DOI:
10.1039/C4CE01852D
(Paper)
CrystEngComm, 2015,
17, 174-182
Well-controlled synthesis of wurtzite-type Cu2ZnSnS4 nanoparticles using multiple sulfur sources via a two-step heating process†
Received
10th September 2014
, Accepted 5th November 2014
First published on 5th November 2014
Abstract
Wurtzite-type copper zinc tin sulfide (Cu2ZnSnS4) nanoparticles were prepared in dodecanethiol (DDT) solutions by reacting corresponding metal acetates with a mixture of sulfur compounds with different reactivities, elemental sulfur (S) and dibutylthiourea (DBTU), via a two-step heat treatment. Initial heating at 200 °C enabled the nucleation of metal sulfide nanoparticles composed of two crystal phases, and subsequent heat treatment at 240 °C resulted in the formation of Cu2ZnSnS4 nanoparticles with a wurtzite-type crystal structure. The resulting particles had rod shapes with a stoichiometric composition, the size and shape of which could be controlled by changing both the reaction time and the molar ratio of S and DBTU used in a sulfur precursor. In contrast, spherical Cu2ZnSnS4 nanoparticles with a kesterite-type crystal structure were produced by reaction with pure S as a precursor. The electronic energy levels of the conduction band and valence band edges were determined for wurtzite-type Cu2ZnSnS4 nanoparticles by photoelectrochemical measurement, the individual levels being comparable to those of kesterite-type ones. The light–electricity conversion efficiency varied remarkably depending on the kind of crystal structure: wurtzite-type Cu2ZnSnS4 particles exhibited an efficiency superior to that of the particles with a kesterite-type crystal structure.
1. Introduction
A semiconductor of copper zinc tin sulfide (Cu2ZnSnS4) has been regarded as an attractive candidate for a light-absorbing material in next-generation photovoltaic devices1–5 because all of the elements constituting the semiconductor are earth-abundant elements with little toxicity unlike conventional metal chalcogenide semiconductors such as cadmium chalcogenide,6 lead chalcogenide,7 and copper indium gallium selenide (CIGS),8,9 which have been used in efficient compound semiconductor solar cells showing an efficiency of ca. 20%. Due to the attractive optical properties of Cu2ZnSnS4, such as a suitable band gap (~1.5 eV) for solar cell application and a high absorption coefficient in the wavelength region from visible to near-IR light, intensive research studies have been carried out to fabricate thin-film solar cells with a Cu2ZnSnS4 layer as a light-absorbing material, and the solar energy conversion efficiency has recently reached 12.6%,3 approaching the efficiency of conventional amorphous silicon or compound semiconductor-based solar cells.
In order to prepare a Cu2ZnSnS4 thin film at a low cost, much attention has been paid to Cu2ZnSnS4 nanoparticles synthesized via solution-based approaches. Aqueous or organic suspensions of Cu2ZnSnS4 nanoparticles can be used as nanoparticle ink, and then sintering Cu2ZnSnS4 nanoparticle films deposited on a substrate under appropriate conditions can produce a high-quality bulk Cu2ZnSnS4 film. Such a technique pioneered by Agrawal et al. in 2009 (ref. 10) has been reported to yield a thin Cu2ZnSnS4 film easily and inexpensively in comparison with other techniques such as spray pyrolysis, electron beam evaporation, sputtering, and chemical deposition. It has also been reported that thin-film solar cells composed of Cu2ZnSn(S,Se)4, fabricated by sintering Cu2ZnSnS4 nanoparticles in selenium vapor,11 exhibited relatively high conversion efficiency of 7.2%. Furthermore, Cu2ZnSnS4 nanoparticles with diameters of less than ca. 5 nm have been reported by Aydil et al. and Liu et al.12,13 to exhibit size-dependent absorption properties of Cu2ZnSnS4 nanoparticles due to the quantum size effect, and they have been of great interest as a light-absorbing material in highly efficient quantum dot solar cells.14 We have recently clarified that size-quantized Cu2ZnSnS4 nanoparticles had a tunable electronic energy structure depending on their size15 and that their light–electricity conversion efficiency was greatly varied by the chemical composition of the particles.16
The thermodynamically stable crystal structure of Cu2ZnSnS4 is usually kesterite, which is derived from a zinc-blende lattice with a tetragonal crystal cell. However, Cu2ZnSnS4 nanoparticles with a metastable wurtzite-derived structure have been reported in recent years.17–24 The wurtzite-type Cu2ZnSnS4 could be exclusively seen in the form of nanoparticles that had unique shapes derived from the wurtzite-type structure of a hexagonal crystal lattice. Most of the wurtzite-type Cu2ZnSnS4 nanoparticles were synthesized by the hot-injection method,17,18,23,24 while some researchers used one-pot heating synthesis.19–22 Peng et al. first synthesized wurtzite-type Cu2ZnSnS4 by hot injection of metal chlorides dissolved in 1-dodecanethiol (DDT) into a mixed solvent of DDT, oleylamine (OLA) and/or oleic acid (OAc) at 240 °C.17 Ryan et al. reported high-quality and monodisperse wurtzite-type Cu2ZnSnS4 nanorods prepared by injection of DDT and tert-dodecylmercaptan (t-DDT) into 1-octadecene (ODE) containing copper(II) acetylacetonate, zinc(II) acetate, tin(IV) acetate, and trioctylphosphine oxide (TOPO).18 Since wurtzite-type Cu2ZnSnS4 nanoparticles were reported to have a higher carrier concentration and lower resistivity than those of kesterite-type ones,19 they have attracted attention as a novel light-absorbing material for photovoltaic applications. Although diverse techniques for the syntheses of wurtzite-type nanoparticles, in which various reagents were used without noting their functions, have been reported, the reaction conditions have not been optimized despite the considerable interest shown in characteristics of wurtzite-type Cu2ZnSnS4 nanoparticles. Furthermore, there have been only a few reports on semiconductor photoelectrodes fabricated with wurtzite-type Cu2ZnSnS4 nanoparticles.
Several researchers have recently demonstrated that wurtzite-type Cu2ZnSnS4 grew from a djurleite (Cu1.94S) nanoparticle as the nucleus.19–21,23 Vela et al. and Jiang et al. showed that controlling reactivity of metal precursors with a sulfur source to generate a djurleite nucleus is of great importance for the subsequent crystal growth of wurtzite-type Cu2ZnSnS4 nanoparticles.23,24 However, a method for synthesis in which the nucleation process can be clearly separated from the crystal growth processes has not been established. Thus, selective preparation of wurtzite-type Cu2ZnSnS4 nanoparticles is still a challenging issue. Herein, we propose a new approach to synthesize wurtzite-type Cu2ZnSnS4 nanoparticles using two different sulfur sources, elemental sulfur (S) and 1,3-dibutylthiourea (DBTU),25via a two-step heating process, in which the difference in reactivity of sulfur precursors with metal ions enables stable nucleus formation of a djurleite structure followed by crystal growth of wurtzite-type Cu2ZnSnS4 nanoparticles. The photoelectrochemical properties of wurtzite Cu2ZnSnS4 particles were investigated by immobilizing the thus-obtained particles on indium tin oxide (ITO) electrodes.
2. Experimental
2.1 Materials
Copper(II) acetate (Cu(CH3COO)2), zinc(II) acetate (Zn(CH3COO)2), 1-dodecanethiol (DDT), and 1,3-dibutylthiourea (DBTU) were commercially available from Wako Pure Chemical Industries. Tin(IV) acetate (Sn(CH3COO)4) was obtained from Aldrich. Europium(III) nitrate (Eu(NO3)3) hexahydrate and sulfur (S) were purchased from Kishida Chemical. All reagents were used as received. Aqueous solutions were prepared with water purified by using a Millipore Milli-Q system.
2.2 Syntheses of wurtzite- and kesterite-type Cu2ZnSnS4 nanoparticles
A two-step heating process was adopted for the synthesis of wurtzite-type Cu2ZnSnS4 nanoparticles using a mixture of S and DBTU as a sulfur precursor, a typical procedure of which is described below. Powders of Cu(CH3COO)2 (0.10 mmol), Zn(CH3COO)2 (0.050 mmol), and Sn(CH3COO)4 (0.050 mmol) were dispersed in DDT (3.0 cm3). The suspension was heated at 200 °C for ca. 5 min with vigorous stirring under an N2 atmosphere to yield a homogeneous transparent solution. To the solution, 0.30 cm3 DDT solution containing S (0.10 mmol) and DBTU (0.10 mmol) was quickly added, followed by stirring for 30 min at 200 °C. Then, the reaction temperature was quickly raised to 240 °C, and the solution was further stirred for 0–180 min. After cooling down to room temperature, the reaction mixture was subjected to centrifugation at 4000 rpm for 5 min. The resulting precipitates were washed with ethanol several times and dried under vacuum. The precipitates were again dissolved in hexane (2.0 cm3), followed by the removal of large precipitates by filtration through a syringe filter (pore size: 1.2 μm) before use. Kesterite-type Cu2ZnSnS4 nanoparticles could be prepared by a similar method except for the addition of pure elemental S (0.20 mol) as a sulfur precursor. Reaction conditions, including the molar ratio of S and DBTU used in the sulfur precursor, reaction temperature of each heating process, and reaction time, were varied to investigate the influence of these parameters on the morphology of Cu2ZnSnS4 nanoparticles and their crystal structure.
2.3 Characterization of nanoparticles
The chemical composition of the particles was determined by X-ray fluorescence (XRF) spectroscopy (Rigaku, EDXL-300) or energy dispersive X-ray (EDX) analysis using a Hitachi SU-1500 scanning electron microscope equipped with an EDX analyzer (Horiba, EMAX ENERGY EX-250). The shape and size distribution of the obtained particles were observed using a Hitachi H7650 transmission electron microscope (TEM) with an acceleration voltage of 100 kV. Samples for TEM measurements were prepared by dropwise addition of a hexane solution containing the particles onto a carbon-coated copper grid, followed by drying under reduced pressure. Average length and width of the particles were determined by measuring the dimensions of at least 100 particles in the TEM images. A Rigaku Smart Lab powder X-ray diffraction (XRD) instrument equipped with an X-ray tube (Cu Kα radiation: λ = 1.54059 Å, 40 kV, 30 mA) was used for analyzing the crystal structure of the particles, with measurements being carried out for samples placed on a low-background silicon sample holder. Absorption spectra of particles in hexane were obtained by using an Agilent Technology 8453 UV-visible spectrophotometer.
2.4 Photoelectrochemical measurements
Cu2ZnSnS4 nanoparticles were immobilized on ITO-coated glass electrodes for photoelectrochemical measurements. A hexane solution of the wurtzite-type Cu2ZnSnS4 nanoparticles was spin-coated onto an ITO electrode at 2000 rpm for 20 s. To immobilize the particles on the ITO, the electrode was heated at 250 °C for 5 min under reduced pressure. The absorbance of thus-obtained particle films was ca. 0.08 at 400 nm. The photoelectrochemical properties of the nanoparticle-immobilized ITO electrodes were measured in an aqueous solution containing 0.20 mol dm−3 Eu(NO3)3 (pH = 2.3) as an electron acceptor. The potential was determined against an Ag/AgCl (sat. KCl) reference electrode. A Pt wire was used as a counter electrode. The photocurrent was detected using a Hokuto Denko HAB-151A potentiostat. Light irradiation (λ > 350 nm) to the electrode was carried out by passing light from a 300 W Xe lamp (USHIO X300) through a UV cut-off filter and a light chopper. The light intensity of the irradiation was ca. 0.9 W cm−2 at the electrode surface. Action spectra of the photocurrent were measured by irradiation of monochromatic light, which was obtained by passing light through a JASCO CT-10 monochromator. The incident photon-to-current efficiency (IPCE) was calculated by dividing the number of electrons detected in the photocurrent by that of incident photons.
3. Results and discussion
3.1 Synthesis of wurtzite-type Cu2ZnSnS4 nanoparticles via a two-step heating process
It is well known that thiol compounds potentially act as a sulfur source for the preparation of metal sulfide particles: metal thiolate complexes, formed by the reaction of metal ions with thiols, can be decomposed via C–S bond cleavage at a high temperature,26 allowing the formation of metal sulfide nanoparticles even without addition of sulfur sources such as elemental S. Therefore, we first investigated the reactivity of metal acetates with DDT. Powders of metal acetates were pre-heated in DDT under an N2 atmosphere to obtain a homogeneous solution containing metal thiolates. Mixture powders of Cu(CH3COO)2 (0.10 mmol), Zn(CH3COO)2 (0.050 mmol), and Sn(CH3COO)4 (0.050 mmol) were not completely dissolved in DDT (3.0 cm3) at a temperature below ca. 120 °C, but the solution became transparent by heat treatment at 150–200 °C for less than 5 min. On the other hand, heating the solution at a temperature higher than 240 °C produced dark brown precipitates within several minutes. The thus-obtained precipitates could not be dissolved in organic solvents such as hexane, toluene, and chloroform. TEM measurement (Fig. S1†) revealed that the precipitates obtained by heat treatment at 240 °C for 60 min were composed of relatively large octahedron-like particles with diagonal lengths of 20–40 nm as a result of thermal decomposition of metal thiolates. The particles had the chemical composition of Cu
:
Zn
:
Sn = 0.86
:
0.07
:
0.07, indicating that metal sulfides produced by decomposition of metal thiolates at 240 °C were not Cu2ZnSnS4 but mainly contained Cu as a metal species. Since the thermal decomposition may proceed gradually according to decomposition kinetics, it is thought that a small number of nuclei are generated in the early stage of the reaction and crystal growth occurs simultaneously with the nucleation, resulting in the formation of relatively large nanoparticles with a wide size distribution. In addition, a Cu precursor tends to form metal sulfide more easily than Zn and Sn ones.23 Therefore, we used temperatures ranging from 150 to 200 °C for preparation of a metal acetate precursor solution without formation of undesired metal sulfides.
As the first heating process in Cu2ZnSnS4 preparation, a 0.30 cm3 portion of DDT solution containing two different sulfur sources, S (0.10 mmol) and DBTU (0.10 mmol), was injected into the metal thiolate precursor solution at 200 °C, followed by stirring for 30 min. Fig. 1a shows a representative TEM image of the thus-obtained nanoparticles. Mushroom-like polygonal nanoparticles could be observed in the images, which consisted of at least two parts with different contrast, and the average sizes of the nanoparticles were ca. 7 nm in length and ca. 5 nm in width. The chemical composition of whole particles was determined to be Cu
:
Zn
:
Sn = 0.79
:
0.13
:
0.08. XRD patterns of the particles (Fig. 1b) revealed that the particles contained at least two crystal phases. The main peaks could be assigned to the wurtzite phase of Cu2ZnSnS4, while a small peak at 38° could be assigned to the (842) plane of a djurleite crystal with a monoclinic structure. Since wurtzite-type Cu2ZnSnS4 nanoparticles have been reported to grow from the nuclei of djurleite (Cu1.94S) nanoparticles,19–21,23 it is reasonable to assign the diffraction pattern to the mixture of djurleite (Cu1.94S) and wurtzite Cu2ZnSnS4 crystal phases. Prolongation of the reaction time from 30 to 180 min still resulted in the formation of polygonal particles composed of a mixture of crystal phases but not pure wurtzite Cu2ZnSnS4 (Fig. S2†).
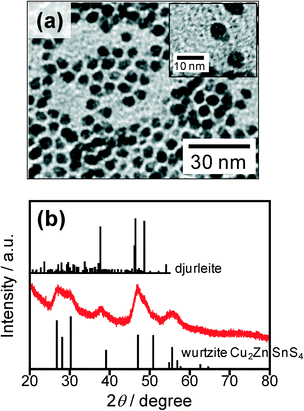 |
| Fig. 1 TEM image (a) and XRD pattern (b) of nanoparticles obtained by injection of S and DBTU (S : DBTU = 50 : 50) into the precursor solution at 200 °C and stirring for 30 min (first heating process). Reference XRD pattern of djurleite (PDF card: #00-034-0660) and simulated pattern of wurtzite-type Cu2ZnSnS4 shown in ref. 17 are also shown. | |
In contrast, an increase in the reaction temperature, represented as the second heating process in the present study, resulted in conversion of the mixed crystal phases of metal sulfide particles into pure wurtzite phase. Just after the reaction of metal acetates with a mixture of S and DBTU in DDT at 200 °C for 30 min, heating the reaction solution at a higher temperature of 240 or 270 °C for 30 min resulted in the formation of nanoparticles with rod-like shapes as shown in Fig. 2a and b, the average lengths and widths being 9.3 and 5.7 nm for 240 °C and 10.7 and 7.0 nm for 270 °C, respectively. The chemical compositions of the particles obtained at 240 °C and 270 °C were Cu
:
Zn
:
Sn = 0.57
:
0.20
:
0.23 and 0.48
:
0.22
:
0.28, respectively, being close to the stoichiometric chemical composition of Cu2ZnSnS4 (Cu
:
Zn
:
Sn = 0.50
:
0.25
:
0.25). These results indicated that Zn and Sn ions were sufficiently incorporated into the particles in the second heating process. Fig. 2c shows XRD patterns of the resulting particles. The diffraction peaks observed for each kind of particle could be definitely assigned to the wurtzite Cu2ZnSnS4 crystal phase, while there was no secondary crystal phase, such as djurleite Cu1.94S or other metal sulfides.
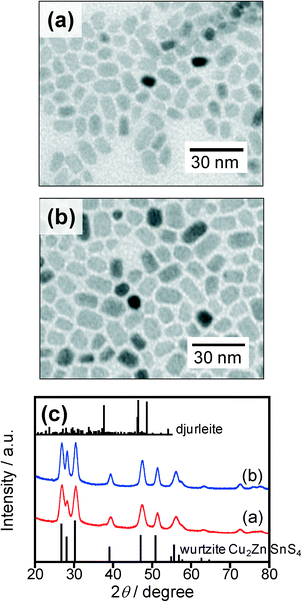 |
| Fig. 2 (a, b) TEM images of nanoparticles obtained by heating at 240 (a) and 270 °C (b) for 30 min in the second heating process. The solution used was prepared by heating at 200 °C for 30 min in the first heating process after the injection of a sulfur precursor (S : DBTU = 50 : 50). (c) Corresponding XRD patterns for the nanoparticles shown in panels a and b. Reference pattern of djurleite (PDF card: #00-034-0660) and simulated pattern of wurtzite-type Cu2ZnSnS4 shown in ref. 17 are also shown. | |
Consequently, wurtzite-type Cu2ZnSnS4 nanoparticles were successfully obtained via a two-step heating process (200 °C for 30 min followed by 240 or 270 °C for 30 min) with the second step requiring a reaction temperature higher than 240 °C for the formation of stoichiometric wurtzite-type Cu2ZnSnS4 nanoparticles. We could assume in the present study that the first heating process at 200 °C for 30 min after the addition of sulfur sources of S and DBTU was the “nucleation process” giving mushroom-like nanoparticles as shown in Fig. 1 and that the following heating process at 240 or 270 °C was the “crystal growth process” of wurtzite Cu2ZnSnS4 nanoparticles. It should be noted that the decrease in the nucleation temperature from 200 to 150 °C hardly changed the morphology of the resulting particles: when the heating in the nucleation process was carried out at 150 °C for 30 min followed by the crystal growth process at 240 °C for 30 min, pure wurtzite Cu2ZnSnS4 nanoparticles were produced as shown in Fig. S3† with average length and width of 9.8 nm and 5.5 nm, respectively, being similar to those of particles prepared through the nucleation at 200 °C (Fig. 2a). These indicated that the size and crystal structure of the resulting Cu2ZnSnS4 particles were not significantly dependent on the nucleation temperature ranging from 150 to 200 °C.
3.2 Influence of the kind of sulfur precursor on the morphology of Cu2ZnSnS4 nanoparticles
It was reported in our previous paper27 that the reaction of corresponding metal acetates with elemental sulfur in pure OLA simply produced spherical Cu2ZnSnS4 nanoparticles of a kesterite-type crystal structure at a reaction temperature higher than 240 °C, being different from the present case of using multiple sulfur precursors. Thus, it is thought that the molar ratio of S and DBTU used in a sulfur precursor can affect the size, shape and crystal structure of the resulting Cu2ZnSnS4 particles. Two-step synthesis of Cu2ZnSnS4 particles was carried out by changing the ratio of S to DBTU in a sulfur precursor, in which the total concentration of S and DBTU was fixed at 0.20 mmol. Fig. 3a–e show representative TEM images of nanoparticles prepared with various ratios of S to DBTU. The obtained Cu2ZnSnS4 nanoparticles had a nearly stoichiometric ratio of Cu
:
Zn
:
Sn regardless of the precursor ratio of S to DBTU. At a glance, the size and shape of the particles dramatically changed depending on the ratio of S to DBTU: small spherical particles with sizes of ca. 4 nm were formed with injection of pure S, while large wedge- or rod-shaped particles of ca. 50 nm in length and ca. 10 nm in width were produced with the use of pure DBTU as a sulfur precursor. However, thin rod-shaped particles (width: ca. 4–6 nm) were formed when a mixture of S and DBTU was used. Their length was dependent on the ratio of S to DBTU: the average rod lengths (standard deviations) were determined to be 14.3 nm (6.1 nm) for S
:
DBTU = 75
:
25, 9.3 nm (1.9 nm) for S
:
DBTU = 50
:
50, and 11.3 nm (4.3 nm) for S
:
DBTU = 25
:
75, with the precursor ratio of S
:
DBTU = 50
:
50 being suitable for obtaining wurtzite-type Cu2ZnSnS4 nanoparticles with a narrower size distribution. XRD patterns of these particles shown in Fig. 3f revealed that synthesis in the presence of DBTU produced wurtzite-type Cu2ZnSnS4 nanoparticles, but a pure kesterite phase of Cu2ZnSnS4 nanoparticles appeared only in the case of injection of pure S as a sulfur precursor.
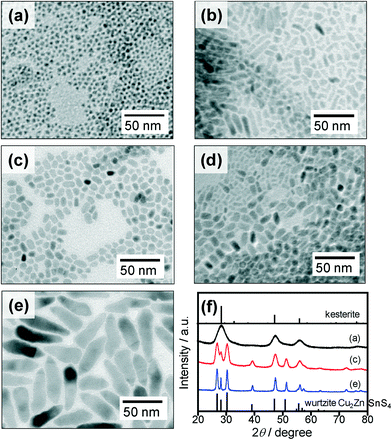 |
| Fig. 3 (a–e) TEM images of nanoparticles obtained by reactions using various ratios of S and DBTU. The ratios of S : DBTU were 100 : 0 (a), 75 : 25 (b), 50 : 50 (c), 25 : 75 (d), and 0 : 100 (e). (f) Corresponding XRD patterns for the nanoparticles shown in panels a, c, and e. Reference pattern of kesterite Cu2ZnSnS4 (PDF card: #01-075-4122) and simulated pattern of wurtzite-type Cu2ZnSnS4 shown in ref. 17 are also shown. | |
So far, phase-controlled synthesis has usually been performed by changing the kind or ratio of coordinating solvents such as DDT, OLA, ODE, and OAc.19,20,22 However, the effect of the coordinating solvent on selective formation of wurtzite-type Cu2ZnSnS4 nanoparticles was not sufficiently discussed in the literature. In the present study, spherical Cu2ZnSnS4 particles with a kesterite structure were formed even in pure DDT by using pure S as a sulfur source, being similar to the synthesis in pure OLA described in our previous paper.27 This suggested that surface modification with DDT was not a key factor to determine the polymorphism of Cu2ZnSnS4 nanocrystals. In contrast, inclusion of DBTU in the sulfur precursor resulted in the production of nanoparticles of wurtzite-type Cu2ZnSnS4 regardless of the fraction of DBTU, indicating that DBTU played an important role in the formation of wurtzite-type Cu2ZnSnS4 nanoparticles. The results showing that pure DBTU only caused the formation of large wedge-like nanoparticles could be reasonably understood by the relatively weak reactivity of DBTU for metal sulfide formation. Active sulfur species would be gradually provided to the solution via thermal decomposition of DBTU, so that a small number of nuclei were generated in the early stage of the reaction along with crystal growth, resulting in the formation of relatively large nanoparticles with a wide size distribution.
3.3 Controlling the crystal growth process
As mentioned in section 3.1, we could assume that the first heating process at 200 °C for 30 min after injection of the sulfur precursor was the “nucleation process” and that the following heating process at 240 °C was the “crystal growth process” of wurtzite Cu2ZnSnS4 nanoparticles. We investigated the reaction time used in the second step to control the degree of crystal growth of wurtzite-type Cu2ZnSnS4 nanocrystals. Synthesis was carried out by injecting the precursor mixture of S and DBTU (50
:
50), with mushroom-like nanoparticles having a heterostructure as shown in Fig. 1a being formed in the first heating process and then used as nuclei for the crystal growth process. With heat treatment at 240 °C for 15 min in the second heating step, the initial mushroom-like particles completely disappeared and then particles with rod or polygon shapes were alternatively formed as shown in Fig. S4b.† Prolongation of heat treatment in the second step to 30–180 min resulted in slight changes in the size and shape of the particles (Fig. S4†). Fig. 4 shows the changes in morphology of particles and their chemical compositions as a function of reaction time in the second heating step, determined from TEM measurement and XRF spectroscopy. In the early stage of crystal growth up to 30 min, the particles anisotropically grew as evidenced by an increase in the aspect ratio from 1.39 to 1.64. By heating for more than 30 min, the width of the particles predominantly increased with an increase in the reaction time, but the length was almost constant, resulting in a monotonously decrease in the aspect ratio to ca. 1.4. This suggested an increase in the fraction of polygonal particles with nearly isotropic shapes along with crystal growth. Cu-rich nanoparticles with the composition of Cu
:
Zn
:
Sn = 0.79
:
0.13
:
0.08 were formed just after the nucleation process. With elapse of heating time in the crystal growth process, Zn and Sn ions were gradually incorporated into the nanoparticles. A nearly stoichiometric composition of Cu2ZnSnS4 particles was obtained by heating for more than 30 min. These results suggested that the incorporation of Sn and Zn ions dissolved in the solution into nanocrystals induced elongation of Cu2ZnSnS4 rod particles in which the aspect ratio increased from 1.39 to 1.64.
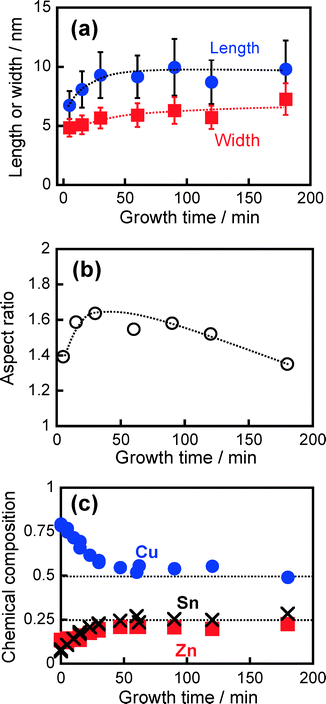 |
| Fig. 4 Change in the morphology (a, b) and chemical composition (c) of nanoparticles with an increase in heating time in the second step. The length (solid circles) and width (solid squares) of the particles and their aspect ratio are shown in panels a and b. Error bars in panel a represent standard deviations of length and width. In panel c, the fractions of metal species are Cu (solid circles), Zn (solid squares), and Sn (crosses). | |
Fig. 5 shows changes in the XRD patterns of the particles obtained with different reaction times. As aforementioned, the nuclei, that is, particles heat-treated in the second heating process for 0 min, contained two crystal phases consisting of a djurleite Cu1.94S crystal exhibiting a strong diffraction peak at ca. 38° and a wurtzite Cu2ZnSnS4 crystal exhibiting peaks at around 27, 28, 30, 39, 47, 51, and 56°. With an increase in reaction time, the peaks assignable to the wurtzite Cu2ZnSnS4 crystal became sharper, but the intensity of a broad peak at 38° assigned to the djurleite Cu1.94S crystal decreased. Particles heat-treated for more than 30 min exhibited diffraction peaks assignable to the wurtzite structure only. This behavior was in good agreement with the change in chemical composition of the particles with the crystal growth process as shown in Fig. 4c: a nearly stoichiometric composition of Cu2ZnSnS4 particles was obtained by heating for more than 30 min, while Cu-rich nanoparticles were formed at the initial stage of the reaction.
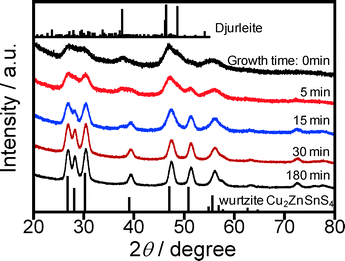 |
| Fig. 5 Change in XRD diffraction pattern of obtained nanoparticles with an increase in reaction time in the second heating step at 240 °C. Reference pattern of djurleite (PDF card: #00-034-0660) and simulated pattern of wurtzite-type Cu2ZnSnS4 shown in ref. 17 are also shown. | |
The absorption spectra of particles dispersed in the solution support the disappearance of djurleite along with formation of stoichiometric Cu2ZnSnS4 having a wurtzite phase. As shown in Fig. 6, a broad absorption band was observed in the near-IR region with a wavelength longer than 900 nm in the case of particles just after the nucleation process, which was assigned to localized surface plasmon resonance (LSPR) absorption of djurleite nanoparticles of Cu1.94S.25,28 This LSPR-peak intensity of Cu1.94S decreased with elapse of reaction time and finally the absorption onset appeared at ca. 850 nm, roughly corresponding to the energy gap of wurtzite-type Cu2ZnSnS4 (~1.5 eV).18–21
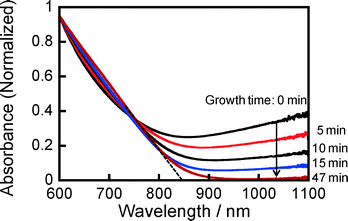 |
| Fig. 6 Change in absorption spectrum of obtained nanoparticles in hexane with an increase in reaction time in the second heating step at 240 °C. | |
3.4 Mechanism of crystal-phase-selective formation of Cu2ZnSnS4 nanoparticles
We successfully developed crystal-phase-selective synthesis of Cu2ZnSnS4 nanoparticles, in which the crystal structure of Cu2ZnSnS4 nanoparticles, wurtzite or kesterite type, could be controlled only by changing the molar ratio of the two different sulfur sources. The results obtained in this study are summarized in Scheme 1. For the formation of wurtzite-type Cu2ZnSnS4 nanoparticles, an equal molar ratio of S and DBTU (S
:
DBTU = 50
:
50) produced mushroom-like nuclei (Fig. 1a). Similar heterostructured nanoparticles have been reported for multinary metal chalcogenide semiconductors. For example, Gao et al. reported tadpole-like nanoparticles composed of djurleite Cu1.94S as the “head” and wurtzite-type ZnS as the “tail”,29 in which the wurtzite-type ZnS epitaxially grew on monoclinic Cu1.94S along the c-crystallographic axis. The anisotropic growth of wurtzite ZnS was derived from the small lattice mismatch on the corresponding interface of djurleite Cu1.94S.29,30 The formation of heterostructured nuclei shown in Fig. 1a can be explained by a similar mechanism.
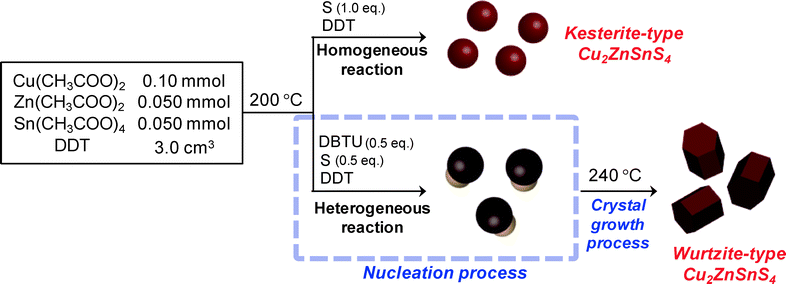 |
| Scheme 1 Schematic diagram of the syntheses of kesterite- and wurtzite-type Cu2ZnSnS4 nanoparticles. | |
We propose the following mechanism for the selective formation of wurtzite-type Cu2ZnSnS4 particles. At first, during the heating at 200 °C as the nucleation process, djurleite Cu1.94S nanoparticles were formed by the reaction of Cu precursors with a more reactive precursor of S (0.5 eq. to the metal precursors) because Cu sulfide is apt to form more easily than those of Zn and Sn.23 Along with the formation of djurleite nuclei, thermal decomposition of DBTU gradually occurred to release additional active sulfur species, resulting in the formation of a wurtzite-type ZnS (or Cu- and Sn-doped ZnS) crystal phase that was anisotropically grown from thus-obtained djurleite Cu1.94S nanoparticles. In the following heating step at a higher temperature of 240 °C as the second step, the ions remaining in the solution, such as Zn and Sn, reacted with an active sulfur species that was formed by the complete decomposition of DBTU or metal thiolates partially decomposed to form metal sulfides, resulting in crystal growth of the nuclei.
Heat treatment at a temperature higher than 240 °C was essential in the present study for the formation of stoichiometric wurtzite-type Cu2ZnSnS4 nanoparticles. It has been reported that the formation of homogeneous nanoparticles was based on crystal growth accompanied by interdiffusion of Cu ions in the nanoparticles at a higher temperature.19–21 In addition, a ripening process via forward and backward reactions in the system should also relate to the formation of the homogeneous nanoparticles. The forward reactions consisted of the formation of metal sulfides by reaction of metal thiolates with injected sulfur precursors and by partial decomposition of metal thiolates, while the backward reaction was dissolution of metal sulfide nanoparticles by DDT to regenerate metal thiolates. When the reaction temperature was raised to 240 °C, the forward and backward reactions should repeatedly occur until the reaction reaches an apparent equilibrium in the system, resulting in the formation of wurtzite-type Cu2ZnSnS4 nanoparticles with a stable shape and chemical composition. So far, it has been reported that pure wurtzite-type Cu2ZnSnS4 nanoparticles were synthesized only at high temperature (>210 °C) in the presence of alkylthiol.17–24 These can support the mechanism described above.
On the other hand, kesterite-type Cu2ZnSnS4 particles could be selectively formed only in the case of the metal precursors having completely reacted with a sulfur source to form metal sulfides in a single step. For example, when a 0.20 mmol portion of S (1.0 eq. to the metal precursors) was injected into the reaction solution, the total amounts of metal precursors homogeneously reacted due to the addition of a sufficient amount of a highly reactive sulfur source, resulting in thermodynamically stable Cu2ZnSnS4 nanoparticles of a kesterite structure without formation of djurleite Cu1.94S nuclei.
3.5 Photoelectrochemical properties of wurtzite-type Cu2ZnSnS4 nanoparticles depending on the crystal structure
Photoelectrochemical measurements were carried out in order to evaluate photoelectrochemical properties of wurtzite-type Cu2ZnSnS4 nanoparticles, in comparison with kesterite-type Cu2ZnSnS4 nanoparticles. Fig. 7a shows a photocurrent–potential curve of a Cu2ZnSnS4 nanoparticle-immobilized electrode measured in an Eu(NO3)3 aqueous solution upon intermittent light irradiation (λ > 350 nm), where wurtzite-type particles of 9.3 nm in length and 5.7 nm in width as shown in Fig. 2a were used. A cathodic photocurrent was observed with light irradiation, indicating that the wurtzite-type Cu2ZnSnS4 nanoparticles behaved as a p-type semiconductor, being similar to kesterite-type ones. In the case of bulk p-type semiconductor electrodes, photogenerated holes diffuse inside the semiconductor and then can be injected into a contacting electrode as long as the electrode potential is more negative than that of the flatband potential (EFB). Therefore, it is reasonably assumed that EFB in most p-type semiconductors is comparable to the potential of the valence band edge (EVB). The onset potential of the cathodic photocurrent in Fig. 7a could be regarded as EVB and was determined to be ca. +0.15 V vs. Ag/AgCl, the value of which agreed with that previously reported for kesterite-type Cu2ZnSnS4 nanoparticles of 5–6 nm in diameter.15,27
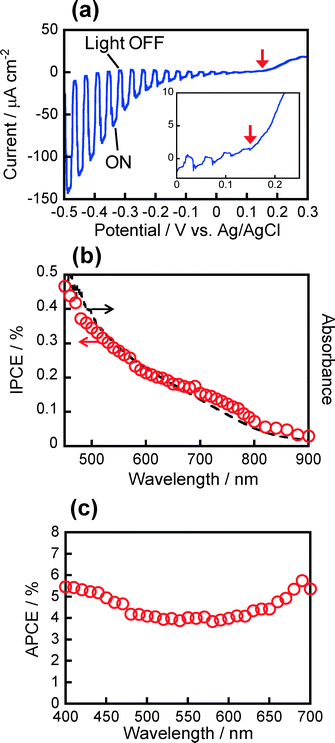 |
| Fig. 7 (a) Photocurrent–potential curves of wurtzite-type Cu2ZnSnS4 nanoparticles immobilized on an ITO electrode. An arrow shows the onset potential of the photocurrent. (b) IPCE and (c) APCE of the wurtzite-type Cu2ZnSnS4 nanoparticulate electrode measured under potential application of −0.5 V vs. Ag/AgCl. The broken line in panel b represents absorption spectrum of the corresponding wurtzite-type Cu2ZnSnS4 nanoparticles in hexane. | |
The action spectrum of the cathodic photocurrent is shown in Fig. 7b. The onset wavelength appeared at ca. 850–900 nm, being in rough agreement with the absorption onset of particles in the solution. Since the prepared film was very thin with absorbance of ca. 0.08 at 400 nm to avoid carrier trapping inside the particulate films, the obtained IPCE was relatively low (less than ca. 0.6%). The absorbed photon-to-current efficiency (APCE), calculated by dividing the number of electrons detected in the photocurrent by that of photons absorbed by Cu2ZnSnS4 film, was ca. 4–5% as shown in Fig. 7c, regardless of the wavelength of irradiating monochromatic light. It should be noted that the present wurtzite-type Cu2ZnSnS4 nanoparticles exhibited a ca. 10 times larger IPCE value than those obtained with kesterite-type ones: as reported in our previous paper,16 the photoelectrochemical activity of ITO electrodes modified with kesterite-type Cu2ZnSnS4 particles, the absorbance of which was ca. 0.045 at 400 nm, was considerably dependent on the chemical composition, and the maximum IPCE value at 400 nm irradiation was 0.045% (corresponding to APCE of 0.48%) with the use of particles having the composition of Cu
:
Zn
:
Sn = 0.47
:
0.23
:
0.29. These results suggest that wurtzite-type Cu2ZnSnS4 nanoparticles showed better performance than kesterite-type ones as a photovoltaic material. This is consistent with the results of a previous study showing that wurtzite-type Cu2ZnSnS4 nanoparticles had a higher carrier concentration and lower resistivity than those of kesterite-type ones.19
Conclusions
We demonstrated well-defined synthesis of wurtzite-type Cu2ZnSnS4 nanoparticles using two different sulfur sources via a two-step heating process. The method could clearly distinguish a nucleation process from a crystal growth process. In the nucleation process, injection of the sulfur precursor mixture, S and DBTU (with a 50
:
50 ratio), into the metal precursor solution enabled the formation of heterostructured particles composed of wurtzite-type Cu2ZnSnS4 and djurleite Cu1.94S nanoparticles. In the following crystal growth process, these particles acted as nuclei to produce wurtzite-type Cu2ZnSnS4 nanoparticles with a stoichiometric composition. In addition, the morphology, chemical composition, and crystal structure of the particles could be controlled by changing the reaction conditions, including the molar ratio of S and DBTU, reaction temperature, and reaction time. This well-controlled method for synthesis of wurtzite-type Cu2ZnSnS4 nanoparticles will have a great impact on studies not only on Cu2ZnSnS4 nanoparticles but also on other multinary metal sulfide semiconductor nanoparticles such as CuInS2 because they can also form a metastable wurtzite-type structure from copper sulfide nuclei.31
Another interesting finding is that the photoelectrochemical properties of Cu2ZnSnS4 nanoparticles varied depending on the kind of crystal structure. Notably, the light–electricity conversion efficiency of wurtzite-type particles was much higher than that of kesterite-type ones, suggesting prominent properties of the wurtzite-type nanoparticles as an efficient light-absorbing material for photovoltaic applications. Improvement in the method for synthesis of wurtzite-type Cu2ZnSnS4 nanoparticles should increase the light energy conversion efficiency and lead to the development of novel low-cost and highly efficient light energy conversion systems.
Acknowledgements
This work was supported by a Funding Program for Next Generation World-Leading Researchers (NEXT Program) and a Grant-in-Aid for Young Scientists (B) (no. 26810043) from the Japan Society for the Promotion of Science.
Notes and references
- K. Ramasamy, M. A. Malik and P. O'Brien, Chem. Commun., 2012, 48, 5703–5714 RSC.
- S. Delbos, EPJ Photovoltaics, 2012, 3, 35004 CrossRef CAS.
- W. Wang, M. T. Winkler, O. Gunawan, T. Gokmen, T. K. Todorov, Y. Zhu and D. B. Mitzi, Adv. Energy Mater., 2014, 4, 1301465 Search PubMed.
- D. A. R. Barkhouse, O. Gunawan, T. Gokmen, T. K. Todorov and D. B. Mitzi, Prog. Photovoltaics, 2012, 20, 6–11 CAS.
- K. Wang, O. Gunawan, T. Todorov, B. Shin, S. J. Chey, N. A. Bojarczuk, D. Mitzi and S. Guha, Appl. Phys. Lett., 2010, 97, 143508 CrossRef PubMed.
- G. Khrypunov, A. Remeo, F. Kurdeasu, D. L. Bätzner, H. Zogg and A. N. Tiwari, Sol. Energy Mater. Sol. Cells, 2006, 90, 664–677 CrossRef CAS PubMed.
- M. Law, J. M. Luther, Q. S. Barbara, K. Hughes, C. L. Perkins and A. J. Nozik, J. Am. Chem. Soc., 2008, 130, 5974–5985 CrossRef CAS PubMed.
- P. Jackson, D. Hariskos, E. Lotter, S. Paetel, R. Wuerz, R. Menner, W. Wischmann and M. Powalla, Prog. Photovoltaics, 2011, 19, 894–897 CAS.
- I. Repins, M. A. Contreras, B. Egaas, C. DeHart, J. Scharf, C. L. Perkins, B. To and R. Noufi, Prog. Photovoltaics, 2008, 16, 235–239 CAS.
- Q. Guo, H. W. Hillhouse and R. Agrawal, J. Am. Chem. Soc., 2009, 131, 11672–11673 CrossRef CAS PubMed.
- Q. Guo, G. M. Ford, W.-C. Yang, B. C. Walker, E. A. Stach, H. W. Hillhouse and R. Agrawal, J. Am. Chem. Soc., 2010, 132, 17384–17386 CrossRef CAS PubMed.
- A. Khare, A. W. Wills, L. M. Ammerman, D. J. Norris and E. S. Aydil, Chem. Commun., 2011, 47, 11721–11723 RSC.
- W. C. Liu, B. L. Guo, X. S. Wu, F. M. Zhang, C. L. Mak and K. H. Wong, J. Mater. Chem. A, 2013, 1, 3182–3186 CAS.
- A. J. Nozik, M. C. Beard, J. M. Luther, M. Law, R. J. Ellingson and J. C. Johnson, Chem. Rev., 2010, 110, 6873–6890 CrossRef CAS PubMed.
- H. Nishi, T. Nagano, S. Kuwabata and T. Torimoto, Phys. Chem. Chem. Phys., 2014, 16, 672–675 RSC.
- H. Nishi, S. Kuwabata and T. Torimoto, J. Phys. Chem. C, 2013, 117, 21055–21063 CAS.
- X. Lu, Z. Zhuang, Q. Peng and Y. Li, Chem. Commun., 2011, 47, 3141–3143 RSC.
- A. Singh, H. Geaney, F. Laffir and K. M. Ryan, J. Am. Chem. Soc., 2012, 134, 2910–2913 CrossRef CAS PubMed.
- M. Li, W.-H. Zhou, J. Guo, Y.-L. Zhou, Z.-L. Hou, J. Jiao, Z.-J. Zhou, Z.-L. Du and S.-X. Wu, J. Phys. Chem. C, 2012, 116, 26507–26516 CAS.
- M.-D. Regulacio, C. Ye, S.
H. Lim, M. Bosman, E. Ye, S. Chen, Q.-H. Xu and M.-Y. Han, Chem. – Eur. J., 2012, 18, 3127–3131 CrossRef CAS PubMed.
- H.-C. Liao, M.-H. Jao, J.-J. Shyue, Y.-F. Chen and W.-F. Su, J. Mater. Chem. A, 2013, 1, 337–341 CAS.
- J. Chang and E. R. Waclawik, CrystEngComm, 2013, 15, 5612–5619 RSC.
- M. J. Thompson, T. P. A. Ruberu, K. J. Blakeney, K. V. Torres, P. S. Dilsaver and J. Vela, J. Phys. Chem. Lett., 2013, 4, 3918–3923 CrossRef CAS.
- Y. Zou, X. Su and J. Jiang, J. Am. Chem. Soc., 2013, 135, 18377–18384 CrossRef CAS PubMed.
- M. Kanehara, H. Arakawa, T. Honda, M. Saruyama and T. Teranishi, Chem. – Eur. J., 2012, 18, 9230–9238 CrossRef CAS PubMed.
- D. Mott, J. Yin, M. Engelhard, R. Loukrakpam, P. Chang, G. Miller, I.-T. Bae, N. C. Das, C. Wang, J. Luo and C.-J. Zhong, Chem. Mater., 2010, 1, 261–271 CrossRef.
- T. Kameyama, T. Osaki, K. Okazaki, T. Shibayama, A. Kudo, S. Kuwabata and T. Torimoto, J. Mater. Chem., 2010, 20, 5319–5324 RSC.
- J. M. Luther, P. K. Jain, T. Ewers and A. P. Alivisatos, Nat. Mater., 2011, 10, 361–366 CrossRef CAS PubMed.
- L. Yi, A. Tang, M. Niu, W. Han, Y. Hou and M. Gao, CrystEngComm, 2010, 12, 4124–4130 RSC.
- S.-K. Han, M. Gong, H.-B. Yao, Z.-M. Wang and S.-H. Yu, Angew. Chem., Int. Ed., 2012, 51, 6365–6368 CrossRef CAS PubMed.
- M. Kruszynska, H. Borchert, J. Parisi and J. Kolny-Olesiak, J. Am. Chem. Soc., 2010, 132, 15976–15986 CrossRef CAS PubMed.
Footnotes |
† Electronic supplementary information (ESI) available: TEM image of nanoparticles produced by thermal decomposition of metal thiolates in DDT at 240 °C for 60 min (Fig. S1); TEM image and XRD pattern of nanoparticles prepared by addition of an equal molar ratio of S and DBTU and subsequent stirring at 200 °C for 180 min (Fig. S2); TEM image and XRD pattern of nanoparticles prepared through the nucleation process at 150 °C for 30 min (Fig. S3). TEM images of nanoparticles obtained after the cystal growth process for 0–180 min (Fig. S4). See DOI: 10.1039/c4ce01852d |
‡ Present address: Institute of Industrial Science, The University of Tokyo, 4-6-1 Komaba, Meguro-ku, Tokyo 153-8505, Japan. E-mail: nishi-h@iis.u-tokyo.ac.jp |
|
This journal is © The Royal Society of Chemistry 2015 |
Click here to see how this site uses Cookies. View our privacy policy here.