DOI:
10.1039/C4RA09171J
(Paper)
RSC Adv., 2014,
4, 54372-54381
The synthesis and characterization of carboxybetaine functionalized polysiloxanes for the preparation of anti-fouling surfaces†
Received
24th August 2014
, Accepted 8th October 2014
First published on 8th October 2014
Abstract
Nonspecific protein adsorption and bacterial adhesion have caused serious problems in biomedical devices, marine engineering, membrane separation and many other areas. In order to develop a water-soluble bio-adhesion resistant material, a series of novel zwitterionic polymers, carboxybetaine functionalized polysiloxanes (PDMS-g-CB), were synthesized via a three-step procedure. FT-IR and NMR were used to characterize the chemical structures of PDMS, and the solution properties (i.e. CMC) and biological characteristics were investigated. The high biocompatibility of PDMS-g-CB was demonstrated by hemolysis assay, skin irritation evaluation and acute oral toxicity testing. To evaluate the anti-fouling and hydrophilic properties of PDMS-g-CB, it was blended with PDMS elastomer to form films (b-PDMS). Water contact angle and ATR-FTIR measurements revealed that the hydrophobic PDMS surfaces were converted to hydrophilic surfaces after the introduction of PDMS-g-CB. The anti-fouling properties of b-PDMS were evaluated by protein adsorption and bacterial adhesion tests. Results showed that the amount of adsorbed protein and bacteria adhesion were significantly reduced compared with the untreated PDMS. These findings suggest that PDMS-g-CB is a biocompatible and promising material for the construction of anti-fouling surfaces.
1 Introduction
Over the past few decades, chemical pesticides have played a pivotal role for pest management in agriculture. Although pesticides have proved to be reliable and effective pest control agents, they also cause undesirable ecological and economic consequences in our lives, such as insecticide resistance, outbreaks of secondary pests, increased health risks to humans and livestock, and destruction of the natural environment.1,2 Therefore, a growing emphasis is being laid on reducing the use of chemical pesticides. To overcome these problems, continuous efforts have been made to develop a harmless way for pest management, which has a little adverse impact on human health and is harmless to the environment.3,4
It has been observed that the effective adsorption of protein on the material surface is fundamental to the attachment, survival and reproduction of phytophagous insects on the plant surface.5 If this process could be regulated by changing the micro-environment of plant surfaces, we may be able to control the pests in a harmless way. Thus, the construction of low-fouling or non-fouling surfaces is highly desirable. For example, according to the normal procedure of phytophagous insect oviposition, insects, such as Spodoptera exigua (beet armyworm), would secrete mucus on the abaxial leaf surface to help the adhesion of eggs, in which the mucus mainly contains water, protein and a small amount of carbohydrate.6 We assume that if a non-toxic and protein adsorption resistant leaf surface was achieved by spraying with a polymeric coating, the adhesion of eggs on the leaf surface could be damaged or weakened, and the sprayed leaf surface would be an unsuitable environment for the pest to secrete, and thus pest control could be partly achieved without killing or deterring the pest.
Moreover, there is a significant need for the development of anti-fouling materials to resist nonspecific protein adsorption and bacteria adhesion for implantable biomaterials, biosensors, ocean engineering and membrane separation application.7,8
To date, few materials can be used for construction of anti-fouling or non-fouling surfaces, which can resist nonspecific protein adsorption effectively. Polyethylene glycol (PEG) and oligoethylene glycol (OEG) based materials are the most widely used anti-fouling materials.9–11 Studies show that PEG or OEG modified surfaces can reduce protein adsorption and cell adhesion to a large scale. Steric exclusion effect and surface hydration are considered as key factors to the antifouling properties for PEG and OEG based materials, respectively.12–14 However, PEG and OEG based materials belong to the polyether group, which is susceptible to oxidation damage, especially in the presence of oxygen and transition metal ions.15,16 Recently, zwitterionic polymers, such as phosphorylcholine (PC), polysulfobetaine (pSB) and polycarboxybetaine (pCB), have attracted considerable attention due to their super low-fouling characteristics and relative stability.17–19 Jiang and co-workers have prepared several zwitterionic poly(carboxybetaine methacrylate) (pCBMA) modified surfaces that are highly resistant to nonspecific protein adsorption and bacterial adhesion.20–22 It is believed that zwitterionic polymer modified surfaces could form a hydration layer near the surface via ionic solvation and hydrogen bonding, which would prevent the adsorption of protein by the hydration barrier.23,24
In this study, in order to obtain a water-soluble, non-toxic and biocompatible polymer with excellent anti-fouling and film forming properties, we designed a novel carboxybetaine functionalized polymer (PDMS-g-CB). The polymer consisted of a PDMS backbone and carboxybetaine pendant-side. The PDMS backbone endows the polymer with extremely low glass transition temperature, low surface tension, optical transparency, permeability to gas, durability, biocompatibility, and good film forming properties, while the introduction of carboxybetaine groups offers high resistance to protein adsorption and bacterial adhesion. In addition, we could tailor the hydrophilicity and anti-fouling properties by adjusting the ratio of the carboxybetaine pendant-side. The biocompatibility (i.e., skin irritation, acute toxicity and hemolysis activity) and solution properties of PDMS-g-CB (i.e., critical micelle concentration, CMC) were investigated. Due to the high water solubility of PDMS-g-CB, it is difficult to evaluate the antifouling properties of PDMS-g-CB directly, and therefore they were blended with liquid silicones to give a PDMS-g-CB/PDMS blend film (b-PDMS) and to examine the anti-fouling properties, in which PDMS-g-CB could be fixed onto the surface of the PDMS elastomer. Subsequently, the anti-fouling properties of PDMS-g-CB (resistance to protein adsorption and bacterial adhesion) were demonstrated by b-PDMS indirectly.
2 Experimental
2.1 Materials
Octamethylcyclotetrasiloxane (D4, > 99.5%, Dow Corning, USA), 1,3,5,7-tetramethylcyclo-tetrasiloxane (DH4, > 99%, Hangzhou Silong Material Technology Co., Ltd, China) and hexamethyldisiloxane (MM, > 99%, Dow Corning, USA) were dried before use. N,N-Dimethylallylamino (DMAA, > 99%, Haining City Huangshan Chemical Industry Co., Ltd, China) was purified by distillation before use. Platinum (0)-1,3-divinyl-1,1,3,3-tetramethyl-disiloxane complex solution (Karstedt's catalyst solution, Pt ∼ 2%, Aladdin Reagent Co. Ltd, China) was used as the catalyst of hydrosilylation. Purolite® CT175 (Purolite (China) Co. Ltd) is a clay containing sulphonic acid, it was used as received. Vinyl functionalized polysiloxanes (PMVS, Vi ∼ 0.35%, Dow Corning, USA) and sodium chloroacetate (SC, Aladdin Reagent Co., Ltd, China) were used as received. Other solvents, such as isopropanol, were used without any pretreatment. Escherichia coli (E. coli) was kindly supplied by the College of Natural Resources & Environment, South China Agricultural University.
2.2 Synthesis of PDMS-g-CB
In this paper, a series of carboxybetaine functionalized polysiloxanes (PDMS-g-CB) were synthesized via a three-step procedure (Scheme 1).
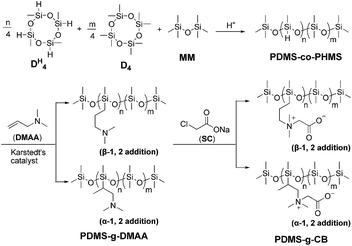 |
| Scheme 1 The three-step synthesis route of PDMS-g-CB. | |
First of all, various hydrogen-containing polysiloxanes (PDMS-co-PHMS) with a relatively narrow molecular weight distribution were synthesized by the ring-opening polymerization of D4 and DH4, and then N,N-dimethylallylamino (DMAA) was grafted to PDMS-co-PHMS via hydrosilylation reaction in the presence of Karstedt's catalyst to obtain PDMS-g-DMAA. Finally, carboxybetaine functionalized polysiloxanes (PDMS-g-CB) were prepared by subsequent quaternization reaction. (A detailed description can be found in the ESI: Part S1.†)
2.3 Preparation of PDMS-g-CB blended PDMS film (b-PDMS)
To evaluate the hydrophilic properties and anti-fouling properties of PDMS-g-CB, a series of PDMS-g-CB were embedded into polydimethylsiloxanes elastomer (PDMS) by blending. Typically, vinyl functionalized polysiloxanes were mixed thoroughly with PDMS-g-CB/methanol solution (50 wt%), then hydrogen containing polysiloxanes (H wt% = 0.145 wt%, lab-made) and certain amount of Karstedt's catalyst were added. The homogeneous solutions were poured into a clean moulds coated with tetrafluoroethylene, then degassed under vacuum for 30 min. Films were cured at room temperature for 1 day. After curing, the b-PDMS films were peeled from the moulds carefully and cut into circular pieces (10 mm in diameter).
2.4 Structure characterization
Fourier transform infrared (FT-IR) spectra were recorded using a VERTEX-70 spectrometer (Brucker Instrument Corp., Germany) at resolution of 4 cm−1, samples were coated on KBr plates and dried under the infrared lamp. The measurements were carried out over 400–4000 cm−1 range at room temperature. Attenuated total reflection Fourier transform infrared (ATR-FTIR) spectra were measured on VERTEX-70 spectrometer equipped with an ATR accessory, 64 scans were collected for each sample.
1H-NMR and 13C-NMR spectra were collected with a Brucker Avance III-400 (400 MHz) Fourier digital NMR spectrometer (Brucker Instrument Corp., Germany) at room temperature using CDCl3 or D2O as the solvent.
Gel permeation chromatography (GPC) was performed on an Elite EC2000 GPC apparatus (Dalian, China) equipped with a Shodex K-G guard column and a Shodex K-804L chromatographic column. Detection was achieved using a refractive index detector, and the samples were analyzed at 30 °C using CHCl3 as the eluent at a flow rate of 1 mL min−1. The instrument was calibrated using low polydisperse polystyrene standards.
The static water contact angles of PDMS-g-CB and b-PDMS surfaces were measured by a contact angle goniometer (JY-82, China) at room temperature. One drop of water (10 μL) was placed onto the surface with a proper pipette, and the water droplet was observed and recorded with an optical microscope. More than three random positions were measured for each sample.
2.5 Determination of critical micelle concentration (CMC) of PDMS-g-CB
The CMC of PDMS-g-CB was determined by two commonly used methods, namely, fluorescence probes and electrical conductivity methods. (A detailed description can be found in the ESI: Part S2.†)
2.6 Antibacterial activity assessment
The antibacterial activities of PDMS-g-CB in different concentrations (15, 30 and 60 mg mL−1) were evaluated by measuring the inhibition zones against Escherichia coli (E. coli), using the Agar well (Oxford cup) diffusion method. Briefly, 20 mL sterile nutrient agar was poured into sterile Petri dishes with four sterile Oxford cups (inner diameter 6 mm), and 2 mL bacterial (E. coli) suspensions (OD600 ≈ 1.0) were added into the dishes after the agar coagulated. Then, PDMS-g-CB solutions with varying concentrations, positive control (25 μg mL−1 of chloramphenicol) and negative control (sterile physiological saline) were added into each Oxford cup, respectively. All the dishes were incubated at 37 °C for 24 h, and the inhibition zones were measured and recorded.
2.7 Skin irritation testing
Evaluation of the potential for PDMS-g-CB to cause skin irritation was conducted on rabbits in vivo according to ISO 10993-10: 2002. (A detailed description can be found in the ESI: Part S3.†).
2.8 Acute oral toxicity testing
In order to assess the toxicity of PDMS-g-CB, the acute oral toxicity (LD50 as the index) was determined using NIH mice (22–25 g), according to the OECD Acute Oral Toxicity Test Guideline 425 procedure (OECD TG 425). (A detailed description can be found in the ESI: Part S4.†).
2.9 Hemolysis assay
Hemolysis assay was performed with fresh rabbit blood obtained from a New Zealand white rabbit, according to ISO 10993-4: 2002. (A detailed description can be found in the ESI: Part S5.†).
2.10 Protein adsorption
Bovine serum albumin (BSA) was used as the model protein for evaluation of protein adsorption. To measure the amounts of protein adsorbed onto PDMS and b-PDMS surfaces, the films were immersed in 10 mL protein solutions (BSA, 4 mg mL−1) and incubated at 37 °C for 60 min, then the films were removed from the BSA solution and rinsed with PBS three times. Subsequently, the films were sonicated for 120 min in PBS to detach the protein adsorbed onto native PDMS and b-PDMS surfaces, then the solution was collected and the amount of protein was determined by the Micro BCA (bicinchoninic acid) Protocol (Micro BCA Protein Assay Kit, Sangon Biotech Co., Ltd., Shanghai, China).26,27 Afterwards, the amount of adsorbed protein was calculated according to the standard protein curve, which was measured with different concentrations of protein standard.
2.11 Bacterial adhesion
Escherichia coli (E. coli) was used to investigate the bacterial adhesion behaviours on the surface of native PDMS and b-PDMS films. E. coli was incubated in a broth medium (containing 3.0 mg mL−1 beef extract, 10.0 mg mL−1 peptone and 5.0 mg mL−1 sodium chloride) at 37 °C for 24 h. The bacterial concentration was measured by a spectrophotometer at 540 nm (OD540) with the assumption that the OD540 = 1.0 corresponds to a bacterial concentration of approximately 109 colony-forming units (CFU) per millilitre.28 For the antibacterial assay, the E. coli containing broth was washed and re-suspended in PBS to obtain a bacterial suspension, which has a concentration of 108 CFU mL−1. The native PDMS and modified PDMS (b-PDMS) were sterilized with ultraviolet rays (each side for 30 min), and then immersed in the bacterial suspension in a sterile flask, which was cultured at 37 °C for 20 h. Afterwards, the films were removed and rinsed three times for 10 min in sterile PBS, and bacteria adhered onto the surface were immobilized with 2.5% glutaraldehyde solution for 6 h at 4 °C. Then, the films were washed with sterile PBS three times, and dehydrated step by step in a series of ethanol–water mixtures (30%, 50%, 70%, 80%, 95% and 100% by volume, 20 min for each). Finally, the dried films were coated with gold and observed with a scanning electron microscope (Zeiss EVO 18, Germany).
3 Results and discussion
3.1 Synthesis and characterization PDMS-g-CB
A series of PDMS-g-CB with different carboxybetaine grafting ratios were prepared via a three-step process (ring-opening polymerization, hydrosilylation reaction and quaternization reaction) as described in Scheme 1. The chemical structures of the products at each stage were confirmed by FT-IR and 1H-NMR spectroscopy.
It is well known that hydrogen-containing polysiloxanes can be prepared by the ring-opening polymerization (ROP) of cyclosiloxanes or condensation of linear siloxanes,29,30 and researchers found that the hydrogen content and molecular weight of the resulting polymer is more controllable via ROP of cyclosiloxanes. As reported previously,25 PDMS-co-PHMS was synthesized by the cationic ring-opening polymerization of D4, DH4 and MM using strong acid ion exchange resin (Purolite® CT175) as the catalyst. The hydrogen content and molecular weights of PDMS-co-PHMS were controlled by adjusting the mole ratio of D4, DH4 and end-agent (MM). The structures of PDMS-co-PHMS were characterized by FT-IR and 1H-NMR. Representative FT-IR spectra of PDMS-co-PHMS are shown in Fig. 1, and the absorption peaks at 2962, 2158, 1261, 1093, 1010 and 802 cm−1 correspond to the stretching vibrations of C–H, Si–H, Si–CH3 and Si–O–Si, respectively. Moreover, the intensity of Si–H absorption peak increased with the increment of DH units. Fig. 2 shows the classic 1H-NMR spectra of PDMS-co-PHMS. The characteristic signal of Si–H protons appeared at δ = 4.7 ppm, and the signal at δ = 0–0.2 ppm was attributed to the protons in Si–CH3. The molecular weight and hydrogen content of various PDMS-co-PHMS were measured with GPC and 1H-NMR (as shown in Table S1†), which indicated that the molecular weight of PDMS-co-PHMS is close to the theoretical value calculated from the designed molecular formula.
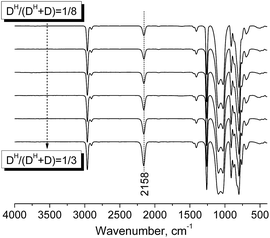 |
| Fig. 1 The FT-IR spectra of PDMS-co-PHMS with varying compositions. | |
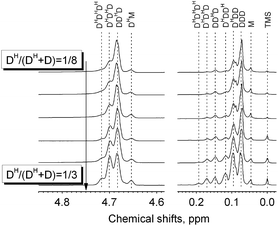 |
| Fig. 2 The 1H-NMR spectra of PDMS-co-PHMS with varying compositions. | |
In the second step of PDMS-g-CB synthesis, tertiary amino functionalized groups were introduced into the siloxanes backbone via the hydrosilylation reaction, which is the most important methodology for the synthesis of organo-functionalized siloxanes in silicone chemistry. Hydrosilylation reaction refers to an addition reaction of Si–H bonds to unsaturated bonds such as C
C, the addition process in principle could occur in two directions to give α-1, 2 and β-1, 2 addition products, as shown in Scheme 2. The course of addition has been proven to depend on the structure of unsaturated monomer, the temperature and the catalyst.31
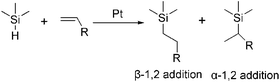 |
| Scheme 2 Two possible hydrosilylation patterns of Si–H containing siloxanes. | |
Herein, PDMS-g-DMAA was synthesized by the hydrosilylation reaction of PDMS-co-PHMS with DMAA in the presence of Karstedt's catalyst. The progress of the hydrosilylation reactions were traced by the variation of the characteristic Si–H absorption at 2158 cm−1 by FT-IR. Fig. 3 represents the FT-IR spectra of PDMS-co-PHMS and PDMS-g-DMAA, in which the Si–H absorption band at 2158 cm−1 and 912 cm−1 disappeared completely, demonstrating the effectiveness of the hydrosilylation reaction, and the absorption bands observed at 2816 cm−1 and 2764 cm−1 are due to the introduction of –N(CH3)2 groups. The completion of the hydrosilylation reaction was confirmed by 1H-NMR spectra (Fig. 4), which presented the disappearance of Si–H group (characteristic signal at δ = 4.7 ppm) and appearance of a series of C–H protons. In addition, the 1H-NMR spectrum of PDMS-g-DMAA indicated that both α-1, 2 addition products and β-1, 2 addition products were obtained in the hydrosilylation of PDMS-co-PHMS and DMAA, because the characteristic signal of methyl protons, which are connected to –CCH2-N(CH3)2 can be observed at about 1.0 ppm (α-1, 2 addition pattern). The two addition patterns of the hydrosilylation reaction could also be confirmed by the 13C-NMR spectrum (Fig. 5). The proportion of α-1, 2 addition products and β-1, 2 addition products calculated from the 1H-NMR spectrum was about 25% and 75%, respectively.
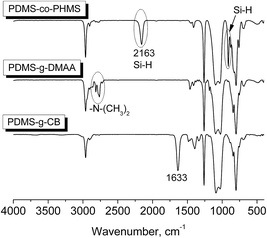 |
| Fig. 3 The FT-IR spectra of PDMS-co-PHMS, PDMS-g-DMAA and PDMS-g-CB. | |
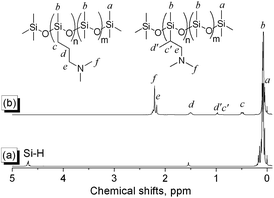 |
| Fig. 4 The 1H-NMR spectra of (a) PDMS-co-PHMS and (b) PDMS-g-DMAA (in CDCl3). | |
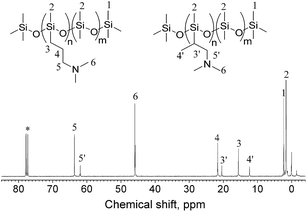 |
| Fig. 5 The 13C-NMR spectrum of PDMS-g-DMAA (in CDCl3). | |
The tertiary amino functionalized polysiloxanes were then allowed to react with sodium chloroacetate (SC) to give a generation of carboxybetaine functionalized polysiloxanes. The substances (PDMS-g-CB) were isolated as faint yellow solids, which are fairly hygroscopic and soluble in polar solvents such methanol, ethanol, isopropanol and water. Fig. 3 shows the FT-IR spectrum of PDMS-g-CB, where there is a new absorption band at 1633 cm−1 compared with the spectrum of PDMS-g-DMAA, which is attributed to the C
O stretching vibration of a carboxylate group. Another change in the FT-IR spectra is the disappearance of –N(CH3)2 absorption bands at about 2800 cm−1, which revealed that the tertiary amino groups have been converted into quaternary ammonium groups completely. Fig. 6 shows the 1H-NMR spectrum of PDMS-g-CB, which indicated that the structure of the resulting compound agrees well with expectation. After the quaternization reaction, the characteristic proton signals of the –N(CH3)2 group at about 2.2 ppm shift to 3.2 ppm completely, which also demonstrated that the tertiary amino groups have been converted to quaternary ammonium groups.
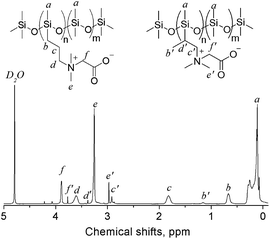 |
| Fig. 6 1H-NMR spectrum of PDMS-g-CB (in D2O). | |
3.2 Surface characterization of b-PDMS films
b-PMDS films were prepared by blending PDMS-g-CB with liquid silicones, which would be cross-linked in the presence of Pt catalyst. Surface structure analysis of b-PDMS films was performed with ATR-FTIR. Fig. 7 shows the typical ATR-FTIR spectra of native PDMS and b-PDMS (0.5 wt%, 1 wt% and 2 wt% PDMS-g-CB1/3, respectively), in which the characteristic signal of the carboxylate group at about 1633 cm−1 was not observed in the native PDMS spectrum but appeared in all spectra of b-PDMS, which indicated that PDMS-g-CB had been blended into the polydimethylsiloxane elastomers successfully. Besides, with the increase of the content of PDMS-g-CB1/3, the peak intensity of C
O bonds also increased, which revealed that the amount of PDMS-g-CB on the surface of PDMS is dependent on the concentration of blended PDMS-g-CB.
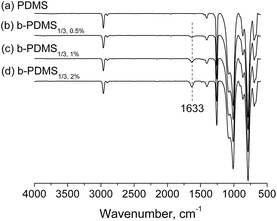 |
| Fig. 7 ATR-FTIR spectra of PDMS-g-CB1/3 blended PDMS: (a) 0%, (b) 0.5%, (c) 1%, (d) 2%. | |
The water contact angles on the surfaces of native PDMS and b-PDMS were measured to characterize the hydrophilic properties of b-PDMS. The detailed contact angle measurement results are shown in Fig. 8. After blending PDMS with PDMS-g-CB, the contact angles of these modified surfaces were decreased significantly, and as the PDMS-g-CB content increased, there was a further slight decrease in the contact angle. The decrease of water contact angles are attributed to the introduction of the hydrophilic carboxybetaine groups on the surfaces of PDMS.
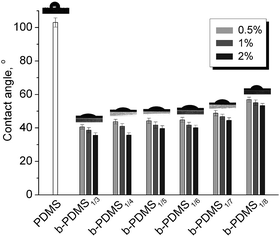 |
| Fig. 8 Static water contact angle of PDMS-g-CB blended PDMS. (The images inserted on the bars are the water contact angle photos for PDMS and b-PDMS1%, respectively.) | |
3.3 Determination of CMC
As a kind of zwitterionic polymer, PDMS-g-CB contains a hydrophobic backbone and several zwitterionic short side chains, and thus it could be considered as a zwitterionic surfactant, and its critical micelle concentration (CMC) was of concern. The CMC values of various grafting ratios were investigated by fluorescence probes and electrical conductivity methods, respectively.
The fluorescence probe technique has been used in the study of surfactant micelles for many years, the so-called pyrene 1
:
3 ratio method is useful for determination of CMC values and aggregation numbers of micelles.32,33 The typical excitation spectra of PDMS-g-CB solutions at different concentrations are presented in Fig. 9. It is noticed that the intensity increases with the concentration of PDMS-g-CB, and the intensity ratio (I1/I3) of first (λ = 373 nm) and third vibrational bands (λ = 384 nm) also changes with the polymer concentration. These changes were attributed to the environment change of pyrene molecules, which were transferred from a water environment to the hydrophobic micelle while the micelles were formed. Thus, we could determinate the CMC values from the relationship between I1/I3 and polymer concentration. Fig. S1† shows the pyrene fluorescent intensity ratio (I1/I3) as a function of varying concentrations of PDMS-g-CB with different carboxybetaine grafting ratios (1/3–1/8). The CMC values of PDMS-g-CB were estimated by the intersection of the two tangents of I1/I3 ratio–log
C curve as shown in Fig. S1† and the results were presented in Fig. 10.
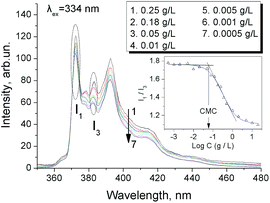 |
| Fig. 9 Fluorescence spectra of pyrene (6 × 10−7 M) in aqueous solutions of PDMS-g-CB1/7 at different concentrations. | |
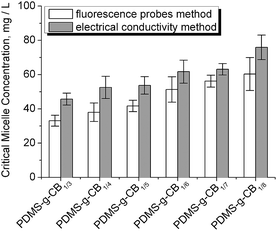 |
| Fig. 10 The CMC values of varied proportions of PDMS-g-CB. | |
We also measured the CMC values of PDMS-g-CB by the common conductance method.34 Fig. S2† shows the conductivity plots for PDMS-g-CB with different carboxybetaine grafting ratios, and Fig. 10 summarizes the results of CMC values calculated from the curves in Fig. S2.†
As can be seen from Fig. 10, The CMC values of PDMS-g-CB vary from 30 mg L−1 to 80 mg L−1, which are even lower than the commonly used sodium dodecyl sulfate surfactant (SDS, CMC ∼ 80 mg L−1 at 25 °C).35 The low CMC of PDMS-g-CB was attributed to the high hydrophobicity of the siloxane backbone. The CMC values increased with the grafting ratio of carboxybetaine groups, and the change may be affected by the hydrophilicity of the grafting zwitterionic carboxybetaine.
3.4 Hemolytic properties of PDMS-g-CB blended PDMS
When biomaterials are exposed to blood, coagulation may be caused by the detrimental interaction between the surfaces of materials and blood constituents. To evaluate the hemolytic properties of b-PDMS and PDMS-g-CB, a hemolysis test was carried out in vitro using fresh rabbit blood under static conditions. Moreover, the hemolysis of red blood cells (RBC) suspension in distilled water and physiological saline was used as positive control and negative control, respectively. Fig. 11 shows the hemolytic activities of b-PDMS with different PDMS-g-CB1/3 content, where the hemolysis ratio of PDMS and b-PDMS were much lower than 5%, which indicated that the blending additive (PDMS-g-CB) also had no hemolytic effect on the RBC suspension.
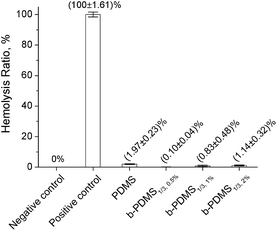 |
| Fig. 11 The results of the hemolysis test for native PDMS and b-PDMS1/3 film. | |
3.5 Antibacterial properties of PDMS-g-CB
To evaluate the antibacterial properties of PDMS-g-CB, different concentrations of PDMS-g-CB solutions were added to the E.coli cultures using the Agar well (Oxford cup) diffusion method, and the antibacterial properties were assessed by measuring inhibition zones. The diameter of inhibition zones of PDMS-g-CB with different concentrations were recorded in Fig. 12 and S6.† Results show that the inhibition zones of PDMS-g-CB were about 7 mm and almost the same with negative control. It indicated that PDMS-g-CB has no antibacterial activity towards E. coli, which conforms to the result of Jiang and co-workers.36
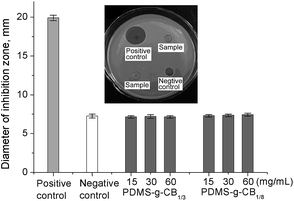 |
| Fig. 12 Antibacterial activity of different concentrations of PDMS-g-CB against E. coli. (The insert image is the antibacterial results for PDMS-g-CB1/3, at 30 mg mL−1). | |
3.6 Evaluation of skin irritation of PDMS-g-CB
As PDMS-g-CB may come in contact with the skin of human or animals in application, we evaluated the skin irritant and sensitization reactions of PDMS-g-CB. Testing for skin irritation of PDMS-g-CB has been performed on rabbits, and the results are shown in Table 1 and Fig. S4.† The results suggested that no skin irritation was caused by PDMS-g-CB. In addition, no significant change was observed in the body weight of test rabbits, and no signs of intoxication were found. As a result, PDMS-g-CB is a novel material with no skin irritation.
Table 1 The evaluation of skin irritation of PDMS-g-CB
Samples |
Mean irritation score |
Irritation indexa |
Intensity |
1 h |
24 h |
48 h |
72 h |
Irritation index = (Σ erythema grades of 24/48/72 h + Σ oedema grades of 24/48/72 h)/(3 × number of rabbits). |
PDMS-g-CB1/3 |
0 |
0 |
0 |
0 |
0 |
No irritation |
PDMS-g-CB1/8 |
0 |
0 |
0 |
0 |
0 |
No irritation |
Gauze |
0 |
0 |
0 |
0 |
0 |
No irritation |
Physiological saline |
0 |
0 |
0 |
0 |
0 |
No irritation |
3.7 Acute oral toxicity study
The toxicities of PDMS-g-CB were investigated by acute oral toxicity test at a dose of 5000 mg kg−1 according to OECD guidelines 425 (OECD TG 425). After feeding for 7 days and 14 days, no abnormal behaviours and adverse reactions were observed in the experimental group mice compared with the control group, and the weight of the experimental group mice and control group mice had no significant difference. During the observation period, no death was noted both in control and experimental groups (Table 2). In addition, the histopathological effect of PDMS-g-CB on organs (heart, liver, spleen, lung and kidney) was observed by light microscopy (Fig. S5†). No histopathological changes were observed both in the experimental groups and control group, and thus PDMS-g-CB has no significant toxicity on the organs (good histocompatibility). Therefore, the oral LD50 of PDMS-g-CB was determined to be greater than 5000 mg kg−1, as the results revealed that PDMS-g-CB could practically be classified as non-toxic.
Table 2 The survival state of mice after acute toxicity test of PDMS-g-CB1/3
|
Dosage (mg kg−1) |
Number of death (n = 8) |
Percentage survival (%) |
12 h |
1 d |
2 d |
3 d |
7 d |
14 d |
Control group |
0 |
0 |
0 |
0 |
0 |
0 |
0 |
100 |
Experimental group |
5000 |
0 |
0 |
0 |
0 |
0 |
0 |
100 |
3.8 Protein adsorption of b-PDMS films
It is believed that most undesired bio-adsorption and bio-fouling on the surfaces of materials are promoted by protein adsorption. Therefore, protein resistance is the primary target for construction of low-fouling or non-fouling surface. Herein, we chose BSA, extensively existing in blood and plasma, as the model protein for protein adsorption testing. Fig. 13 shows the amount of BSA adsorbed onto the surfaces of native PDMS and PDMS-g-CB blended PDMS (b-PDMS), which were calculated according to the micro-BCA method. It was noticed that the amount of adsorbed BSA decreased drastically after modification with PDMS-g-CB, and the amount of adsorbed BSA on b-PDMS surfaces decreased slightly while the carboxybetaine grafting ratio of PDMS-g-CB increased. The results agree well with the water contact angle data as shown in Fig. 8. When PDMS was modified with zwitterionic polymer, the zwitterionic polymer could form a hydration layer on the surface,23 which would prevent the adsorption of protein. Therefore, the more carboxybetaine groups on the modified PDMS surface, the more efficient the resistance to protein adsorption. This explained why less BSA was adsorbed on the b-PDMS1/3 surface compared with b-PDMS1/8 surface.
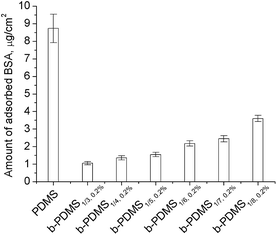 |
| Fig. 13 Amount of protein adsorbed onto the surface of PDMS-g-CB blended PDMS. | |
3.9 Bacterial adhesion of b-PDMS films
The adhesion of bacteria onto materials is an essential factor leading to biofilm formation and infection. To construct a low-fouling surface, it is necessary to reduce or inhibit the adhesion of bacteria. In our work, short-term (24 h) adhesion of E. coli onto b-PDMS surfaces with varying zwitterionic polymer content was investigated, using native PDMS as a control. The adhering bacteria on the materials' surfaces were visualized by SEM. Fig. 14 shows the representative image of native PDMS and b-PDMS after bacterial adhesion. In Fig. 14-A1 and A2, numerous bacteria can be observed on the surface of native PDMS (untreated PDMS). The number of bacteria on the b-PDMS surface (Fig. 14-B–G) was significantly less than the native PDMS surface. After counting the exact number from 10 SEM images for each sample, the average number of adsorbed bacteria was shown in Fig. 15. It was found that the higher the content of PDMS-g-CB is, the less adhering bacteria there are; moreover, the number of adhering bacteria decreased slightly when the carboxybetaine grafting ratio of PDMS-g-CB increased. It was noticed that there is a close relationship between the number of adhering bacteria and the hydrophilicity of the surface. The more hydrophilic the surface is, the less bacteria adhere to it, which is consistent with what Jansen37 and Kodjikian had reported.38 The results of the bacterial adhesion test reveal that PDMS-g-CB modified surfaces could resist the adhesion of E. coli effectively, which is highly desirable for the construction of anti-fouling surfaces.
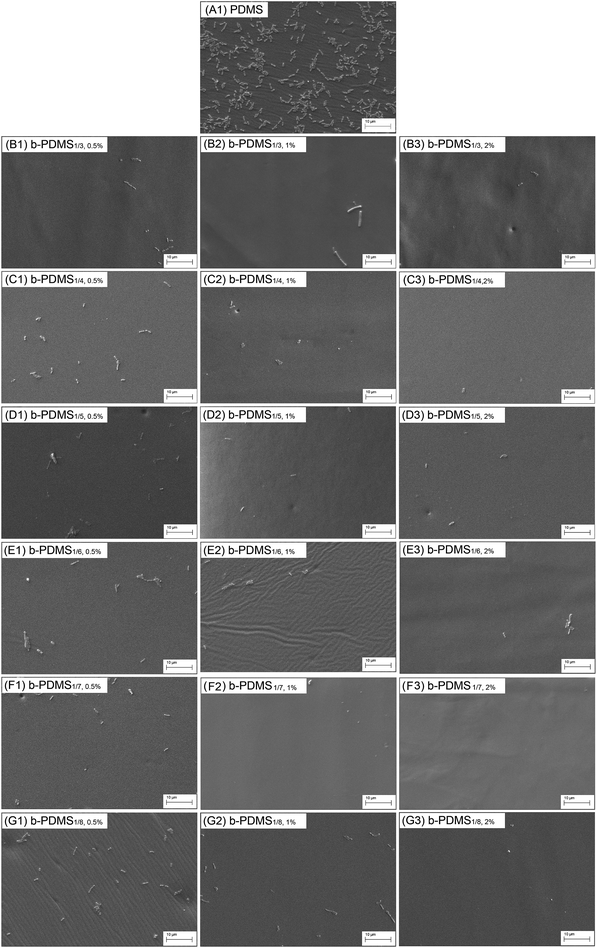 |
| Fig. 14 SEM images of the surfaces of native PDMS and b-PDMS with different PDMS-g-CB content after the short-term bacterial (E. coli) adhesion. | |
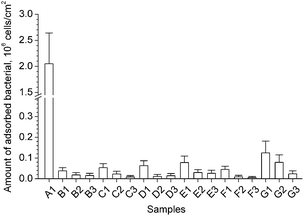 |
| Fig. 15 The average bacterial adsorption on the sample surface calculated from the SEM images. (Samples A1 to G3 have the same meaning with the captions listed in Fig. 14). | |
4. Conclusion
In this study, a series of novel carboxybetaine functionalized polysiloxanes (PDMS-g-CB), composed of a siloxane backbone hydrophobic portion and a carboxybetaine hydrophilic portion, were synthesized and characterized. PDMS-g-CB exhibited a low CMC, water solubility, no antibacterial activity, no skin irritation, non-toxicity and good biocompatibility. PDMS-g-CB modified PDMS films (b-PDMS) showed high resistance to protein adsorption and bacterial adhesion, regardless of the grafting ratio of carboxybetaine groups in PDMS-g-CB. Therefore, PDMS-g-CB is an environmentally friendly material with excellent anti-fouling properties.
Acknowledgements
The authors acknowledge the financial support from the National Natural Science Foundation of China (grants 31201552 and 51003032) and the Specialized Research Fund for the Doctoral Program of Higher Education (grant 20124404120025).
Notes and references
- M. E. Gray, J. Agric. Food Chem., 2010, 59, 5852 CrossRef PubMed.
- M. Perugini, M. Cavaliere, A. Giammarino, P. Mazzone, V. Olivieri and M. Amorena, Chemosphere, 2004, 57, 391 Search PubMed.
- P. Christou, T. Capell, A. Kohli, J. A. Gatehouse and A. M. Gatehouse, Trends Plant Sci., 2006, 11, 302 Search PubMed.
- O. Lopez, J. G. Fernandez-Bolanos and M. V. Gil, Green Chem., 2005, 7, 431 Search PubMed.
- D. R. Walters, Eur. J. Plant Pathol., 2006, 114, 255 Search PubMed.
- F. Jin, B. Z. Ji, S. W. Liu, L. Tian and J. Gao, Acta Entomol. Sin., 2009, 52, 1008 CAS.
- H. G. Silverman and F. F. Roberto, Mar. Biotechnol., 2007, 9, 661 CrossRef CAS PubMed.
- M. F. A. Goosen, S. S. Sablani, H. Ai-Hinai, S. Ai-Obeidani, R. Al-Belushi and D. Jackson, Sep. Sci. Technol., 2005, 39, 2261 CrossRef PubMed.
- P. Kingshott, H. Thissen and H. J. Griesser, Biomaterials, 2002, 23, 2043 CrossRef CAS.
- P. Harder, M. Grunze, R. Dahint, G. M. Whitesides and P. E. Laibinis, J. Phys. Chem. B, 1998, 102, 426 Search PubMed.
- B. Thierry, L. Zimmer, S. McNiven, K. Finnie, C. Barbe and H. J. Griesser, Langmuir, 2008, 24, 8143 CrossRef CAS PubMed.
- P. Gong and D. W. Grainger, Methods Mol. Biol., 2007, 381, 59 CAS.
- L. Li, S. Chen, J. Zheng, B. D. Ratner and S. Jiang, J. Phys. Chem. B, 2005, 109, 2934 CrossRef CAS PubMed.
- J. Zheng, L. Li, H. K. Tsao, Y. J. Sheng, S. Chen and S. Jiang, Biophys. J., 2005, 89, 158 CrossRef CAS PubMed.
- D. A. Herold, K. Keil and D. E. Bruns, Biochem. Pharmacol., 1989, 38, 73 CrossRef CAS.
- E. Ostuni, R. G. Chapman, R. E. Holmlin, S. Takayama and G. M. Whitesides, Langmuir, 2001, 17, 5605 Search PubMed.
- Z. Cao and S. Jiang, Adv. Mater., 2010, 22, 920 CrossRef PubMed.
- J. Ladd, Z. Zhang, S. Chen, J. C. Hower and S. Jiang, Biomacromolecules, 2008, 9, 1357 CrossRef CAS PubMed.
- R. E. Holmlin, X. Chen, R. G. Chapman, S. Takayama and G. M. Whitesides, Langmuir, 2001, 17, 2841 CrossRef CAS.
- G. Cheng, G. Li, H. Xue, S. Chen, J. D. Bryers and S. Jiang, Biomaterials, 2009, 30, 5234 CrossRef CAS PubMed.
- L. R. Carr, H. Xue and S. Jiang, Biomaterials, 2011, 32, 961 Search PubMed.
- W. Yang, H. Xue, L. R. Carr, J. Wang and S. Jiang, Biosens. Bioelectron., 2011, 26, 2454 CrossRef CAS PubMed.
- S. Chen, L. Li, C. Zhao and J. Zheng, Polymer, 2010, 51, 5283 Search PubMed.
- Y. He, J. Hower, S. Chen, M. T. Bernards, Y. Chang and S. Jiang, Langmuir, 2008, 24, 10358 CrossRef CAS PubMed.
- L. Cheng, Q. Liu, L. Yang, Y. Lin and A. Zhang, PMSE Prepr., 2014, 30, 25 Search PubMed , in Chinese.
- K. Ishihara, A. Fujiike, Y. Iwasaki, K. Kurita and N. Nakabayashi, J. Polym. Sci., Part A: Polym. Chem., 1996, 34, 199 Search PubMed.
- S. Fujishita, C. Inaba, S. Tada, H. Kitano, M. Gemmei-Ide and Y. Saruwatari, Biol. Pharm. Bull., 2008, 31, 2309 CAS.
- A. H. Hogt, J. Dankert and J. Feijen, J. Biomed. Mater. Res., 1986, 20, 533 CrossRef CAS PubMed.
- A. Saxena, M. Markanday, A. Sarkar, V. K. Yadav and A. S. Brar, Macromolecules, 2011, 44, 6480 CrossRef CAS.
- X. Hao, J. L. Jeffery, J. S. Wilkie, G. F. Meijs, A. B. Clayton, J. D. Watling, A. Ho, V. Fernandez, C. Acosta, H. Yamamoto, M. G. M. Aly, J. M. Parel and T. C. Hughes, Biomaterials, 2010, 31, 8153 Search PubMed.
- E. Lukevics, L. E. Demicheva, N. P. Yerchak and Y. Popelis, Russ. Chem. Bull., 1993, 42, 1709 Search PubMed.
- J. Aguiar, P. Carpena, J. A. Molina-Bolívar and R. C. Carnero, J. Colloid Interface Sci., 2003, 258, 116 Search PubMed.
- I. Astafieva, X. Zhong and A. Eisenberg, Macromolecules, 1993, 26, 7339 CrossRef CAS.
- A. Dominguez, A. Fernandez, N. Gonzalez, E. Iglesias and L. Montenegro, J. Chem. Educ., 1997, 74, 1227 Search PubMed.
- A. Shiloach and D. Blankschtein, Langmuir, 1998, 14, 7166 CrossRef CAS.
- Z. Zhang, G. Cheng, L. R. Carr, H. Vaisocherova, S. Chen and S. Jiang, Biomaterials, 2008, 29, 4719 CrossRef CAS PubMed.
- B. Jansen and G. Peters, J. Hosp. Infect., 1991, 19, 83 CrossRef CAS.
- L. Kodjikian, C. Burillon, C. Roques, G. Pellon, J. Freney and F. N. R. Renaud, Invest. Ophthalmol. Visual Sci., 2003, 44, 4388 Search PubMed.
Footnote |
† Electronic supplementary information (ESI) available: Synthesis detail of PDMS-g-CB, determination of CMC, skin irritation, acute oral toxicity testing, hemolysis assay and antibacterial activities of PDMS-g-CB. See DOI: 10.1039/c4ra09171j |
|
This journal is © The Royal Society of Chemistry 2014 |
Click here to see how this site uses Cookies. View our privacy policy here.