DOI:
10.1039/C5CE00397K
(Paper)
CrystEngComm, 2015,
17, 8021-8030
A DAC tartrate-based gelator system featuring markedly improved gelation properties: enhancing lifetime and functionality of gel networks†
Received
26th February 2015
, Accepted 31st March 2015
First published on 3rd April 2015
Abstract
The delicate interface between gelation and crystallization can be exemplified using a multicomponent gelator solution (MGS-I) consisting of (1R,2R)-1,2-diaminocyclohexane L-tartrate (1) in MeOH (0.128 M) and concentrated HCl (2.4 equiv. with respect to 1). Solubilization of 1 occurs due to an ionic dissociation–exchange process induced by HCl. A transient chloride-containing assembly of 1 in solution, resembling that in its crystalline state, acts as a supramolecular synthon for the growth of gel networks in the presence of different organic solvents at low temperature. These gels have very short lifetimes (ca. 1–72 h in most cases) as a consequence of the thermodynamic formation of insoluble (1R,2R)-1,2-diaminocyclohexane dihydrochloride (2). However, a more robust formulation can be obtained by replacing MeOH with DMSO, which in the presence of HCl provides similar solubilization of diaminocyclohexane (DAC) tartrate salt 1, but it delays the destabilization of solvated supramolecular aggregates. The new formulation (MGS-II) offers a series of major advantages in comparison to MGS-I, such as the formation of homogeneous, transparent and more elastic gels within seconds at room temperature and at much lower concentrations. Moreover, MGS-II has high tolerance to the nature of the dicarboxylic acid derivative, which acts as an extender and a stabilizer of the physical network. Very interestingly, MGS-II can selectively gel solvents in multiphasic systems and can be also combined with warmed solutions of other gelators for the preparation of supramolecular hybrid gel systems with superior properties.
Introduction
In the past decade, considerable effort has been devoted to the application of crystal engineering strategies to the design of low molecular weight gelators (LMWG).1 With different examples, advances in this area have demonstrated a connection between the 3D crystal packing of small molecules and their ability to form supramolecular gel networks.1 This is not an obvious link if we consider that the occurrence of crystals is predicted by equilibrium thermodynamics upon crossing the solubility boundary, whereas the gelation phenomenon represents transition kinetics.2 Thus, understanding the underlying physics of the so-called delicate balance between gelation and crystallization has required the development of computational methods3 and models2 that account for the type of interactions, and their lifetimes, governing the competition between particle aggregation, dissociation, and rearrangement processes. This fine interface becomes even more diffuse in multicomponent supramolecular gels.4 However, such compositions offer a more versatile way to tailor the properties of the gel materials by changing the formulation parameters.
On the other hand, among a large number of existing gelator structures,5 LMWG based on organic ammonium salts have emerged as versatile “supramolecular synthons”6–8 for fine-tuning non-covalent molecular aggregation modes by the incorporation of selected functional groups. Such control on the dimensionality of the network9 becomes even more appealing when using multicomponent ammonium-based systems because multicomponent systems add further complexity to hierarchical self-assembly processes that drive the formation of larger aggregates.10 Indeed, in these salts the crystal engineering approach was found to have a unique niche for the development of new functional gels.8
Within this context, we have previously reported a synergistic multicomponent gelator solution (MGS-I), which allowed us to identify an exceptional gel–crystal interface through the formation of short-lived organogels at low temperatures.11 The optimal formulation of MGS-I consisted of a solution of (1R,2R)-1,2-diaminocyclohexane L-tartrate (1) in MeOH (0.128 M) containing 2.4 equiv. of HCl (37 wt.% in H2O). The presence of each component in the mixture and its stoichiometry was found to play a crucial role in the formation of transient gels. The gelation mechanism of MGS-I is based on the kinetic self-assembly of DAC tartrate salt 1via electrostatic interactions and hydrogen-bonding between ammonium nitrogen donors and hydroxyl oxygen acceptors. The hydrochloric acid contributes to the solubilization of 1 by activating a thermodynamic ion exchange process, which ends up with the formation of insoluble (1R,2R)-1,2-diaminocyclohexane dihydrochloride (2) and the subsequent destruction of the gel phase (Fig. 1).
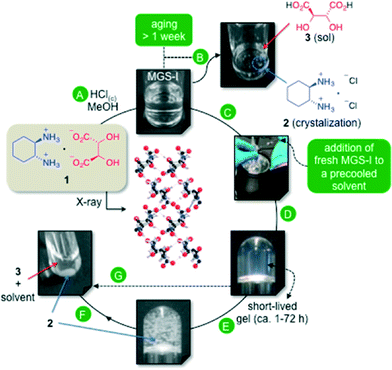 |
| Fig. 1 Formulation of the multicomponent organogelator liquid system MGS-I and its plausible mechanism of action as previously described.11 A) Preparation of MGS-I by dissolution of 1 in MeOH (0.128 M)/HCl(c) (2.4 equiv.). B) Spontaneous crystallization of 2 during aging of MGS-I (aging time >7 days). C) Addition of a small amount of freshly prepared MGS-I to an organic solvent at low temperature to induce gel formation. D) Formation of homogeneous transient organogels. E) Competitive crystallization of 2 over time within the gel matrix. F) Completion of the ion exchange leading to collapse of the gel. G) Aging, thermal or mechanical stress accelerates this process (X-ray structure adapted with permission from ref. 14, copyright © American Chemical Society). | |
In this article, we describe a reformulation of MGS-I that enabled the preparation of similar transient gels at room temperature with superior temporal, thermal and mechanical properties. Moreover, the robustness of the new formulation (MGS-II) allowed the phase selective gelation of multiphasic systems and the preparation of simultaneous interpenetrating supramolecular gel networks.
Experimental procedures
Materials
Unless otherwise indicated, all chemicals and anhydrous solvents were purchased from commercial suppliers and were used as received without further purification. Required stock solutions were always accurately prepared using volumetric flasks. Clean precision glass pipettes or syringes should be used for the accurate preparation and manipulation of HCl-containing solutions. A VWR™ ultrasonic cleaner (USC200TH) was used for solubility tests. The temperature of the ultrasonic water bath was 33 ± 2 °C after 30 min of sonication. trans-(1R,2R)-1,2-Bis(dodecylamido)cyclohexane (4) was synthesized according to the literature procedure12,13 and exhibited identical spectral properties to those reported. Compounds 5–10 (purity >99%) were also purchased from commercial suppliers and used as received.
Preparation of MGS-I
MGS-I was prepared at the optimal molar concentration according to the method described previously.11 Briefly, (1R,2R)-1,2-diaminocyclohexane L-tartrate (253.8 mg, 0.96 mmol) was weighed in a glass vial and dissolved in 7.5 mL of a 0.3 M HCl/MeOH stock solution (this solution was previously prepared using concentrated HCl (37 wt.% in H2O) and dry MeOH). The as-prepared MGS-I isotropic solution (0.128 M with respect to DAC tartrate salt) was colorless and transparent.
Preparation of MGS-II
(1R,2R)-1,2-Diaminocyclohexane L-tartrate (253.8 mg, 0.96 mmol) was weighed in a glass vial and dissolved in 7.5 mL of a 0.3 M HCl/DMSO stock solution (this solution was previously prepared using concentrated HCl (37 wt.% in H2O) and anhydrous DMSO (>99.9%)). The as-prepared MGS-II isotropic solution (0.128 M with respect to DAC tartrate salt) was colorless and transparent. Freshly prepared stock solutions should be used for gelation experiments.
Preparation of gels with MGS-I
The desired solvent (1.0 mL) was placed into a screw-capped round-bottom glass test tube (10 cm in length × 1 cm in diameter, 1 mm in wall thickness) and cooled close to its freezing temperature. After exposing the solvent at low temperature for 5 min, an appropriate volume (see Table 1) of MGS-I was added while the test tube was being stirred gently by hand, and the mixture was kept at low temperature for 1 min. After this, the cooling bath was removed and the resulting clear solution was allowed to warm up to room temperature allowing the formation of the gels.11
Table 1 Comparison of the gelation properties of MGS-I and MGS-IIa
Entry |
Solvent |
MGS-I11 |
MGS-II |
gvb (μL) |
T
mix
(°C) |
T
d
(°C) |
Aspect phasee |
Temporal stabilityf |
gvb (μL) |
T
mix
(°C) |
T
d
(°C) |
Aspect phasee |
Temporal stabilityf |
1 mL of solvent was used in each experiment. Solvent abbreviations: ACT = acetone; ACN = acetonitrile; BN = benzonitrile; CHN = cyclohexanone; DCM = dichloromethane; DEE = diethyl ether; DME = 1,2-dimethoxyethane; DOX = 1,4-dioxane; ETAC = ethyl acetate; MBN = 3-methylbutan-2-one; MEE = 2-methoxyethyl ether; NM = nitromethane; and THF = tetrahydrofuran. Group I for MGS-I = benzene, carbon tetrachloride, dibutyl ether, 1,2-dichloroethane, dimethylacetamide, dimethylformamide, dimethyl sulfoxide, ethanol, ethylene glycol, n-hexane, N-methyl-2-pyrrolidone, toluene, and water. Group I for MGS-II = benzene, dimethylacetamide, dimethylformamide, ethanol, glycerol, methanol, N-methyl-2-pyrrolidone, 2-propanol, toluene, and water. Group II for MGS-I = carbon disulfide, chloroform, cyclohexane, 1,2-dichlorobenzene, glycerol, nitrobenzene, n-octane, and tetrachloroethylene. Group II for MGS-II = cyclohexane and n-hexane. Group III for MGS-I = 1-butyl-3-methylimidazolium hexafluorophosphate (ionic liquid, [BMIM][PF6]), methyl tert-butyl ether, and pyridine. Group III for MGS-II = [BMIM][PF6], pyridine, and chloroform.
Volume of MGS used to obtain reproducible gel samples. This is approximately the minimum volume necessary to achieve gelation.
Temperature of mixing MGS with the tested solvent.
Gel destruction temperature observed by DSC (error = ±2 °C).
Abbreviations: TG = transparent or slightly translucent gel; OG = opaque gel; M = miscible; I = immiscible; and P = precipitate.
Stability of the gels with time when stored undisturbed in a vertical position at 23 ± 2 °C. The ranges were defined by at least 5 random measurements with freshly prepared gelator solutions. Instability was determined when the gel suffered fragmentation or it was partially liquefied without resistance to inversion of the test tube. Abbreviations: d = days and h = hours.
T
d was determined by the Kugelrohr-based method with estimated errors of ±5 °C and ±10 °C for CHN and NM, respectively.
The color of this gel changes from white opaque to dark yellow-green opaque after 1–2 h. No color change was observed with MGS-II.
|
1 |
NM |
100 |
−20 |
95g |
TG |
2–4 h |
40 |
RT |
96 |
OG |
7–9 d |
2 |
ACN |
100 |
−40 |
62 |
OG |
1–3 d |
30 |
RT |
71 |
TG |
1–3 d |
3 |
BN |
150 |
−10 |
152 |
TG |
1–2 h |
50 |
RT |
149 |
TG |
1–2 d |
4 |
ACT |
250 |
−90 |
43 |
OG |
2–5 h |
40 |
RT |
42 |
TG |
8–15 h |
5 |
CHN |
150 |
−10 |
84g |
TG |
2–3 h |
40 |
RT |
123 |
TG |
2–3 h |
6 |
MBN |
100 |
−80 |
68 |
OG |
3–4 dh |
20 |
RT |
75 |
TG |
7–9 d |
7 |
DME |
100 |
−50 |
65 |
OG |
1–4 h |
30 |
RT |
72 |
TG |
3–4 d |
8 |
MEE |
100 |
−60 |
128 |
OG |
3–4 d |
30 |
RT |
129 |
OG |
6–9 d |
9 |
ETAC |
250 |
−78 |
44 |
OG |
9 h–1 d |
20 |
RT |
56 |
TG |
2–3 d |
10 |
THF |
150 |
−78 |
54 |
TG |
4–5 d |
20 |
RT |
56 |
TG |
2–3 d |
11 |
DOX |
80 |
13 |
89 |
TG |
2–3 d |
20 |
RT |
85g |
TG |
1–2 d |
12 |
DEE |
180 |
−100 |
52 |
OG |
3–4 d |
— |
— |
— |
I |
— |
13 |
DCM |
— |
— |
— |
M |
— |
100 |
RT |
45 |
OG |
3–6 h |
14 |
Group I |
— |
— |
— |
M |
— |
— |
— |
— |
M |
— |
15 |
Group II |
— |
— |
— |
I |
— |
— |
— |
— |
I |
— |
16 |
Group III |
— |
— |
— |
P |
— |
— |
— |
— |
P |
— |
Preparation of gels with MGS-II
An appropriate volume of MGS-II (see Table 1) was added into a screw-capped round-bottom glass test tube (the same tubes as those used for the experiments with MGS-I) and a respective solvent (1.0 mL) was added at room temperature. This process was done quickly but carefully to avoid the formation of bubbles. The as-obtained materials were preliminarily classified as gels if they did not exhibit gravitational flow upon turning the test tube upside down at room temperature. The gel state was further confirmed by rheological measurements.
Typical procedure for the phase selective gelation
Pentane (1.0 mL) was layered on top of a respective dry solvent (1.0 mL) placed into a screw-capped round-bottom glass test tube (the same tubes as those above-mentioned were used). An appropriate volume of MGS (i.e., MGS-I or MGS-II) was added via a syringe (see Table 1). The mixture was homogenized by quickly shaking the solution by hand. Gel formation was confirmed after 5 min by turning the test tube upside down and removing the liquid phase (pentane).
Representative procedure for the preparation of hybrid gels
MGS-II (24 μL (a) or 40 μL (b)) was placed into a screw-capped round-bottom glass test tube (10 cm length × 1 cm diameter, 1 mm wall thickness) and slightly warmed via a heat gun. Diamide gelator 4 (0.8 mg (a) or 1.0 mg (b)) was dissolved in acetone (1.0 mL) with gentle heating. Both solutions were mixed via a syringe before reaching room temperature. The formation of bubbles was avoided by fast and consistent addition. Complete gelation was achieved after cooling down the hybrid solution to room temperature.
Characterization of gel-based materials
Differential scanning calorimetry (DSC) measurements were performed using a Perkin-Elmer DSC7. The DSC thermograms were obtained under dynamic nitrogen atmosphere (gas flow rate = 20 mL min−1) at a heating rate of 3 °C min−1. Samples were placed on open aluminum pans (Perkin-Elmer) and an empty sample holder was used as a reference. Thermograms were obtained by heating the samples from 25 to 60–170 °C, depending on each sample. The reported values correspond to the average of two independent measurements. A Büchi GKR-50 Kugelrohr apparatus was used to estimate the gel destruction temperatures (Td) and these were compared with the DSC thermograms.11 Field emission scanning electron microscopy (FESEM) images of xerogels (i.e., freeze-dried gel samples) were obtained with a Zeiss Merlin field emission scanning electron microscope operated at an accelerating voltage of 10 kV. The samples were sputtered (40 mA, 60 seconds) with Pt (film thickness ~ 10 nm) before imaging with a SCD500 Leica EM. Dynamic (oscillatory) rheological measurements were performed using a 20 mm plate (stainless steel) with plain geometry in an advanced rheometer AR 2000 (TA Instruments) equipped with a cooling system (Julabo C). The samples were coated with a very thin layer of low viscosity oil to avoid solvent evaporation during the measurements. In general, dynamic strain sweep (DSS) measurements were first carried out between 0.1–1% and 100% strain at 1 Hz frequency to determine the strain value at which convenient torque values were given (i.e., about 10 times the transducer resolution limit). Dynamic frequency sweep (DFS) measurements (i.e., from 0.1 to 10 Hz at 0.1% strain) and dynamic time sweep (DTS) measurements within the viscoelastic regime (i.e., 0.1–1% strain, 1 Hz frequency) were subsequently performed. Turbidity values were obtained using a Camlab CW8100 turbidimeter equipped with an infrared LED as a light source (λ = 860 nm) and a photodiode scattered light detector at an angle of 90°. Compound 2 formed either with MGS-I or MGS-II was clearly characterized by NMR and X-ray analyses (ESI†).
Results and discussion
In our previous report11 we unequivocally established the optimal composition of MGS-I necessary for the preparation of different organogels, albeit with rather short lifetimes (between 1 and 72 h in most cases). The formulation of MGS-I consisted of a 0.128 M methanolic solution of (1R,2R)-1,2-diaminocyclohexane L-tartrate (1) and 2.4 equiv. of concentrated HCl (37 wt.% in H2O). The corresponding molar ratio of all components (i.e., (1R,2R)-1,2-diaminocyclohexane L-tartrate
:
MeOH
:
HCl
:
H2O = 1
:
193
:
2.4
:
8.3) was proved essential for the gelation process. The corresponding organogels were formed upon addition of small volumes of MGS-I to a variety of organic solvents (i.e., 80–250 μL of MGS-I per milliliter of solvent). Due to the extremely fast kinetics of the gelation process, it was necessary to cool down the solvents close to their freezing points prior to the addition of MGS-I. This procedure allowed the preparation of isotropic solutions containing MGS-I and an appropriate solvent (see Table 1), which further turned into homogeneous gels upon warming up to room temperature. Nevertheless, a small portion of the liquid always remained non-gelled (ca. 5–10 vol.%) regardless of the amount of MGS-I. It is important to note that if the solvents are not cooled only partial gel-like materials are obtained with MGS-I. The introduction of a thermometer probe into the systems demonstrated that the gelation usually started below 0 °C, but well above the mixing temperature.
The proposed mechanism for the formation of transient gels upon mixing MGS-I with some organic solvents is based on the intrinsic bidirectional hydrogen-bonding polymeric network of the chiral ammonium tartrate salt 1 (Fig. 2).14 Although this ion-paired carboxylate–ammonium aggregate is insoluble in MeOH, the addition of aqueous HCl triggers a thermodynamic anion exchange process that culminates with the precipitation of (1R,2R)-1,2-diaminocyclohexane dihydrochloride (2) and the formation of L-tartaric acid (3), which remains in solution (Fig. 1). Nevertheless, the highly solvated15 and complex multicomponent network formed initially constitutes a short-lived metastable phase, which may act as a “supramolecular synthon”16 when it is combined with another solvent that is non-protic and less polar than MeOH. Hence, although the solubility of the chloride-bridged multicomponent aggregate decreases, it can persist for some time within the dense hydrogen-bonded network. This leads to the formation of colloidal-like gels17 that finally collapse due to the inexorable formation of 2via ion exchange.
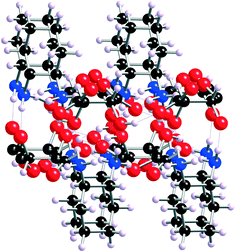 |
| Fig. 2 X-Ray structure of the network formed by 1. The image corresponds to a side view down the b axis showing the bilayered core (adapted with permission from ref. 14, copyright © American Chemical Society). | |
Clearly, the formation of these transient gels depends on the ability to preserve a judicious balance between multiple non-covalent interactions for as long as possible. Therefore, we hypothesized that a reformulation of MGS-I based on the replacement of MeOH with a different solvent (i.e., non-protic polar solvent) could reduce the disruption of the parent hydrogen-bonding network in 1, with the consequent enhancement of the lifetime of the “supramolecular synthon” and, therefore, of the metastable gel phases.
Formulation of MGS-II and gel preparation
Preliminary solubility screening showed that MeOH in MGS-I could be replaced (keeping the stoichiometry and other components constant) with four other solvents, namely dimethyl sulfoxide (DMSO), N,N-dimethylformamide (DMF), N,N-dimethylacetamide (DMA) and N-methyl-2-pyrrolidone (NMP). However, the solutions obtained with DMF, DMA and NMP showed color change and precipitation of the corresponding diaminocyclohexane dihydrochloride within 1 day, whereas the solution in DMSO remained clear and homogeneous for several months (turbidity <0.3 NTU) (Fig. 3).
 |
| Fig. 3 Replacement of MeOH in MGS-I with different solvents (i.e., DMF = dimethylformamide, DMA = dimethylacetamide, NMP = N-methyl-2-pyrrolidone, DMSO = dimethyl sulfoxide). Note: salt 1 remained insoluble in all solvents in the absence of HCl, even after ultrasonic treatment at 50 °C for several hours. | |
Similar to the formulation study made with MGS-I,11 two-variable screening including DAC tartrate salt concentrations and HCl equivalents was carried out in order to optimize the composition of MGS-II based on DMSO (Table S1, ESI†). As expected, the results demonstrated that at least 2.0 equiv. of HCl was also necessary to dissolve 1 in DMSO. Under these conditions, and contrary to MGS-I, transparent (at least for several weeks) solutions in DMSO could be prepared even at concentrations of 1 as high as 0.3 M (ca. 2.3 times higher than in the case of MGS-I). In comparison with the gelation ability of MGS-I,11 MGS-II was also prepared at [1] = 0.128 M and 2.4 equiv. of concentrated HCl with respect to 1.
For the preparation of organogels, the desired solvent was quickly added to the specific volume of fresh MGS-II in a glass test tube (vide infra) at room temperature. This constitutes one major difference with MGS-I, which required precooling of the solvent close to its freezing point in order to reduce the gelation kinetics and obtain homogeneous gels.11 It is important to mention that addition of MGS-II to the solvent, instead of the other way around, usually resulted in solution or partial gelation. Substantial screening under experimental conditions was carried out in order to optimize the protocol for the preparation of the gels (Table S2, ESI†).
Gelation ability
The gelation ability of MGS-II was evaluated for a large variety of solvents and compared to that of MGS-I (Table 1). Materials that did not exhibit gravitational flow upon turning the test tube upside down at room temperature were classified as gels. This state was further confirmed by oscillatory rheological measurements (vide infra).
As shown in Table 1, MGS-II was able to gel the same oxygenated and nitrogenated organic solvents as MGS-I at very low concentrations (entries 1–11). The only exceptions were diethyl ether (DEE) and dichloromethane (DCM), which were gelled by only one of the MGS systems (entries 12–13). In general, intermediate hydrogen-bonding acceptor solvents with moderate polarizability showed a higher tendency to form gels.18 In most cases, gelation took place within seconds. Similar to MGS-I, formation of stable gels was not observed with most halogenated, aromatic and hydrocarbon solvents (entries 14–16). Herein, the term “stable gel” refers to the absence of gravitational flow upon inversion of the vial and the absence of crystals of 2. In contrast to MGS-I, removal of a small portion of the remaining non-gelled liquid (ca. 5 vol.%) usually observed in these materials did not enhance the temporal stability of the gels obtained with MGS-II.
We were delighted to observe that the volume of MGS-II necessary to obtain reproducible gel samples (gelation volume, gv) was established in the range of 20–100 μL, which corresponds to concentrations as low as ca. 0.08–0.4 wt.% of DAC tartrate salt 1. This means that, for example, more than 4800 molecules of THF are immobilized per molecule of 1. These values are much smaller than those determined with MGS-I (i.e., 80–250 μL). The most salient example was found with ethyl acetate (entry 9), which needed up to 12 times less volume of MGS-II than MGS-I. For all other solvents the decrease in gelation volume was at least 2 times when using MGS-II. Interestingly, each solvent showed a very narrow range of MGS volume that provided stable gels (e.g., gels in acetonitrile could be prepared using either 100 μL of MGS-I or 30 μL of MGS-II (entry 2); however, the use of 100 μL of MGS-II provided only a clear solution). This prevented a direct comparison of gels obtained with MGS-I and MGS-II at the same vol.%. Therefore, we focused our comparative studies on gels prepared at their corresponding gv as indicated in Table 1.
Similar to MGS-I,11 FT-IR gel-based materials derived from MGS-II also displayed the expected stretching vibration bands of both carboxylic (ca. 1710–1750 cm−1) and ammonium (ca. 2350–3300 cm−1) groups, as well as the potential hydrogen bond association bands in the range of 3200–3600 cm−1. However, no further conclusive information could be obtained due to the overlap of alkane, water and DMSO stretching bands in all these regions.
Appearance of the gels
Only 18% of the gels obtained with MGS-I were optically transparent or slightly turbid (entries 1, 3, 5, and 10–11) (Fig. 4 and S1, ESI†), whereas this condition was observed in 82% of the gels prepared with MGS-II (entries 2–7 and 9–11). This result suggests that the replacement of MeOH with DMSO in the formulation of the gelator solution allows the construction of stable gel networks with formation of smaller aggregates at the gel point, leading to higher transparency.
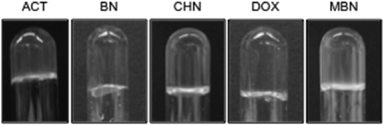 |
| Fig. 4 Selection of photographs of gels made with MGS-II in various organic solvents (i.e., ACT = acetone; BN = benzonitrile; CHN = cyclohexanone; DOX = 1,4-dioxane; and MBN = 3-methylbutan-2-one). | |
Temporal stability of the gels
The growth of (1R,2R)-1,2-diaminocyclohexane dihydrochloride (2) crystals inside the gel matrices11 was proved to be directly associated with the gel weakening and subsequent collapse upon aging (Fig. 5 and S8, ESI†). Remarkably, the use of MGS-II notably reduced the gel-to-crystal transition kinetics (a process related to Ostwald's rule of stages)19 in most cases.
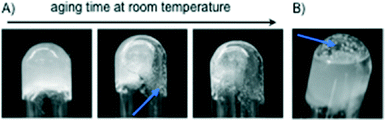 |
| Fig. 5 Competitive crystallization-induced gradual destruction of gels made with (A) [MGS-II + dichloromethane] and (B) [MGS-I + ethyl acetate]11 (Table 1). Regardless of the solvent, crystals (blue arrows) of 2 were formed over time through the entire gel matrix in the case of MGS-II, whereas solvent cavities at the bottom of the tube and large crystals were typically observed in the case of MGS-I. These cavities accelerated the collapse of the gel upon inversion of the tube in comparison to materials prepared with MGS-II where the bulk gel remained more compact. | |
Only organogels prepared in tetrahydrofuran or 1,4-dioxane exhibited relatively lower temporal stabilities than their counterparts prepared with MGS-I (entries 10–11), and no major differences were observed for the gels prepared in acetonitrile or cyclohexanone (entries 2 and 5). Most of the other gels with temporal stabilities of only a few hours (when prepared with MGS-I) were now stable for several days. In some solvents such as nitromethane and 1,2-dimethoxyethane the enhancement factor was established between 24 and 64 (or 2400–6400%) (entries 1 and 7).
In order to accurately evaluate the temporal stability of the gels, the test vials should be sealed to avoid changes in the concentration of the species by solvent evaporation and weakening of the network by water absorption. Indeed, the incorporation of water into these materials (ca. 5–20 μL of water per milliliter of solvent) is enough to destroy the gels (if the water is layered on top) or avoid their formation (if the water is added to MGS). In contrast, most of the gels remain stable when additional organic solvent is layered on top (Tables S3–S4 and Fig. S2, ESI†).
Thermal stability of the gels
In contrast to other supramolecular gels, the materials prepared with MGS-I or MGS-II are not thermoreversible. In general, application of mechanical or thermal stress accelerates the ion exchange causing the precipitation of insoluble 2. The temperature at which the destruction of the gel took place upon heating (Td) was accurately visualized by DSC measurements (first endothermic phase transition) (Fig. 6 and S3, ESI†). As shown in Table 1, MGS-II usually provided gels with higher thermal stability than that of MGS-I (i.e., ΔTdca. 2–10 °C). The most remarkable example corresponded to the gels made in cyclohexanone (entry 5), whose Td was enhanced almost 40 °C when using MGS-II instead of MGS-I at their corresponding gv.
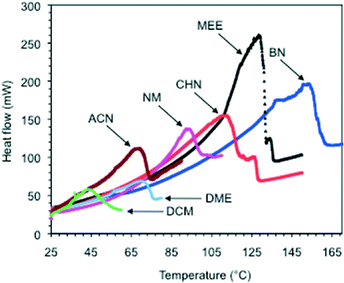 |
| Fig. 6 Selection of DSC thermograms of gels made with MGS-II in different solvents. Each DSC plot corresponds to the average of two measurements. For abbreviations, see Table 1. | |
Effect of DAC tartrate salt on the gelation ability of MGS-II
Similar to MGS-I,11 gels prepared from MGS-II were extremely sensitive to the enantiomeric purity of the ammonium tartrate salt. Complete gelation and stable gels upon inversion of the test tube could be only obtained with enantiomerically pure systems (Fig. 7). This result points out the critical importance of chirality in the formation of multicomponent “supramolecular synthons” for the growth of gel networks, at least with the described formulation.20
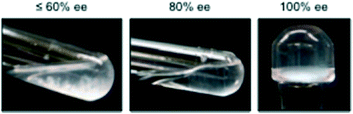 |
| Fig. 7 Effect of the enantiopurity of 1 (ee = enantiomeric excess) on the gelation ability of MGS-II. The pictures correspond to the materials prepared in THF as described in Table 1. However, the same behavior was observed as with other solvents. | |
Very surprisingly, the effect of replacing the L-tartaric acid component with a number of different dicarboxylic acid derivatives, 5–10, was very different for each MGS. While a major reduction of the temporal stability of model gels made with MGS-I was observed, MGS-II enabled the replacement of the diacid component without major detriment to the lifetime of the gels, even with non-chiral diacid derivatives (Fig. 8 and S4–S5, ESI†). On the other hand, the replacement of the (1R,2R)-1,2-diaminocyclohexane component (11) with other diamines in the formulations was challenging for both MGS gelator systems. Turbid gelator solutions or precipitation after combination with organic solvents was routinely observed with different diamines (Fig. S6, ESI†). However, MGS-II was still able to build a gel network with a lifetime around 2 days after replacing 11 with its diastereomer (1R,2S)-1,2-diaminocyclohexane. As expected, a simple solution of 11 in DMSO did not provide any gel-like material. However, the addition of 2.4 equiv. of HCl to this solution enabled the formation of gel-like materials in some solvents, albeit with low stability (Fig. S7, ESI†).
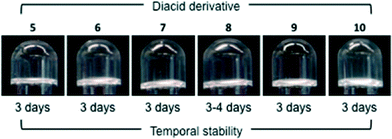 |
| Fig. 8 Gelation of THF (1.0 mL) with reformulated MGS-II (20 μL), in which the tartaric acid component was replaced with other diacid derivatives, namely diethyl L-tartrate (5), succinic acid (6), adipic acid (7), dibenzoyl L-tartrate (8), phthalic acid (9) and L-malic acid (10). In the case of MGS-I, the highest stability (~10 h) was achieved with dibenzoyl L-tartrate and L-malic acid.11 Note that salts made with compounds 5–10 and tartaric acid are expected to have different solubilities than 1, although they were not quantified in this study. | |
These results demonstrated that MGS-II constitutes a gelator system with remarkable higher flexibility toward the use of alternative components for the construction of 3D gel networks. This is appealing because it opens a new door for fine-tuning the mechanical properties and functionality of these materials.
Morphological features of freeze-dried gels
FESEM imaging was used to gain insight into the microstructure of the supramolecular aggregates (Fig. 9). Highly packed basalt-like agglomerates (ca. 2–3 μm in diameter for individual features) were frequently observed in xerogels derived from MGS-I.11 On the other hand, visualization of materials prepared from MGS-II was much more challenging than those from MGS-I because of (1) the extremely low gelator concentration and (2) the presence of DMSO, which was very difficult to remove under vacuum. Despite these difficulties, it was possible to obtain some images of the freeze-dried gel made in dichloromethane (Fig. 9), which showed the presence of a very dense and crumbly structure without basalt-like nanostructures like in the case of MGS-I. In any event, it should be considered that the apparent absence of self-organized fibers could be also due to low magnification and/or high level of stress applied to the samples during their preparation (e.g., artifacts could be generated by formation of tiny crystals during the extensive drying process).
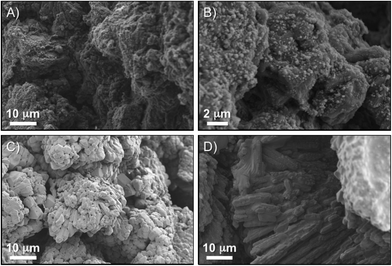 |
| Fig. 9 Representative FESEM images of xerogels prepared from the corresponding gels made under different conditions as depicted in Table 1. A–B) Gelator = MGS-II, solvent = DCM. C) Gelator = MGS-I, solvent = BN. D) Gelator = MGS-I, solvent = MBN. | |
Oscillatory rheological measurements of model gels
Rheological characterization of model gels prepared in different solvents (Fig. S9, ESI†) included dynamic frequency sweep, dynamic strain sweep and dynamic time sweep experiments (DFS, DSS and DTS, respectively). The results confirmed the viscoelastic and brittle nature of the gels with the storage modulus (G′) about one order of magnitude higher than the loss modulus (G′′) within the linear regime (see the Experimental procedures section) regardless of the gelator solution. No significant frequency dependence of the storage modulus was observed, although certain dependence was characteristic of samples with very short lifetimes (i.e., <24 h).11 In general, gels prepared with MGS-II also showed much higher frequency and strain resistance than the counterparts made with MGS-I, as well as a more elastic behavior as indicated by significantly lower average tan
δ values (e.g., gels made in 1,4-dioxane as described in Table 1 showed tan
δ(MGS-II) ~ 0.125 and tan
δ(MGS-I) ~ 0.285; gels made in THF broke at 2% and 10% strain when prepared with MGS-I and MGS-II, respectively).
According to the transient nature of the gels, DTS plots showed a decrease in moduli regardless of the gelator solution used to prepare the materials. However, this reduction was remarkably lower for the gels prepared with MGS-II. For instance, G′ of the gel made in tetrahydrofuran with MGS-I decreased over 76% of the initial value in 10 min.11 In contrast, the reduction was only 33% for the gel prepared with MGS-II (Fig. 10). These results are in good agreement with the higher shear tolerance of gel networks made with MGS-II.
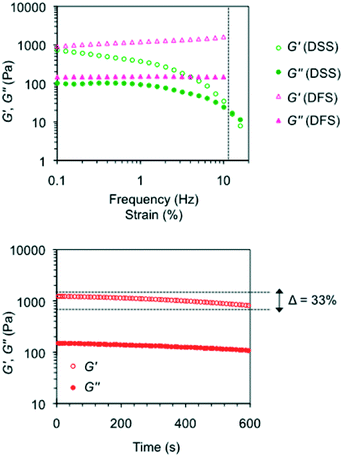 |
| Fig. 10 Representative DFS, DSS (top) and DTS (bottom) rheological measurements of a model gel prepared in 1,4-dioxane using MGS-II. A relatively constant tan δ value (Δ ~ 0.01–0.02), associated with the damping properties of the materials, was observed during short-term experiments. However, a gradual increase was evident with extended periods of stress. | |
Phase selective gelation (PSG)
The higher robustness of MGS-II compared to MGS-I was also accompanied by interesting phase selective gelation ability.21 Biphasic organic mixtures containing a gellable solvent (i.e., nitromethane, acetonitrile, benzonitrile, acetone, and 1.4-dioxane) and a non-gellable solvent (e.g., n-pentane, n-hexane, and 1,2-dichlorobenzene) were selectively gelled by MGS-II at room temperature according to the corresponding gv (Table 1). In sharp contrast, MGS-I provided only partial and/or non-selective gelation (i.e., part of the non-gellable phase was entrapped within the gelled phase) in most cases. Complete and selective gelation of the gellable solvent was achieved even with more challenging triphasic systems, in which a second non-gellable solvent with lower density was layered on top of the above-mentioned biphasic mixture (Fig. 11). Importantly, rheological data of the gelled phase isolated from PSG experiments were in good agreement with the values obtained for the gel prepared using the standard procedure. For instance, the gel made in nitromethane as described in Table 1 showed G′ = 7554 ± 1117 Pa, G′′ = 1617 ± 320 Pa, and tan
δ = 0.213 ± 0.029, whereas the gel obtained as a result of phase selective gelation showed G′ = 8039 ± 207 Pa, G′′ = 2292 ± 607 Pa, and tan
δ = 0.213 ± 0.019 (Fig. S9, ESI†).
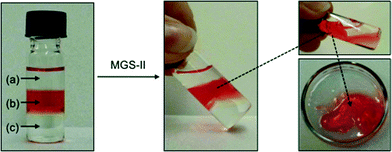 |
| Fig. 11 Phase selective gelation in a triphasic system consisting of a nitromethane phase (b) placed between two phases of different densities, namely hexane (a) and dichlorobenzene (c). Volume of each phase = 1.0 mL. The addition of MGS-II (40 μL) caused full gelation of the nitromethane phase (b). A tiny amount of Disperse Red 1 was added to phase b for better visualization. | |
Supramolecular gel hybrids
Finally, the robustness and superior performance of MGS-II motivated us to do a preliminary investigation into the possibility of fabricating hybrid gels based on the simultaneous growth of two different supramolecular networks. Thus, separation of both networks would only occur if the set of non-covalent interactions through the hybrid network were somehow destroyed. Our previous attempts to obtain reproducible hybrid gels using MGS-I were fruitless due to highly incompatible gelation kinetics with different supramolecular gel networks.
As a proof of concept, we chose trans-(1R,2R)-1,2-bis(dodecylamido)cyclohexane (4), a well-known low molecular weight organogelator,12 to build the additional supramolecular network. A certain structural analogy between 4 and MGS-II, together with their similar gelation abilities, made their combination apparently possible in terms of network compatibility. A series of first round experiments demonstrated that warm solutions of 4 and MGS-II could be mixed prior to gel formation (pre-heating conditions failed with MGS-I) (Table S2, ESI†). The formation of a new gel with a hybrid morphology could be clearly visualized by FESEM imaging, where the crumbly texture derived from MGS-II pervades the fibers from the self-assembly of 4 (Fig. 12). Even when working very slightly below the minimum gelation concentration for both gelators (under these conditions, very weak/partial supramolecular gel networks could still be formed), it was possible to obtain a stable hybrid gel. Although the formation of this hybrid did not prevent the formation of 2 over time, it was delayed as indicated by DTS experiments. In addition, the storage modulus of the hybrid material was ca. one order of magnitude higher than the gel prepared only with 4, albeit to the detriment of some degree of flexibility (Fig. 13, ESI†). Considering the narrow gelation concentration range for MGS, the formation of such hybrids offers new possibilities for the preparation of new MGS-based networks with desired thermal and mechanical properties. Although such systems could be preliminarily defined as a sort of “simultaneous interpenetrating supramolecular networks”,22 in a certain analogy with classical interpenetrating polymer networks,23 further investigation is still necessary in order to fully characterize the structure of these hybrids.
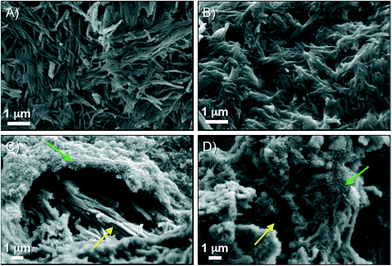 |
| Fig. 12 FESEM images of xerogels prepared with (A–B) a gel made in acetone using 4 (c = 1.0 wt.%) and (C–D) a hybrid gel made in acetone using a mixture of MGS-II and 4. Arrows highlight the coalescence of two structural networks. | |
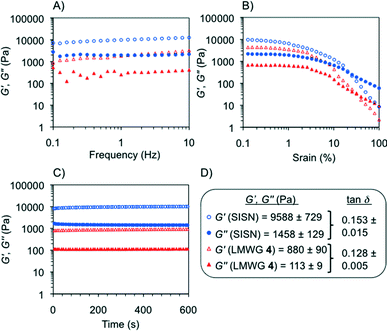 |
| Fig. 13 Comparative rheological measurements of the gel made in acetone (1.0 mL) using 4 (1.0 mg) and the hybrid made using [MGS-II (40 μL) + 4 (1.0 mg)]. A) DFS experiment. B) DSS experiment. C) DTS experiment. D) Overview of average moduli and tan δ values. | |
Conclusions
A well-defined multicomponent gelator solution (MGS-I) consisting of (1R,2R)-1,2-diaminocyclohexane L-tartrate (1) in MeOH (0.128 M) and concentrated HCl (2.4 equiv.) allows the formation of organogels from oxygenated and nitrogenated solvents at low temperature. The formation of a transient chloride-containing assembly of 1 in solution, resembling that in the crystalline state, is the driving force for the observed gelation phenomenon. Experimental evidence have demonstrated that (1R,2R)-1,2-diaminocyclohexane is the major structural director of the assembly process, whereas the tartaric acid partner acts as an important extender and stabilizer of the supramolecular network. These supramolecular gels are characterized by short lifetimes (1–72 h in most cases) defined by the thermodynamic formation of insoluble (1R,2R)-1,2-diaminocyclohexane dihydrochloride (2) via the HCl-induced ionic dissociation–exchange process. However, replacement of MeOH with DMSO in the formulation of the gelator causes a remarkable enhancement of the lifetime of the transient gels. In the presence of HCl, DMSO provides similar solubilization of 1 to that of MeOH, but its non-protic polar nature drastically delays the destabilization of solvated supramolecular aggregates by reducing the disruption of the pattern hydrogen-bonding network.
Moreover, the robustness of the new formulation (MGS-II) offers a series of additional advantages in comparison to MGS-I, namely: a) homogeneous gels can be formed within seconds at room temperature, with the consequent lower cost of the technique; b) the minimum gelation volumes of MGS-II are also much lower (up to 12 times lower in some cases); c) most gels prepared with MGS-II have higher mechanical elasticity and are optically transparent, expanding the potential uses of these materials; d) the replacement of the tartaric acid component in MGS-II with different dicarboxylic acid derivatives also allows the formation of gels with practically the same temporal stability; e) MGS-II displays a remarkable phase selective gelation ability in bi- or triphasic systems; and f) warmed MGS-II can be combined with warmed solutions of other LMWG for the fabrication of supramolecular hybrid gel systems.
Advanced computational studies to determine the exact nature of the supramolecular nucleating species24 and the precise dynamic of MGS-based elusive supramolecular networks are currently ongoing in our laboratories. These systems constitute a unique and resourceful platform for the study of subtle interfaces during molecular crystallization.
Acknowledgements
Financial support from DFG (PRJ 9209720), Universität Regensburg (Anschubfinanzierung von Wissenschaftlichen Projekten) and CSIC (PRJ PIE200980I059) is gratefully acknowledged. The authors acknowledge the assistance of Dr. J. Bachl (synthesis of compound 4), Prof. Dr. A. Göpferich (rheological measurements) and PD Dr. R. Müller (DSC measurements). D.D.D. thanks DFG for the Heisenberg Professorship Award and Prof. José Juan Marrero-Tellado for his exemplary professional conduct and valuable contribution made over the past several years for the development of MGS-I.
Notes and references
- T. K. Adalder and P. Dastidar, Cryst. Growth Des., 2014, 14, 2254 Search PubMed; T. K. Adalder, U. K. Das, J. Majumder, R. Roy and P. Dastidar, J. Indian Inst. Sci., 2014, 94, 9 Search PubMed; T. K. Adalder, D. P. Kumar and P. Dastidar, Cryst. Growth Des., 2014, 14, 11 Search PubMed; S. Banerjee, N. N. Adarsh and P. Dastidar, Soft Matter, 2012, 8, 7623 RSC; N. N. Adarsh and P. Dastidar, Cryst. Growth Des., 2011, 11, 328 Search PubMed; N. N. Adarsh, P. Sahoo and P. Dastidar, Cryst. Growth Des., 2010, 10, 4976 Search PubMed.
- N. M. Dixit and C. F. Zukoski, Phys. Rev. E: Stat., Nonlinear, Soft Matter Phys., 2003, 67, 061501 CrossRef PubMed.
- D. J. Adams, K. Morris, L. Chen, L. C. Serpell, J. Bacsa and G. M. Day, Soft Matter, 2010, 6, 4144 RSC.
- J. Raeburn and D. J. Adams, Chem. Commun., 2015, 51, 5170 RSC; L. E. Buerkle and S. J. Rowan, Chem. Soc. Rev., 2012, 41, 6089 RSC.
-
D. K. Smith, Self-assembling fibrillar networks—Supramolecular gels, in Supramolecular Chemistry: From Molecules to Nanomaterials, ed. J. W. Steed and A. P. Gale, Wiley & Sons Ltd., Chichester, UK, 2012, 1st edn, vol. 7, pp. 3355–3376 RSC; D. D. Díaz, D. Kühbeck and R. J. Koopmans, Chem. Soc. Rev., 2011, 40, 427 RSC; A. Ajayaghosh, V. K. Praveen and C. Vijayakumar, Chem. Soc. Rev., 2008, 37, 109 RSC; D. K. Smith, Chem. Commun., 2006, 34 RSC; N. M. Sangeetha and U. Maitra, Chem. Soc. Rev., 2005, 34, 821 RSC.
- G. R. Desiraju, Angew. Chem., Int. Ed. Engl., 1995, 34, 2311 CrossRef CAS.
- G. R. Desiraju, J. Am. Chem. Soc., 2013, 135, 9952 CrossRef CAS PubMed.
- U. K. Das, S. Banerjee and P. Dastidar, Chem. – Asian J., 2013, 8, 3022 CrossRef CAS PubMed; U. K. Das and P. Dastidar, Cryst. Growth Des., 2013, 13, 4559 Search PubMed; U. K. Das, V. G. Puranik and P. Dastidar, Cryst. Growth Des., 2012, 12, 5864 Search PubMed; P. Sahoo and P. Dastidar, Cryst. Growth Des., 2012, 12, 5917 Search PubMed; T. K. Adalder, N. N. Adarsh, R. Sankolli and P. Dastidar, Beilstein J. Org. Chem., 2010, 6, 848 CrossRef PubMed; D. R. Trivedi and P. Dastidar, Cryst. Growth Des., 2006, 6, 2114 Search PubMed.
- K. Sada, T. Tani and S. Shinkai, Synlett, 2006, 15, 2364 CrossRef CAS; D. R. Trivedi and P. Dastidar, Chem. Mater., 2006, 18, 1470 CrossRef.
- A. R. Hirst and D. K. Smith, Chem. – Eur. J., 2005, 11, 5496 CrossRef CAS PubMed.
- I. Kapoor, E.-M. Schön, J. Bachl, D. Kühbeck, C. Cativiela, S. Saha, R. Banerjee, S. Roelens, J. J. Marrero-Tellado and D. D. Díaz, Soft Matter, 2012, 8, 3446 RSC.
- K. Hanabusa, M. Yamada, M. Kimura and H. Shirai, Angew. Chem., Int. Ed. Engl., 1996, 35, 1949 CrossRef CAS.
- H. Sato, T. Nakae, K. Morimoto and K. Tamura, Org. Biomol. Chem., 2012, 10, 1581 Search PubMed.
- S. Hanessian, M. Simard and S. Roelens, J. Am. Chem. Soc., 1995, 117, 7630 CrossRef CAS.
- A. M. Rijs, N. Sändig, M. N. Blom, J. Oomens, J. S. Hannan, D. A. Leigh, F. Zerbetto and W. J. Buma, Angew. Chem., Int. Ed., 2010, 49, 3896 CrossRef CAS PubMed.
- P. Sahoo, N. N. Adarsh, G. E. Chacko, S. R. Raghavan, V. G. Puranik and P. Dastidar, Langmuir, 2009, 25, 8742 CrossRef CAS PubMed.
- E. Zaccarelli, J. Phys.: Condens. Matter, 2007, 19, 323101 CrossRef.
- W. Edwards, C. A. Lagadec and D. K. Smith, Soft Matter, 2011, 7, 110 RSC.
- V. J. Anderson and H. N. W. Lekkerkerker, Nature, 2002, 416, 811 CrossRef CAS PubMed; P. Zhu, X. Yan, Y. Su, Y. Yang and J. Li, Chem. – Eur. J., 2010, 16, 3176 CrossRef PubMed.
- Z. Dzolic, K. Wolsperger and M. Zinic, New J. Chem., 2006, 30, 1411 RSC.
- Rajkamal, D. Chatterjee, A. Paul, S. Banerjee and S. Yadav, Chem. Commun., 2014, 50, 12131 RSC; S. Basak, J. Nanda and A. Banerjee, J. Mater. Chem., 2012, 22, 11658 RSC; D. R. Trivedi, A. Ballabh, P. Dastidar and B. Ganguly, Chem. – Eur. J., 2004, 10, 5311 CrossRef CAS PubMed; S. R. Jadhav, P. K. Vemula, R. Kumar, S. R. Raghavan and G. John, Angew. Chem. Int. Ed., 2010, 49, 7597 CrossRef; S. Bhattacharya and Y. Krishnan-Ghosh, Chem. Commun., 2001, 185 RSC.
-
S. R. Batten, Interpenetration, in Supramolecular Chemistry: From Molecules to Nanomaterials, ed. P. A. Gale and J. W. Steed, John Wiley & Sons, United Kingdom, 2012, pp. 3107–3120 Search PubMed.
- E. S. Dragan, Chem. Eng. J., 2014, 243, 572 CrossRef CAS.
- P. Jonkheijm, P. van der Schoot, A. P. H. J. Schenning and E. W. Meijer, Science, 2006, 313, 80 CrossRef CAS PubMed.
Footnote |
† Electronic supplementary information (ESI) available: Additional experimental details, figures and tables. See DOI: 10.1039/c5ce00397k |
|
This journal is © The Royal Society of Chemistry 2015 |
Click here to see how this site uses Cookies. View our privacy policy here.