 4, 6657 RSC.
- G.-R. Xu, M.-L. Xu, J.-M. Zhang, S. Kim and Z.-U. Bae, Bioelectrochemistry, 2008, 72, 87 CrossRef CAS PubMed.
- H. Zhang, G. Duan, G. Liu, Y. Li, X. Xu, Z. Dai, J. Wang and W. Cai, Nanoscale, 2013, 5, 2460 RSC.
- A. Stein, B. E. Wilson and S. G. Rudisill, Chem. Soc. Rev., 2013, 42, 2763 RSC.
- A. Walcarius, TrAC, Trends Anal. Chem., 2012, 38, 79 CrossRef CAS PubMed.
- R. Szamocki, S. Reculusa, S. Ravaine, P. N. Bartlett, A. Kuhn and R. Hempelmann, Angew. Chem., Int. Ed., 2006, 45, 1317 CrossRef CAS PubMed.
- R. Szamocki, A. Velichko, C. Holzapfel, F. Mücklich, S. Ravaine, P. Garrigue, N. Sojic, R. Hempelmann and A. Kuhn, Anal. Chem., 2007, 79, 533 CrossRef CAS PubMed.
- C. H. Wang, C. Yang, Y. Y. Song, W. Gao and X. H. Xia, Adv. Funct. Mater., 2005, 15, 1267 CrossRef CAS.
- D. Gornowich and G. Blanchard, J. Phys. Chem. C, 2012, 116, 12165 CAS.
- S. Tian, Q. Zhou, Z. Gu, X. Gu, L. Zhao, Y. Li and J. Zheng, Talanta, 2013, 107, 324 CrossRef CAS PubMed.
- M. R. Jones, K. D. Osberg, R. J. Macfarlane, M. R. Langille and C. A. Mirkin, Chem. Rev., 2011, 111, 3736 CrossRef CAS PubMed.
- G. Hong, C. Li and Q. Limin, Adv. Funct. Mater., 2010, 20, 3774 CrossRef CAS.
- P. N. Bartlett, J. J. Baumberg, S. Coyle and M. E. Abdelsalam, Faraday Discuss., 2004, 125, 117 RSC.
- F. Sun, W. Cai, Y. Li, B. Cao, Y. Lei and L. Zhang, Adv. Funct. Mater., 2004, 14, 283 CrossRef CAS.
- P. V. Braun and P. Wiltzius, Nature, 1999, 402, 603 CrossRef CAS PubMed.
- P. N. Bartlett, J. J. Baumberg, P. R. Birkin, M. A. Ghanem and M. C. Netti, Chem. Mater., 2002, 14, 2199 CrossRef CAS.
- P. Manivel, M. Dhakshnamoorthy, A. Balamurugan, N. Ponpandian, D. Mangalaraj and C. Viswanathan, RSC Adv., 2013, 3, 14428 RSC.
- T. Qian, C. Yu, X. Zhou, P. Ma, S. Wu, L. Xu and J. Shen, Biosens. Bioelectron., 2014, 58, 237 CrossRef CAS PubMed.
- B. Massoumi, S. Fathalipour, A. Massoudi, M. Hassanzadeh and A. A. Entezami, J. Appl. Polym. Sci., 2013, 130, 2780 CrossRef CAS.
- Y. Gao, D. Shan, F. Cao, J. Gong, X. Li, H.-y. Ma, Z.-m. Su and L.-y. Qu, J. Phys. Chem. C, 2009, 113, 15175 CAS.
- P. Lacroix-Desmazes and J. Guillot, J. Polym. Sci., Part B: Polym. Phys., 1998, 36, 325 CrossRef CAS.
- Y. Li, W. Cai and G. Duan, Chem. Mater., 2007, 20, 615 CrossRef.
- G. Zotti, S. Cattarin and N. Comisso, J. Electroanal. Chem. Interfacial Electrochem., 1987, 235, 259 CrossRef CAS.
- X. Gu, Y. Yan, G. Jiang, J. Adkins, J. Shi, G. Jiang and S. Tian, Anal. Bioanal. Chem., 2014, 406, 1885 CrossRef CAS PubMed.
- A. Walcarius and A. Kuhn, TrAC, Trends Anal. Chem., 2008, 27, 593 CrossRef CAS PubMed.
- Z. Li, V. Ravaine, S. Ravaine, P. Garrigue and A. Kuhn, Adv. Funct. Mater., 2007, 17, 618 CrossRef CAS.
- C. Quintana, P. Atienzar, G. Budroni, L. Mora, L. Hernández, H. García and A. Corma, Thin Solid Films, 2010, 519, 487 CrossRef CAS PubMed.
- Z. González-Granados, G. Sánchez-Obrero, R. Madueño, J. M. Sevilla, M. Blázquez and T. Pineda, J. Phys. Chem. C, 2013, 117, 24307 Search PubMed.
- D. García Raya, C. Silien, M. Blázquez, T. Pineda and R. Madueño, J. Phys. Chem. C, 2014, 118, 14617 Search PubMed.
- B. Lertanantawong, A. P. O'Mullane, W. Surareungchai, M. Somasundrum, L. D. Burke and A. M. Bond, Langmuir, 2008, 24, 2856 CrossRef CAS PubMed.
- Y. Li, J. Du, J. Yang, D. Liu and X. Lu, Colloids Surf., B, 2012, 97, 32 CrossRef CAS PubMed.
- T. E. Young and B. W. Babbitt, J. Org. Chem., 1983, 48, 562 CrossRef CAS.
- F. Zhang and G. Dryhurst, J. Electroanal. Chem., 1995, 398, 117 CrossRef.
- A. J. Bard and L. R. Faulkner, Electrochemical methods fundamentals and applications, Wiley and Sons, Hoboken, 2001 Search PubMed.
- G. S. Lai, H. L. Zhang and D. Y. Han, Microchim. Acta, 2008, 160, 233 CrossRef CAS.
- F. Gao, X. Cai, X. Wang, C. Gao, S. Liu, F. Gao and Q. Wang, Sens. Actuators, B, 2013, 186, 380 CrossRef CAS PubMed.
- S. Liu, J. Yan, G. He, D. Zhong, J. Chen, L. Shi, X. Zhou and H. Jiang, J. Electroanal. Chem., 2012, 672, 40 CrossRef CAS PubMed.
- C.-L. Sun, H.-H. Lee, J.-M. Yang and C.-C. Wu, Biosens. Bioelectron., 2011, 26, 3450 CrossRef CAS PubMed.
Footnote |
† Electronic supplementary information (ESI) available: Fig. S1–S6. See DOI: 10.1039/c4ra07649d |
|
This journal is © The Royal Society of Chemistry 2014 |
Click here to see how this site uses Cookies. View our privacy policy here.