DOI:
10.1039/C4MT00264D
(Paper)
Metallomics, 2015,
7, 174-187
Differential expression of microRNAs by arsenate and arsenite stress in natural accessions of rice†
Received
6th October 2014
, Accepted 18th November 2014
First published on 18th November 2014
Abstract
Arsenic (As) contamination of rice (Oryza sativa) imposes a serious threat to human health worldwide. Understanding the molecular mechanisms of As transport and accumulation in rice may provide promising solutions to the problem. MicroRNAs (miRNAs) are a novel class of short, endogenous, non-coding small RNA molecules involved in a wide variety of biological processes such as organ polarity, morphogenesis, floral transition, hormone signalling and adaptation to environment. In the past, a few studies led to the identification of differentially expressed miRNAs in rice in response to arsenite (As(III)) stress. However, studies related to differential miRNA expression involving natural rice accessions exposed to different species of As have not been carried out. Such studies are required to identify As-species responsive miRNAs in different rice accessions. In this study, we have carried out miRNA profiling in contrasting As accumulating rice accessions using miRNA Array. We report identification of differentially expressed miRNAs in contrasting As accumulating rice cultivars in response to As(III) (25 μM) and As(V) (50 μM) stress. A significant up-regulation in expression was observed among members of the miR396, miR399, miR408, miR528, miR1861, miR2102 and miR2907 families in response to As(III) and As(V) stress in both cultivars. In addition, members of the miR164, miR171, miR395, miR529, miR820, miR1432 and miR1846 families were down-regulated. The differentially expressed miRNAs were subjected to validation of expression and bioinformatic analyses to predict and categorise the key miRNAs and their target genes involved in As stress. Analysis suggests that As-species and rice accession specific miRNA might be responsible for the differential response of contrasting rice accessions towards As(III) and As(V) stress. Study of the proximal promoter sequences of the As-responsive miRNAs suggests that these identified miRNAs contain metal-responsive cis-acting motifs and other elicitor and hormonal related motifs. Our study suggests a miRNA-dependent regulatory mechanism during As species-specific stress in different rice accessions. Further analysis based on results obtained will be helpful in dissecting the molecular mechanism behind As responses in different rice accessions.
Introduction
Arsenic (As), a toxic metalloid, found naturally in the Earth’s crust is a global challenge to human beings and other life forms. It is a major concern in many South-East Asian developing countries, where the amount of As in ground water is present in more than the permissible level. The arsenic level in the ground water of Bangladesh and the West Bengal region of India exceeds 50 μg L−1; according to World Health Organization guidelines1 the recommended level is 10 μg L−1. The presence of As in drinking water and food is a serious concern to human health.2,3 Arsenic contaminated water used for irrigation of paddy fields causes high As levels in rice grains. Since rice is the staple crop growing in these areas, the population living in these areas as well as around the world is currently under threat due to As toxicity in their diets.4 Based on a plethora of reports, it is established that As containing crops, mainly rice, are the primary avenue of As exposure to people. Studies also revealed that As is equally harmful to plants and can limit uptake and accumulation of essential micro-nutrients and amino acids.5,6
Owing to the huge negative effect on human health, studies related to As uptake, accumulation, transport and detoxification in rice has gained momentum in the past few years with a major objective being to develop less As accumulating rice cultivars.7 With the application of high throughput techniques like transcriptome and proteome profiling, knowledge about the underlying molecular mechanisms of As metabolism in rice has enhanced.8–12 Arsenite (As(III)) and arsenate (As(V)) are predominant inorganic species of As in soil and depending upon the changing redox potential and pH, these two inorganic As species are readily interconvertible.13 As(V) is the most prevalent form of As in aerobic soils and is an analogue of inorganic phosphate. Due to this chemical similarity, uptake of As(V) is mediated through phosphate transporters into the plant cells.14–17 As(III), on the other hand, mainly predominates in anaerobic conditions such as flooded paddy soil and moves into the roots via nodulin 26-like intrinsic protein (NIP) aquaporin channels. NIP2 (Lsi1), which is known for its permeability to silicon, mediates the bidirectional transport of As(III) in rice.17 One of the established mechanisms of As detoxification in plants depends on complexation with cellular thiols followed by sequestration into vacuoles.19,26,27 Within the plant system, As(V) is reduced to As(III) which is ultimately sequestered into vacuoles after conjugation with glutathione (GSH) or phytochelatin (PC).10 The transcriptome modulation suggests that a number of genes involved in diverse physiological processes might also be playing an important role during As(III) and As(V) stress.6,9,12 Recently, a rice NRAMP (Natural Resistance-Associated Macrophage Protein) transporter, OsNRAMP1, has been reported to be involved in xylem loading and enhanced accumulation of As in Arabidopsis.18
Both the inorganic forms of As are highly toxic, one of which (As(III)) interacts and binds with sulfhydryl groups of proteins and therefore inhibits their functions. On the other hand, As(V) interferes with phosphate metabolism by inhibiting phosphorylation and ATP synthesis.3,19,20 Recent studies regarding a bacterial strain (GFAJ-1) showed that organisms can rely on As instead of phosphorus. These studies suggested that As(V) can replace phosphate in biomolecules that are essential to sustain cell life.21,22 It was suggested that, within a bacterium, As(V) interacts and replaces phosphate to form arsenylated analogs with small molecular weight metabolites such as NADH, ATP, glucose, and acetyl-CoA. It also substitutes phosphate at serine, tyrosine, and threonine residues to form arsenylated proteins.21,22 Apart from this, a series of recent studies report the substantial virtue of anti-cancerous activity of As(III) for curing acute promyelocytic leukemia patients.23–25 These studies also described and successfully dissected the molecular mechanism of the anti-cancerous property of As(III).
MicroRNAs (miRNAs) are established as a critical determinant of the growth and development of organisms.28–30 miRNAs comprise a major class of non-coding small RNA molecules which mediate silencing of their targets by either translation inhibition or mRNA cleavage in plants.28,31–34 In addition to regulating plant growth and development, miRNAs are known to regulate gene expressions in response to nutritional deprivation as well as biotic and abiotic stresses.35–39 Recently, the role of miRNAs has been investigated during metal stress.40–42 Using a miRNA microarray, cadmium (Cd) responsive miRNAs have been identified in rice36 and Brassica.43 Through transcriptome sequencing Yu et al. (2012)44 have identified As(III) responsive miRNAs in rice. In addition, miRNAs have been identified later through deep sequencing of a small RNA library in the root of a rice seedling under As(III) stress.45,46 However, despite having a huge impact on gene expressions, little is known about the proportion of miRNA mediated regulation of the regulatory network of As assimilation in rice. Most of these studies have been carried out on As(III) stress on specific rice germplasm. Studies suggests that transcriptomes of different rice germplasms are modulated differentially in response to As(III) and As(V) stress.9,12 Therefore, it seems that differential miRNA expression patterns might be playing an important role in modulating transcriptomes of rice germplasms in response to As(III) and As(V) stress.
The study of natural variations is often considered as an indispensable system nowadays in biology.47 Natural accessions of Arabidopsis are frequently used for understanding the mechanisms and pinpoint the causal genes underlying a particular allele.48 Similar to Arabidopsis, a large genetic variation of rice germplasm has been identified from the Indian subcontinent,49 and these accessions can serve as a model to identify mechanisms that enable them to tolerate various stresses. Screening of rice accessions commonly growing in the Indian subcontinent for As levels in grains established a range of cultivars with varying potential for As accumulation, including High As accumulating Rice Germplasm (HARG) and Low As accumulating Rice Germplasm (LARG).50,51 However, limited information about the role of miRNAs in different species of As stress in different rice germplasm is available. This study has been carried out to investigate the role of miRNA mediated gene regulation in different species of As (As(III) and As(V)) using contrasting As accumulating rice accessions (HARG and LARG). We have identified a set of miRNAs with differential expression and a possible role in As stress. Some of these miRNAs show germplasm as well as As-species specific expression and might be playing an important role in deciding the contrasting response of these germplasms. Our data provide more insights about the role of miRNA on a molecular basis of As(III) and As(V) stress in HARG and LARG.
Experimental
Plant growth conditions and treatments
Seeds of HARG (IC-115730) and LARG (IC-340072) rice (Oryza sativa L. indica) genotypes50 were utilized for the study. Seeds were surface sterilized in water containing 0.1% HgCl2 for 30 s, washed with sterile water and kept overnight in milli-Q water. Soaked seeds were transferred into Petri plates and incubated at 28 °C for 3–4 days for germination. Germinated rice seedlings were transferred in modified Hewitt medium52 and grown under controlled conditions; temperature (28 ± 2 °C), relative humidity (70%) and 16/8 h light/dark cycle for 10 days. Plants were subjected to As(III) [10 μM and 25 μM (stock solution 10 mM; NaAsO2, ICN, USA)] and As(V) [50 μM and 100 μM (stock solution 50 mM; Na2HAsO4, ICN, USA)] stress for seven days. All nutrient solutions were changed twice per week, and the pH was adjusted to 5.5 using 0.1 KOH or HCl. After that, seedlings were harvested, washed and stored immediately at −80 °C for further RNA extraction.
Microarray experiment and data analysis
Total RNA was isolated from root samples using a mirVana™ miRNA isolation kit (Ambion, USA) according to the manufacturer's instructions. The amount of RNA was measured with a Nanodrop ND-1000 Spectrophotometer (Thermo Scientific, USA). Presence of small RNA molecules was determined by denaturing polyacrylamide (15%) gel electrophoresis. RNA was labelled using FlashTag Biotin HSR RNA Labelling Kits (Genesphere, USA). Labelled RNA was hybridised at Affymetrix GeneChip miRNA-2.0 for 16 h. During hybridisation, washing and staining, Affymetrix GeneChip protocols (Affymetrix, USA) were strictly followed. The percentage of hybridization was analysed with the Affymetrix miRNA QC Tool. Normalization of the CEL files has been performed by using the R-bioconductor setting P ≤ 0.05.53
For construction of a heat map related to the expression of targets, the CEL file of our previous study that described the effect of As(V) on rice seedlings was taken into consideration.9 Affymetrix rice genome array probe IDs of targets were identified through searching of the Rice Multi-platform Microarray Search tool (http://www.ricearray.org/element/search_single.shtml).54 An expression map was generated using Dchip software.55
miRNA expression analysis
To validate the differential expression of mature miRNAs, a TaqMan MicroRNA assay kit (Applied Biosystems, Foster City, CA) was used for selected miRNAs identified through microarray analysis. TaqMan assays specifically detect mature and biologically active miRNA. Total RNA (0.2 μg) was used for a reverse transcription reaction using miRNA specific primers included in the TaqMan MicroRNA assay kit. Quantitative real-time polymerase chain reaction (qRT-PCR) was performed using the ABI 7500 instrument (Applied Biosystems, Foster City, CA, USA). In a total volume of 20 μL of reaction mixture, 1.33 μL of complementary DNA templates was mixed with 10 μL of TaqMan Universal Master Mix No AmpEras UNG (Applied Biosystems, Foster City, CA) along with 1 μL of TaqMan small RNA assays (20×). A standard TaqMan RT-PCR protocol with reaction conditions of 50 °C for 2 min and 95 °C for 10 min, followed by 40 cycles of 95 °C for 15 s and 60 °C for 60 s was followed. Ubiquitin (U6) was used as an endogenous control for data normalization. The relative fold change of the miRNAs in the treated samples compared to the controls was calculated using the 2−ΔΔCT method. Expression of each miRNA was analysed in triplicate in three independent experiments.
Target prediction of miRNAs and expression analysis
To predict the targets, the online miRNA target prediction tool psRNATarget (http://plantgrn.noble.org/psRNATarget/) was used by searching miRNAs in the cDNA OSA1 release 5 as reference genomes.56 Default parameters were set and a minimal weighed score of <3.0 was applied to sort the potential targets. Gene ontology annotation of the targets, especially the molecular function was assigned by using the AgriGO (http://bioinfo.cau.edu.cn/agriGO/) web-based analysis tool.57
To study expression of target genes, quantitative RT-PCR (qRT-PCR) analysis was carried out. Approximately, 2 μg RNase free DNase treated total RNA isolated from different rice samples was reverse-transcribed using SuperScriptII (Fermentas, USA), following the manufacturer's recommendation. The synthesized cDNA was diluted 1
:
20 in DEPC water and subjected to qRT-PCR analysis using SYBR Green Supermix (ABI Biosystems, USA) in an ABI 7500 instrument (ABI Biosystems, USA). Rice actin gene was used as an internal control to estimate the relative transcript level of the gene tested. The list of different genes and oligonucleotides used is provided in Table S1 (ESI†).
Motif analysis in promoters of miRNAs
The 2-kbp promoter sequences of all differentially expressed miRNAs were retrieved from the plant miRNA database (http://bioinformatics.cau.edu.cn/PMRD/).58 Sequences were individually searched for presence of cis-elements by using the PlantCARE tool (http://bioinformatics.psb.ugent.be/webtools/plantcare/).59 The distribution of individual motifs among all the promoters was manually investigated.
Statistical analysis
Each experiment was carried out under completely randomized design with three replicates repeated at least thrice. The data were analyzed by the Student's unpaired t-test, and the treatment mean values were compared at P ≤ 0.05–0.001.
Results and discussion
Inorganic arsenic species inhibit growth of HARG and LARG
Similar to the natural variation of Arabidopsis, rice accessions are also becoming potentially valuable in terms of studying various agronomically important traits and in revealing the biology of adaptive responses towards abiotic stresses. Due to the impact of As on the nutritional values of rice and its associated toxicity in human beings, an extensive screening of native accessions of rice grown in the Indian subcontinent for their inherent characteristics to accumulate varying levels of As in grains was performed.6,50,51 The investigation revealed that variation in As accumulation patterns existed in a diverse set of rice accessions. These accessions were categorised as High As accumulating Rice Germplasm (HARG) and Low As accumulating Rice Germplasm (LARG).6 In addition, As(III) tolerant and As(III) sensitive accessions were screened from 303 rice genotypes on exposure to As(III) (10 μM and 25 μM) in hydroponic media. As accumulation analysis suggested a contrast (13-fold difference) in As accumulation between HARG (IC-115730) and LARG (IC-340072) accessions. Studies also reported that a higher antioxidant potential and stress responsive amino acid level was seen in the case of HARG compared to LARG.50 The differences between HARG and LARG might be due to the presence of evolutionary genetic variations. In this study, we studied the growth of HARG (IC-115730) and LARG (IC-340072) accessions after exposure to two As inorganic species (As(III) and As(V)). Ten-day-old rice seedlings were grown in the presence of As(III) (10 and 25 μM) and As(V) (50 and 100 μM) for 7 days. A substantial reduction in overall growth of both the cultivars was noted in the presence of As(III) and As(V) (Fig. 1A and B). However, the observed phenotypic difference was not significant in both cultivars upon As(V) treatment (Fig. 1B). Also, it is observed that As exposure stimulates more lateral root formation in both the cultivars. This suggests a contrasting response of HARG and LARG accession towards the different species of As. Studies have already reported that out of both inorganic As-species, As(III) is more toxic in comparison to As(V) due to its effect on enzymes and proteins containing cysteine residues which leads to conformational distortion as well as preventing the disulphide bridge formation.60
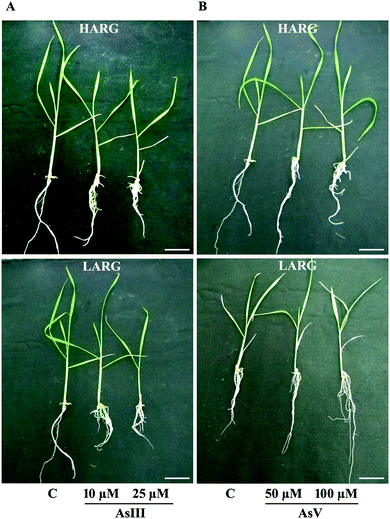 |
| Fig. 1 A representative picture showing the effect of As-stress on the morphology/phenotype of HARG and LARG rice cultivars. The cultivars, were germinated and allowed to grow for 5 days at 37 °C and then transferred to a Hewitt solution. After 10 days of growth, the seedlings were treated with different concentrations of As(III) (A) and As(V) (B) for 7 days under standard growth conditions. Photographs of representative seedlings of the control and two cultivars were taken 7 days post treatment. The white line in each panel indicates the scale bar (1 cm). | |
Modulated expression of miRNAs in HARG and LARG in response to arsenic
To study the possible involvement of miRNAs for the contrasting response of HARG and LARG towards As(III) and As(V) stress, global miRNA expression analysis was carried out. High throughput techniques like microarrays and small RNA library sequencing are frequently used for studying the involvement of miRNAs in the growth, development and towards stress response in organisms. Many stress related crucial miRNAs in Arabidopsis and Cd responsive miRNAs in rice were previously identified through a microarray platform.36,40 Here, we have utilized a microarray for studying the role of candidate miRNAs responsible for the differential As response of HARG and LARG. We performed a miRNA microarray using Gene Chip miRNA 2.0 arrays (Affymetrix) with RNA isolated from As(III) (25 μM) and As(V) (50 μM) treated rice roots. This array contains a complete set of miRNAs documented in miRBase release 15 that covers miRNA of 131 organisms including Arabidopsis thaliana, Brassica napus, Glycine max, Populus trichocarpa, Sorghum bicolor, Triticum aestivum and many others. The miRNAome of rice is represented by the presence of 496 individual miRNAs on the array.
Our analysis showed hybridization of a large number of miRNAs from rice in addition to other plants present on the array. This may be due to a high degree of sequence resemblance between the miRNAs present on the array used in the analysis.61 For detailed analysis, we have considered only those miRNAs which hybridized with probe sets of rice miRNAs to avoid any discrepancy. The analysis revealed that a set of miRNA from different families express differentially in both the cultivars in response to As(III) and As(V) stress. Analysis revealed that 114 members of 30 miRNA families were differentially expressed upon As(III) exposure and among these 24 miRNAs were up- and 90 were down-regulated respectively in HARG (Fig. 2A). Similarly, 166 members of 62 miRNA families were differentially expressed in LARG, out of which 5 and 161 members were up- and down-regulated respectively (Fig. 2A). It is interesting to note that the majority of miRNAs were down-regulated in both LARG and HARG. Of the identified set, only 5 miRNAs showed an As(III) induced expression in LARG whereas 24 miRNAs were up-regulated in HARG. This suggests that the modulated expression of miRNAs, in terms of the number of miRNA between HARG and LARG, could be responsible for their variable responses towards As(III) stress in these cultivars.
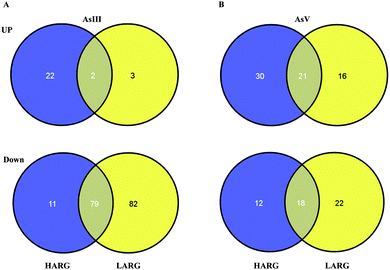 |
| Fig. 2 Venn diagram representing numbers of differentially expressed miRNAs induced by As-stress in LARG and HARG. (A) Up/down-regulated miRNAs identified with a 1-fold change in expression under As(III) stress. (B) Up/down-regulated miRNAs identified with a 1-fold change in expression under As(V) stress. | |
Our phenotypic studies suggest that in contrast to As(III), there is no significant change in the growth of HARG and LARG under As(V) stress (Fig. 1). This fact is also supported by many earlier studies which described As(V) as less toxic when compared to As(III).9,62 Our analyses clearly suggest that As(III) modulates the expression of a significant number of miRNAs in comparison to As(V) (Fig. 2). Of the total 81 miRNAs, 51 members were up- and 30 are down-regulated respectively in HARG (Fig. 2B). Likewise, 37 and 40 members were up- and down-regulated respectively in LARG (Fig. 2B). The analysis suggests that the expression of a large number of miRNAs is significantly modulated in either HARG or LARG under As(III) and As(V) stress. This might be one of the major reasons for the contrasting nature of cultivars towards As(III) and As(V) stress. Number of miRNAs with significantly modulated expression under As(III) and As(V) stress as well as in HARG and LARG are shown in Fig. S1 (ESI†).
Quantitative RT-PCR analysis of miRNAs in HARG and LARG
Our analysis identified a set of miRNAs with a modulated expression in response to As(III) and As(V) stress in rice. Some of these miRNAs showed a rice genotype-specific expression in response to specific As species. To further identify miRNAs with significant changes in the expression, the threshold value for the fold change expression was increased to 1.5 fold (P ≤ 0.05). Interestingly, 14 miRNAs distributed in various families were identified through this analysis and are listed in Table 1.
Table 1 Expression patterns of As(III) and As(V) stress induced miRNAs in HARG and LARG rice cultivarsa
S. no. |
miRNAs |
As(III) |
As(V) |
HARG |
LARG |
HARG |
LARG |
miRNAs with fold change >1.5 and P-value < 0.05.
|
1 |
miR164 |
Down |
Down |
Down |
Down |
2 |
miR171 |
Down |
Down |
Down |
Down |
3 |
miR395 |
Down |
Down |
Down |
Down |
4 |
miR399 |
Down |
Up |
Up |
Up |
5 |
miR396 |
Down |
Down |
Up |
Up |
6 |
miR408 |
Up |
Up |
Up |
Up |
7 |
miR528 |
Up |
Up |
Down |
Down |
8 |
miR529 |
Down |
Down |
Down |
Down |
9 |
miR820 |
Down |
Down |
Down |
Down |
10 |
miR1432 |
Down |
Down |
Down |
Down |
11 |
miR1846 |
Up |
Down |
Down |
Down |
12 |
miR1861 |
Up |
Up |
Up |
Up |
13 |
miR2102 |
Up |
Up |
Up |
Up |
14 |
miR2907 |
Up |
Up |
Up |
Up |
To validate the microarray data, qRT-PCR analysis of selected miRNAs from different gene families (miR164e, miR171g, miR395b, miR399h, miR528, miR529b, miR820a and miR1432) was performed. The analysis suggests that expressions of most of the miRNAs are in accordance to that of the microarray results. Similar to the microarray data, a contrast expression between HARG and LARG was observed for some of the miRNAs in the qRT-PCR results. A set of miRNA including miR171g, miR529b, miR820a and miR1432 was repressed in HARG whereas their expressions were significantly enhanced in LARG in response to As(III) stress (Fig. 3A). Surprisingly, the microarray expression pattern of a few miRNAs did not correlate with the qRT-PCR results. Examples of such miRNAs are miR820a and miR1432, whose expressions were down-regulated in the microarray in LARG, while in the qRT-PCR enhanced expression was observed under As(III) and As(V) stresses (Fig. 3). One possible explanation of such inconsistency may be due to degenerate probe sets for the miRNAs, which may have cross hybridised with the same miRNA of other species. However, Taqman probes used in qRT-PCR would have measured a miRNA level accurately.
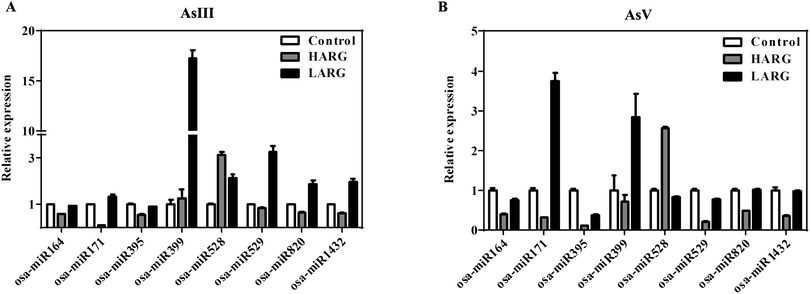 |
| Fig. 3 qRT-PCR expression analysis of selected differentially expressing miRNAs identified through microarray analysis. (A) Expression levels of eight miRNAs between HARG and LARG rice cultivars exposed to As(III) stress. (B) Expression levels of eight miRNAs between HARG and LARG rice cultivars exposed to As(V) stress. Data are reported as mean ± SE for three independent experiments. | |
A differential expression pattern was observed among members within each miRNA family. In the miR2907 family, miR2907a and miR2907d were significantly up-regulated whereas miR2907b and miR2907c were down-regulated in the LARG variety in response to As(III) stress. Similarly, miR162, miR166, miR396, miR529, miR1846 and miR1862 family members showed differential expression patterns (Table S2, ESI†). In a previous study, numerous As(III) responsive miRNAs including miR156, miR159, miR171, miR396, miR444, miR535, miR820, miR827 and miR1432 were identified in As(III) using the Nipponbare rice cultivar.18,44–46 In our study, we have identified a set of additional As-stress responsive miRNAs using different rice germplasm. These results suggested the possible involvement of these miRNAs for controlling As responses and accumulation in different rice cultivars.
Target prediction of rice miRNA
Various studies have suggested that miRNAs are highly specific for their targets and inhibit target expression post-transcriptionally either through target cleavage or via translation inhibition.18,63,64 To identify the potential targets of the As-responsive miRNAs identified in this study, we have employed a psRNATarget algorithm, a web-based program using default parameters.56 miRNAs which showed significantly modulated expression profiles under As(III) and As(V) stress in LARG and HARG were chosen for target identification. Interestingly, most of the predicted targets identified belong to DNA binding proteins, transcription factors such as NAC domain-containing protein, nuclear transcription factor Y, growth-regulating factor 1, and AP2, MADS-box, F-box, MYB and SPB families. Most of these transcription factors have been shown to play an important role in plant development and during abiotic stresses.26,35,65–67 Modulated expression of these transcription factors has been shown in several earlier studies,9,12 and it was speculated that these transcription factors might play a prominent role in determining the physiological response like As tolerance/sensitivity in rice.9 Numerous loci that encoded the metal transport protein, such as the ATP-binding protein, protein kinases and methyltransferases were also predicted among as putative targets of identified miRNAs in this study (Table 2). Importantly, the genes involved in the sulphur metabolism such as the low affinity sulphate transporter, bifunctional 3-phosphoadenosine 5-phosphosulphate synthetase and ATP sulfurylases are identified as the targets of miRNAs with modulated expression. These genes act as sensors for sulphur limitation and constitute an integral part of the regulatory network of sulphate assimilation in plants.68–70 A set of genes that maintains redox homeostasis of the cell is another promising category in the target list (Table 2). These include a L-ascorbate oxidase precursor, copper ion binding protein, superoxide dismutase, ZIP zinc/iron transport family protein and blue copper protein precursor.
Table 2 Putative targets of As-responsive miRNAs
miRNA accession |
Target accession |
Target start |
Target end |
Annotation |
osa-miR164 |
Os06g23650 |
794 |
814 |
CUC2, No apical meristem (NAM) protein |
Os06g46270 |
954 |
974 |
NAC domain-containing protein |
Os12g41680 |
911 |
931 |
NAC domain-containing protein |
Os03g47310 |
43 |
63 |
Transposon protein |
Os05g25960 |
368 |
388 |
No apical meristem protein |
Os04g41540 |
1060 |
1080 |
Calmodulin |
|
osa-miR171 |
Os02g44360 |
1351 |
1371 |
SCARECROW gene regulator |
Os06g01620 |
456 |
476 |
Scarecrow-like 6 |
Os02g19990 |
1598 |
1617 |
Reticulon family protein |
Os07g01020 |
1190 |
1209 |
Pyridoxin biosynthesis protein ER1 |
Os01g65720 |
1069 |
1089 |
Glycosyl hydrolase |
Os03g19070 |
1043 |
1063 |
Long cell-linked locus protein |
Os08g31560 |
200 |
220 |
WD-repeat protein-like |
Os05g21120 |
3128 |
3147 |
Retrotransposon protein |
Os07g33630 |
2566 |
2586 |
Microtubule-associated protein TORTIFOLIA1 |
|
osa-miR395 |
Os03g09940 |
318 |
338 |
Low affinity sulphate transporter 3 |
Os03g53230 |
595 |
615 |
Bifunctional 3-phosphoadenosine 5-phosphosulfate synthetase |
Os03g09930 |
111 |
131 |
Sulfate transporter 2.1 |
Os03g19750 |
832 |
851 |
Transposon protein |
Os06g46480 |
1105 |
1124 |
Expressed protein |
Os11g44580 |
1171 |
1190 |
Disease resistance RPP13-like protein 1 |
|
osa-miR396 |
Os06g10310 |
282 |
302 |
Growth-regulating factor 1 |
Os06g02560 |
605 |
625 |
Growth-regulating factor |
Os04g51190 |
535 |
555 |
Growth-regulating factor |
Os02g47280 |
570 |
590 |
Growth-regulating factor |
Os06g02560 |
613 |
633 |
Growth-regulating factor |
Os02g47280 |
667 |
687 |
Growth-regulating factor |
Os02g53690 |
570 |
590 |
atGRF5 |
Os03g47140 |
947 |
967 |
atGRF2 |
Os04g24190 |
828 |
847 |
Growth-regulating factor 1 |
Os04g48510 |
858 |
878 |
Transcription activator |
Os03g51970 |
419 |
439 |
Growth-regulating factor 1 |
Os12g29980 |
732 |
752 |
atGRF2 |
Os01g25330 |
1038 |
1058 |
SAC9 |
Os11g35030 |
870 |
889 |
Expressed protein |
Os11g35030 |
1557 |
1576 |
Expressed protein |
Os12g29980 |
727 |
747 |
atGRF2 |
Os02g58214 |
69 |
92 |
Expressed protein |
Os01g27430 |
1352 |
1372 |
Retrotransposon protein |
Os09g26000 |
1623 |
1645 |
Glutamate receptor 2.8 precursor |
Os09g25960 |
1731 |
1753 |
Glutamate receptor 2.7 precursor |
Os09g25990 |
1725 |
1747 |
Glutamate receptor 2.6 precursor |
Os11g43410 |
2850 |
2870 |
NB-ARC domain containing protein |
|
osa-miR399 |
Os05g48390 |
904 |
924 |
Ubiquitin conjugating enzyme |
Os05g45350 |
1110 |
1130 |
Electron transporter/heat shock protein binding protein |
Os09g33830 |
1271 |
1291 |
Solute carrier family 35, member F1 |
Os03g13800 |
820 |
839 |
NHP2-like protein 1 |
Os09g38330 |
2144 |
2163 |
Expressed protein |
Os06g19660 |
1785 |
1804 |
Nucleotide binding protein |
Os04g33860 |
495 |
514 |
Expressed protein |
|
osa-miR408 |
Os11g01470 |
1333 |
1353 |
Retrotransposon protein |
Os03g05650 |
644 |
664 |
Expressed protein |
Os02g43660 |
679 |
699 |
Blue copper protein precursor |
Os10g41040 |
362 |
382 |
Expressed protein |
Os08g37670 |
657 |
677 |
Blue copper protein precursor |
Os07g43540 |
184 |
204 |
Origin recognition complex subunit 6 |
Os01g53880 |
1357 |
1376 |
OsIAA6 – Auxin-responsive Aux/IAA gene family member |
Os05g25120 |
79 |
98 |
Hypothetical protein |
Os07g29660 |
66 |
86 |
Hypothetical protein |
Os02g06250 |
98 |
118 |
Phytosulfokine receptor precursor |
Os06g19130 |
66 |
86 |
Conserved hypothetical protein |
Os09g21520 |
1514 |
1534 |
ATP binding protein |
Os09g08030 |
231 |
250 |
Seven-transmembrane-domain protein 1 |
Os09g08440 |
405 |
424 |
mtN3/saliva family protein |
|
osa-miR528 |
Os08g04310 |
247 |
267 |
Uclacyanin-2 precursor |
Os06g06050 |
2648 |
2668 |
F-box/LRR-repeat MAX2 |
Os01g40150 |
2510 |
2530 |
Translation initiation factor IF-2 |
Os10g24090 |
577 |
597 |
Expressed protein |
Os06g37150 |
1998 |
2018 |
L-Ascorbate oxidase precursor |
Os07g38290 |
528 |
547 |
Copper ion binding protein |
Os01g44330 |
41 |
61 |
L-Ascorbate oxidase precursor |
Os09g33800 |
371 |
391 |
Arabinogalactan protein |
Os08g44770 |
299 |
319 |
Superoxide dismutase, chloroplast precursor |
Os01g03620 |
59 |
79 |
Copper ion binding protein |
Os01g03640 |
45 |
65 |
Copper ion binding protein |
|
osa-miR529 |
Os09g31438 |
805 |
824 |
Squamosa promoter-binding-like protein 9 |
Os01g69830 |
1149 |
1168 |
Teosinte glume architecture 1 |
Os04g51830 |
1646 |
1666 |
Cation transporter HKT7 |
Os09g32944 |
1030 |
1049 |
Teosinte glume architecture 1 |
Os08g41940 |
1050 |
1069 |
Teosinte glume architecture 1 |
Os08g34820 |
482 |
501 |
F-box domain containing protein |
Os09g07300 |
11114 |
11134 |
BIG |
Os05g04150 |
149 |
169 |
Expressed protein |
Os05g14010 |
80 |
99 |
Expressed protein |
Os12g08760 |
82 |
102 |
Carboxyvinyl-carboxyphosphonate phosphorylmutase |
Os01g65780 |
66 |
86 |
Secondary cell wall-related glycosyltransferase family 8 |
Os02g07720 |
1136 |
1155 |
Choline-phosphate cytidylyltransferase B |
Os05g07090 |
1587 |
1606 |
Glutaryl-CoA dehydrogenase, mitochondrial precursor |
Os03g57900 |
576 |
596 |
Zinc finger A20 and AN1 domains-containing protein |
Os02g03620 |
40 |
59 |
Ubiquitin ligase SINAT2 |
Os04g28420 |
1846 |
1865 |
Peptidyl-prolyl isomerase |
|
osa-miR820 |
Os03g02010 |
304 |
324 |
DNA cytosine methyltransferase Zmet3 |
Os11g13650 |
93 |
113 |
ATCSLC12 |
Os11g03310 |
129 |
149 |
NAC domain-containing protein |
Os10g42196 |
4014 |
4033 |
Expressed protein |
|
osa-miR1432 |
Os03g59790 |
45 |
65 |
Calcium-binding protein |
Os03g59770 |
117 |
137 |
Calcium-binding allergen Ole e 8 |
Os04g35590 |
485 |
505 |
Thioesterase superfamily member |
Os05g07210 |
1166 |
1186 |
ZIP zinc/iron transport family protein |
Os08g36910 |
587 |
606 |
Alpha-amylase isozyme 3D precursor |
|
osa-miR1846 |
Os08g10350 |
477 |
497 |
Expressed protein |
Os02g18870 |
76 |
95 |
Anther-specific proline-rich protein APG |
Os01g70100 |
668 |
687 |
Palmitoyltransferase ZDHHC9 |
|
osa-miR1861 |
Os01g63810 |
610 |
629 |
Starch binding domain containing protein |
Os05g51790 |
578 |
599 |
ATP binding protein |
Os09g09500 |
1805 |
1824 |
Lectin-like receptor kinase 7 |
Os11g08950 |
1213 |
1232 |
ATP binding protein |
Os01g03090 |
740 |
759 |
SLL2 |
|
osa-miR2102 |
Os08g22780 |
110 |
129 |
Retrotransposon protein |
Os01g12280 |
145 |
164 |
Protein dimerization |
Os03g32610 |
763 |
782 |
hypothetical protein |
Os07g42834 |
1539 |
1558 |
Retrotransposon protein |
|
osa-miR2907 |
Os05g18660 |
460 |
481 |
F-box domain containing protein |
Os10g12130 |
987 |
1008 |
Retrotransposon protein |
Gene ontology classification of targets of significantly differentially regulated miRNA targets (≥1.5-fold) in both the varieties under As(III) and As(V) stress was also performed by using the AgriGO online available tool.57 The molecular characterizations of target loci indicated that a major proportion of the target genes belongs to transcription factors followed by DNA binding proteins and genes involved in catalytic activity (Fig. 4).
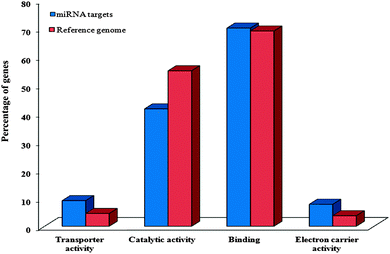 |
| Fig. 4 GO analyses of the targets of the 14 As-stress responsive miRNAs in rice. The blue bars indicate the enrichment of the GO terms in the miRNA targets in GO. The red bars indicate the percentage of reference genes present in different GO in rice. | |
Targets are negatively related with miRNA expressions
In our analysis, a large number of target loci of each miRNA were predicted by psRNATarget. In order to investigate the actual targets among putative targets identified by in silico analysis, we further analysed our results. First, we examined the transcript abundance of putative targets in the microarray analysis carried out in our previous study related to the transcriptome modulation during As(V) stress in rice seedlings.9 It was observed that the majority of targets of up-regulated miRNAs such as miR399, miR408, miR528, miR1861 and miR2907 were down-regulated in the analysis (Fig 5A). Similarly, the expression of targets of down-regulated miRNAs such as miR164, miR171, miR395, miR529, miR820, miR1432 and miR1846 were induced during As(V) stress in rice (Fig 5C). This suggests a direct correlation between expression of identified miRNAs in this study and expression of targets during As(V) stress.
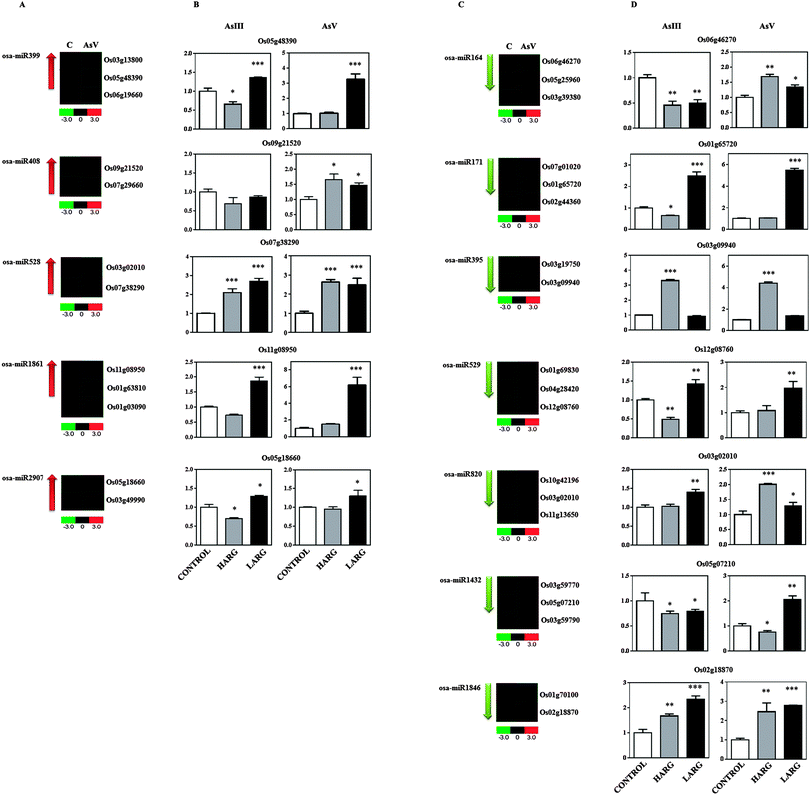 |
| Fig. 5 Expression profiles of As-induced miRNAs and their putative targets in HARG and LARG rice cultivars. (A) and (C) represent expression patterns of up- and down-regulated As-responsive miRNAs and their targets respectively. Arrows represent enhanced (A) and reduced (C) expression of miRNAs in As exposed rice seedlings. The heat map shown represents the expression profiles of putative target genes in As(V) stress. Expression data for As(V) stress treatment were used for the analysis.9 The color scale for fold change values is shown at the bottom. (B) and (D) represent qRT-PCR analysis of target genes of up- and down-regulated miRNAs in HARG and LARG in response to As(III) and As(V) stress respectively. | |
Second, to validate the expression, qRT-PCR analyses of the putative targets of As-responsive miRNAs in the root of HARG and LARG was carried out. It has been demonstrated that miR164 targets no apical meristem (NAM) protein, NAC-like transcription factors and Cup-shaped Cotyledon (CUC) of which CUC1 and CUC2 are necessary for the formation of boundaries between meristems, flower development and proper control of organ number in Arabidopsis.65–67 Some NAC proteins, which are a putative target of miR164, for instance NAC1, participate in transducing auxin signals for the development lateral roots.71 Emergence of more lateral roots under As stressed condition (Fig. 1) might be due to modulated transcripts of NAC. miR171 is known to target scarecrow-like regulators which participated in signal transductions, root development and plant development.72,73 Our data clearly revealed an inverse relation between expression of miR171 and its predicted target Os06g01620 which encodes a scarecrow-like 6 protein (Fig. 5C). miR395 regulates the expression of low-affinity sulphate transporter (SULTR2;1) and ATP sulfurylases involved in sulphate metabolism.69 miR395 is strongly induced upon sulphate starved conditions and controls sulphur uptake required during plant growth and development as well as in stress conditions.68,74 It has been demonstrated that the demand of sulphur generally increases during As stress due to enhanced biosynthesis of sulphur containing amino acids and peptides required for As detoxification.75–78 The down-regulation of miR395 in HARG and LARG and thereby up-regulation of sulphate transporter (Os03g09940) supports involvement of regulated sulphur homeostasis during As stress. Importantly, significantly higher expression of Os03g09940 in HARG is in accordance with its higher As accumulation phenotype. This higher As uptake may require high cellular thiols and sulphur for detoxification regulated by miR395.
The expression of miR399 was induced in both cultivars as evident from microarray and RT-PCR results (Fig. 3 and 5A). The inorganic phosphate starvation induces miR399 that represses E2 conjugase and regulate phosphate assimilation in plants.79 Due to structural analogy, As(V) shares phosphate transporters for the uptake and translocation.17,80 In this study, a substantial high expression (nearly 15 fold) of miR399 in LARG was observed in As(III) and upto 3 fold in As(V) stress compared to HARG. However, expression of one of the putative targets, ubiquitin conjugating enzyme, was not much repressed in HARG compared to LARG (Fig. 5B). The reason of miR399 induction upon As stress might be due to phosphate limitation because of competition with As(V). Another interesting observation in this study, is the difference in expression of miR528 under As(III) and As(V) exposure. The miR528 was induced upon As(III) stress whereas down-regulated upon As(V) stress and simultaneously their target expression was also modulated (Table 1, Fig. 5A and B). In general, microarray expression of miR408, miR529, miR820, miR1432, miR1846, miR1861 and miR2907, and their corresponding targets showed an inverse relation. Nonetheless, some inconsistency between expression patterns of the target through a microarray dataset and qRT-PCR was observed which might be due to the differential As accumulating rice cultivars used in this study. These observations suggested that modulated expression of these miRNAs could be one of the prime reasons for less As accumulation in the phenotype of LARG.
Metal responsive cis-elements in promoters of miRNAs
Binding of trans-acting factors on cis-elements of the promoter region of any gene and resulted changes in a RNA polymerase complex are thought to be one of the critical determinants of gene expression. In the past, the interaction of cis-elements with trans-factors such as stress induced DNA binding proteins has been identified through extensive research.81,82 In this study, expression of several miRNAs from diverse families was modulated during As stress. It is important to study whether specific cis-elements and interacting trans-factors control their As-responsive expression. To study this, the 2-kbp up-stream region of promoters of As-responsive miRNAs was analysed through the PlantCare database.59 Analysis revealed the presence of numerous conserved motifs in these promoters. Some of the conserved motifs include ARE elements (anaerobic induction), abscisic acid responsive elements such as ABRE, motif IIb and CE3, BOX-W1 (response to fungal elicitors), GC-motif (anoxic inducibility), TC-rich repeat for defence and stress response, LTR elements for low temperature, MYB and CCAAT-box elements for MYB binding site involved in drought inducibility, and heat stress-responsive elements (HSE) amongst many promoters. Besides these, potential cis-acting elements responsive to TCA (salicylic acid responsive), ERE (ethylene responsive) GARE, TATC and P-box (gibberellins responsive) and methyl jasmonate (MeJA) were also identified in the analysed promoters (Table S3, ESI†).
The presence of metal recognition elements (MREs; 5′-TGCGCNC-3′) in almost every promoter of miRNA genes with a modulated expression in response to As stress is an interesting observation in this analysis. A MRE-like sequence is a highly conserved motif commonly present in the promoters of metallothioneins in animals.83 Various trans-acting regulatory factors interact with this motif and modulate expression in response to metal stress. The MRE motif was evenly distributed in promoters of 14 studied miRNA families (Table S4, ESI†). The presence of MREs indicates the existence of a fine tuned mechanism by which expressions of miRNAs are under the control of trans-acting stress induced factors. Curiously, the Skn-1_motif was present in almost every promoter of miRNAs with modulated expression during As stress. This motif is required for endosperm specific expression, and function in cooperation with other motifs. Again, the presence of a methyl jasmonate responsive element (CGTCA and TGACG motif), abscisic acid responsive ABRE element, salicylic acid responsive TCA- element, gibberellin-responsive GARE-element and defence responsive TC-rich repeats clearly indicate that stress induced trans-factors/proteins triggers transcriptional activation of these miRNAs. Taken together, cis-element analysis of promoters suggested that most of the miRNAs identified in this study are stress responsive.
miRNAs expression and HARG and LARG phenotype
The presence of As(III) and As(V) led to the significant modulation in miRNAs expression profile in HARG and LARG as listed in Table S2 (ESI†). Most of the identified miRNAs are conserved across plant species and are known to regulate growth and development as well as stress responses in plants. This suggests that As(III) or As(V) are not solely responsible for induction of these miRNAs and As response may be linked to various other biotic, abiotic and environmental cues. Studies suggest that miR171, miR396, miR395, miR408 and miR529 are responsive to salt, cold, mannitol and drought stress in rice.40,42,84 However, a contrast expression profile of a set of miRNAs and their targets between HARG and LARG suggests that their contrasting As accumulating potentials may be regulated by miRNAs. miRNAs are known to work downstream to trans-acting proteins such as SA, JA, stress responsive and signal cascading factors. The cis-elements, present in the proximal promoters of various miRNAs with modulated expression in response to As stress suggests that regulations of these miRNAs are not only controlled with the SA and JA pathway, but also by GA, ethylene, ABA and other factors. Hence, an assessment of such factors including epigenetic changes which are known to regulate expression of miRNAs in LARG and HARG rice accessions will be required to dissect the molecular basis of an adaptive trait.
Conclusions
Arsenic, a highly toxic metalloid, is present in low amounts in the environment and is a dreadful health hazard to millions of people across the globe. Inorganic forms of As, As(III) and As(V), present in the soil accumulate in plant parts and contaminate the food chain. There is an urgent need to develop strategies to restrict As accumulation in plants, however, this requires understanding of the molecular mechanisms involved in As uptake, accumulation and detoxification. Previous studies based on microarray and deep sequencing have identified numerous differentially expressed genes in response to As(III) and As(V) in rice. In addition, several As(III)-responsive miRNAs have been identified in rice. Since uptake and transport mechanisms of these As species differ, it is important to investigate the involvement of As(III)- and As(V)-responsive miRNAs and their targets. In this study, microarray hybridization was carried out to identify differentially expressed miRNAs in response to As(III) and As(V) using two rice cultivars contrasting in As response and accumulation. Though, a number of miRNAs were differentially expressed in an As species- and cultivar-specific manner, expression of 14 miRNAs was modulated in response to As(III) and As(V) in both the cultivars. Among these miRNAs, members of the miR396, miR399, miR408, miR528, miR1861, miR2102 and miR2907 families were significantly up-regulated whereas members of the miR164, miR171, miR395, miR529, miR820, miR1432 and miR1846 families were down-regulated. Predicted targets for identified As-responsive miRNAs and study of cis-regulatory elements supported our findings regarding the involvement of these miRNAs in As-stress. Altogether, our data indicate that differentially expressed miRNAs in HARG and LARG may encompass a critical sector of molecular responses for the As-stress adaptation in rice cultivars.
Acknowledgements
This work was supported by research grants from the Department of Biotechnology, Government of India, New Delhi and Council of Scientific and Industrial Research (CSIR), New Delhi, as Network Project (BSC-0107). DS and MT thankfully acknowledge the Council of Scientific and Industrial Research (CSIR), New Delhi, India and Indian Council of Medical Research (ICMR), India for Senior Research Fellowship.
References
-
WHO, Arsenic and arsenic compounds, Environmental Health Criteria, World Health Organization, Geneva, 2001, p. 224 Search PubMed.
- D. Halder, A. Biswas, Z. Slejkovec, D. Chatterjee, J. Nriagu, G. Jacks and P. Bhattacharya, Arsenic species in raw and cooked rice: Implications for human health in rural Bengal, Sci. Total Environ., 2014, 497-498, 200–208 CrossRef CAS PubMed.
- F. J. Zhao, S. P. McGrath and A. A. Meharg, Arsenic as a food chain contaminant: mechanisms of plant uptake and metabolism and mitigation strategies, Annu. Rev. Plant Biol., 2010, 61, 535–559 CrossRef CAS PubMed.
- M. A. Rahman and H. Hasegawa, High levels of inorganic arsenic in rice in areas where arsenic-contaminated water is used for irrigation and cooking, Sci. Total Environ., 2011, 409, 4645–4655 CrossRef CAS PubMed.
- G. Duan, W. Liu, X. Chen, Y. Hu and Y. Zhu, Association of arsenic with nutrient elements in rice plants, Metallomics, 2013, 5, 784–792 RSC.
- S. Dwivedi, R. D. Tripathi, S. Srivastava, R. Singh, A. Kumar, P. Tripathi, R. Dave, U. N. Rai, D. Chakrabarty, P. K. Trivedi, R. Tuli, B. Adhikari and M. K. Bag, Arsenic affects mineral nutrients in grains of various Indian rice (Oryza sativa L.) genotypes grown on arsenic-contaminated soils of West Bengal, Protoplasma, 2010, 245, 113–124 CrossRef CAS PubMed.
- P. Tripathi, A. Mishra, S. Dwivedi, D. Chakrabarty, P. K. Trivedi, R. P. Singh and R. D. Tripathi, Differential response of oxidative stress and thiol metabolism in contrasting rice genotypes for arsenic tolerance, Ecotoxicol. Environ. Saf., 2012, 79, 189–198 CrossRef CAS PubMed.
- N. Ahsan, D. G. Lee, K. H. Kim, I. Alam, S. H. Lee, K. W. Lee, H. Lee and B. H. Lee, Analysis of arsenic stress-induced differentially expressed proteins in rice leaves by two-dimensional gel electrophoresis coupled with mass spectrometry, Chemosphere, 2010, 78, 224–231 CrossRef CAS PubMed.
- D. Chakrabarty, P. K. Trivedi, P. Misra, M. Tiwari, M. Shri, D. Shukla, S. Kumar, A. Rai, A. Pandey, D. Nigam, R. D. Tripathi and R. Tuli, Comparative transcriptome analysis of arsenate and arsenite stresses in rice seedlings, Chemosphere, 2009, 74, 688–702 CrossRef CAS PubMed.
- S. Kumar, R. S. Dubey, R. D. Tripathi, D. Chakrabarty and P. K. Trivedi, Omics and biotechnology of arsenic stress and detoxification in plants: current update and prospective, Environ. Int., 2015, 74, 221–230 CrossRef CAS PubMed.
- Y. Liu, M. Li, C. Han, F. Wu, B. Tu and P. Yang, Comparative proteomic analysis of rice shoots exposed to high arsenate, J. Integr. Plant Biol., 2013, 55, 965–978 CAS.
- G. J. Norton, G. Duan, T. Dasgupta, M. R. Islam, M. Lei, Y. Zhu, C. M. Deacon, A. C. Moran, S. Islam, F. J. Zhao, J. L. Stroud, S. P. McGrath, J. Feldmann, A. H. Price and A. A. Meharg, Environmental and genetic control of arsenic accumulation and speciation in rice grain: comparing a range of common cultivars grown in contaminated sites across Bangladesh, China, and India, Environ. Sci. Technol., 2009, 43, 8381–8386 CrossRef CAS PubMed.
- Z. Wu, H. Ren, S. P. McGrath, P. Wu and F. J. Zhao, Investigating the contribution of the phosphate transport pathway to arsenic accumulation in rice, Plant Physiol., 2011, 157, 498–508 CrossRef CAS PubMed.
- M. J. Abedin, M. S. Cresser, A. A. Meharg, J. Feldmann and J. Cotter-Howells, Arsenic accumulation and metabolism in rice (Oryza sativa L.), Environ. Sci. Technol., 2002, 36, 962–968 CrossRef CAS.
- G. P. Bienert, M. Thorsen, M. D. Schussler, H. R. Nilsson, A. Wagner, M. J. Tamas and T. P. Jahn, A subgroup of plant aquaporins facilitate the bi-directional diffusion of As(OH)3 and Sb(OH)3 across membranes, BMC Biol., 2008, 6, 26 CrossRef PubMed.
- E. Gonzalez, R. Solano, V. Rubio, A. Leyva and J. Paz-Ares, Phosphate transporter traffic facilitator1 is a plant-specific SEC12-related protein that enables the endoplasmic reticulum exit of a high-affinity phosphate transporter in Arabidopsis, Plant Cell, 2005, 17, 3500–3512 CrossRef CAS PubMed.
- J. F. Ma, N. Yamaji, N. Mitani, X. Y. Xu, Y. H. Su, S. P. McGrath and F. J. Zhao, Transporters of arsenite in rice and their role in arsenic accumulation in rice grain, Proc. Natl. Acad. Sci. U. S. A., 2008, 105, 9931–9935 CrossRef CAS PubMed.
- M. Tiwari, D. Sharma, S. Dwivedi, M. Singh, R. D. Tripathi and P. K. Trivedi, Expression in Arabidopsis and cellular localization reveal involvement of rice NRAMP, OsNRAMP1, in arsenic transport and tolerance, Plant, Cell Environ., 2014, 37, 140–152 CrossRef CAS PubMed.
- R. D. Tripathi, S. Srivastava, S. Mishra, N. Singh, R. Tuli, D. K. Gupta and F. J. Maathuis, Arsenic hazards: strategies for tolerance and remediation by plants, Trends Biotechnol., 2007, 25, 158–165 CrossRef CAS PubMed.
- R. D. Tripathi, P. Tripathi, S. Dwivedi, S. Dubey, S. Chatterjee, D. Chakrabarty and P. K. Trivedi, Arsenomics: omics of arsenic metabolism in plants, Front. Physiol., 2012, 3, 275 CAS.
- F. Wolfe-Simon, J. Switzer Blum, T. R. Kulp, G. W. Gordon, S. E. Hoeft, J. Pett-Ridge, J. F. Stolz, S. M. Webb, P. K. Weber, P. C. Davies, A. D. Anbar and R. S. Oremland, A bacterium that can grow by using arsenic instead of phosphorus, Science, 2012, 332, 1163–1166 CrossRef PubMed.
- Y. Xu, B. Ma and R. Nussinov, Structural and functional consequences of phosphate-arsenate substitutions in selected nucleotides: DNA, RNA, and ATP, J. Phys. Chem. B, 2012, 116, 4801–4811 CrossRef CAS PubMed.
- J. Hu, Y. F. Liu, C. F. Wu, F. Xu, Z. X. Shen, Y. M. Zhu, J. M. Li, W. Tang, W. L. Zhao, W. Wu, H. P. Sun, Q. S. Chen, B. Chen, G. B. Zhou, A. Zelent, S. Waxman, Z. Y. Wang, S. J. Chen and Z. Chen, Long-term efficacy and safety of all-trans retinoic acid/arsenic trioxide-based therapy in newly diagnosed acute promyelocytic leukemia, Proc. Natl. Acad. Sci. U. S. A., 2009, 106, 3342–3347 CrossRef CAS PubMed.
- R. Nasr, M. C. Guillemin, O. Ferhi, H. Soilihi, L. Peres, C. Berthier, P. Rousselot, M. Robledo-Sarmiento, V. Lallemand-Breitenbach, B. Gourmel, D. Vitoux, P. P. Pandolfi, C. Rochette-Egly, J. Zhu and H. de The, Eradication of acute promyelocytic leukemia-initiating cells through PML-RARA degradation, Nat. Med., 2008, 14, 1333–1342 CrossRef CAS PubMed.
- X. W. Zhang, X. J. Yan, Z. R. Zhou, F. F. Yang, Z. Y. Wu, H. B. Sun, W. X. Liang, A. X. Song, V. Lallemand-Breitenbach, M. Jeanne, Q. Y. Zhang, H. Y. Yang, Q. H. Huang, G. B. Zhou, J. H. Tong, Y. Zhang, J. H. Wu, H. Y. Hu, H. de The, S. J. Chen and Z. Chen, Arsenic trioxide controls
the fate of the PML-RARalpha oncoprotein by directly binding PML, Science, 2010, 328, 240–243 CrossRef CAS PubMed.
- A. Raab, H. R. Hansen, L. Zhuang and J. Feldmann, Arsenic accumulation and speciation analysis in wool from sheep exposed to arsenosugars, Talanta, 2002, 58, 67–76 CrossRef CAS.
- W. Y. Song, J. Park, D. G. Mendoza-Cozatl, M. Suter-Grotemeyer, D. Shim, S. Hortensteiner, M. Geisler, B. Weder, P. A. Rea, D. Rentsch, J. I. Schroeder, Y. Lee and E. Martinoia, Arsenic tolerance in Arabidopsis is mediated by two ABCC-type phytochelatin transporters, Proc. Natl. Acad. Sci. U. S. A., 2010, 107, 21187–21192 CrossRef CAS PubMed.
- D. P. Bartel, MicroRNAs: target recognition and regulatory functions, Cell, 2009, 136, 215–233 CrossRef CAS PubMed.
- J. C. Carrington and V. Ambros, Role of microRNAs in plant and animal development, Science, 2003, 301, 336–338 CrossRef CAS PubMed.
- M. W. Jones-Rhoades, D. P. Bartel and B. Bartel, MicroRNAS and their regulatory roles in plants, Annu. Rev. Plant Biol., 2006, 57, 19–53 CrossRef CAS PubMed.
- D. P. Bartel, MicroRNAs: genomics, biogenesis, mechanism, and function, Cell, 2004, 116, 281–297 CrossRef CAS.
- R. W. Carthew and E. J. Sontheimer, Origins and Mechanisms of miRNAs and siRNAs, Cell, 2009, 136, 642–655 CrossRef CAS PubMed.
- C. Poulsen, H. Vaucheret and P. Brodersen, Lessons on RNA silencing mechanisms in plants from eukaryotic argonaute structures, Plant Cell, 2013, 25, 22–37 CrossRef CAS PubMed.
- M. Tiwari, D. Sharma and P. K. Trivedi, Artificial microRNA mediated gene silencing in plants: progress and perspectives, Plant Mol. Biol., 2014, 86, 1–18 CrossRef CAS PubMed.
- J. C. de Lima, G. Loss-Morais and R. Margis, MicroRNAs play critical roles during plant development and in response to abiotic stresses, Genet. Mol. Biol., 2012, 35, 1069–1077 CrossRef CAS PubMed.
- Y. Ding, Z. Chen and C. Zhu, Microarray-based analysis of cadmium-responsive microRNAs in rice (Oryza sativa), J. Exp. Bot., 2011, 62, 3563–3573 CrossRef CAS PubMed.
- B. Khraiwesh, J. K. Zhu and J. Zhu, Role of miRNAs and siRNAs in biotic and abiotic stress responses of plants, Biochim. Biophys. Acta, 2012, 1819, 137–148 CrossRef CAS PubMed.
- C. Lelandais-Briere, C. Sorin, M. Declerck, A. Benslimane, M. Crespi and C. Hartmann, Small RNA diversity in plants and its impact in development, Curr. Genomics, 2010, 11, 14–23 CrossRef CAS PubMed.
- M. Zhao, H. Ding, J. K. Zhu, F. Zhang and W. X. Li, Involvement of miR169 in the nitrogen-starvation responses in Arabidopsis, New Phytol., 2011, 190, 906–915 CrossRef CAS PubMed.
- H. H. Liu, X. Tian, Y. J. Li, C. A. Wu and C. C. Zheng, Microarray-based analysis of stress-regulated microRNAs in Arabidopsis thaliana, RNA, 2008, 14, 836–843 CrossRef CAS PubMed.
- A. B. Mendoza-Soto, F. Sanchez and G. Hernandez, MicroRNAs as regulators in plant metal toxicity response, Front. Plant Sci., 2012, 3, 105 CAS.
- R. Sunkar and J. K. Zhu, Novel and stress-regulated microRNAs and other small RNAs from Arabidopsis, Plant Cell, 2004, 16, 2001–2019 CrossRef CAS PubMed.
- Z. S. Zhou, J. B. Song and Z. M. Yang, Genome-wide identification of Brassica napus microRNAs and their targets in response to cadmium, J. Exp. Bot., 2012, 63, 4597–4613 CrossRef CAS PubMed.
- L. J. Yu, Y. F. Luo, B. Liao, L. J. Xie, L. Chen, S. Xiao, J. T. Li, S. N. Hu and W. S. Shu, Comparative transcriptome analysis of transporters, phytohormone and lipid metabolism pathways in response to arsenic stress in rice (Oryza sativa), New Phytol., 2012, 195, 97–112 CrossRef CAS PubMed.
- Q. Liu, Novel miRNAs in the control of arsenite levels in rice, Funct. Integr. Genomics, 2012, 12, 649–658 CrossRef CAS PubMed.
- Q. Liu and H. Zhang, Molecular identification and analysis of arsenite stress-responsive miRNAs in rice, J. Agric. Food Chem., 2012, 60, 6524–6536 CrossRef CAS PubMed.
- D. Weigel, Natural variation in Arabidopsis: from molecular genetics to ecological genomics, Plant Physiol., 2012, 158, 2–22 CrossRef CAS PubMed.
- I. Schmalenbach, L. Zhang, M. Ryngajllo and J. M. Jimenez-Gomez, Functional analysis of the Landsberg erecta allele of FRIGIDA, BMC Plant Biol., 2014, 14, 218 CrossRef PubMed.
- S. Yadav, A. Singh, M. R. Singh, N. Goel, K. K. Vinod, T. Mohapatra and A. K. Singh, Assessment of genetic diversity in Indian rice germplasm (Oryza sativa L.): use of random versus trait-linked microsatellite markers, J. Genet., 2013, 92, 545–557 CrossRef CAS PubMed.
- R. Dave, R. D. Tripathi, S. Dwivedi, P. Tripathi, G. Dixit, Y. K. Sharma, P. K. Trivedi, F. J. Corpas, J. B. Barroso and D. Chakrabarty, Arsenate and arsenite exposure modulate antioxidants and amino acids in contrasting arsenic accumulating rice (Oryza sativa L.) genotypes, J. Hazard. Mater., 2013, 262, 1123–1131 CrossRef CAS PubMed.
- S. Dwivedi, A. Mishra, P. Tripathi, R. Dave, A. Kumar, S. Srivastava, D. Chakrabarty, P. K. Trivedi, B. Adhikari, G. J. Norton, R. D. Tripathi and C. S. Nautiyal, Arsenic affects essential and non-essential amino acids differentially in rice grains: inadequacy of amino acids in rice based diet, Environ. Int., 2012, 46, 16–22 CrossRef CAS PubMed.
- W. J. Liu, Y. G. Zhu, F. A. Smith and S. E. Smith, Do phosphorus nutrition and iron plaque alter arsenate uptake by rice seedlings in hydroponic culture?, New Phytol., 2004, 162, 481–488 CrossRef CAS PubMed.
- D. J. Stekel, Y. Git and F. Falciani, The comparison of gene expression from multiple cDNA libraries, Genome Res., 2000, 10, 2055–2061 CrossRef CAS PubMed.
- A. I. Saeed, V. Sharov, J. White, J. Li, W. Liang, N. Bhagabati, J. Braisted, M. Klapa, T. Currier, M. Thiagarajan, A. Sturn, M. Snuffin, A. Rezantsev, D. Popov, A. Ryltsov, E. Kostukovich, I. Borisovsky, Z. Liu, A. Vinsavich, V. Trush and J. Quackenbush, TM4: a free, open-source system for microarray data management and analysis, Biotechniques, 2003, 34, 374–378 CAS.
- C. Li and W. H. Wong, Model-based analysis of oligonucleotide arrays: expression index computation and outlier detection, Proc. Natl. Acad. Sci. U. S. A., 2001, 98, 31–36 CrossRef CAS.
- X. Dai and P. X. Zhao, psRNATarget: a plant small RNA target analysis server, Nucleic Acids Res., 2011, 39, W155–W159 CrossRef CAS PubMed.
- Z. Du, X. Zhou, Y. Ling, Z. Zhang and Z. Su, agriGO: a GO analysis toolkit for the agricultural community, Nucleic Acids Res., 2010, 38, W64–W70 CrossRef CAS PubMed.
- X. Jian, L. Zhang, G. Li, L. Zhang, X. Wang, X. Cao, X. Fang and F. Chen, Identification of novel stress-regulated microRNAs from Oryza sativa L, Genomics, 2010, 95, 47–55 CrossRef CAS PubMed.
- M. Lescot, P. Dehais, G. Thijs, K. Marchal, Y. Moreau, Y. Van de Peer, P. Rouze and S. Rombauts, PlantCARE, a database of plant cis-acting regulatory elements and a portal to tools for in silico analysis of promoter sequences, Nucleic Acids Res., 2002, 30, 325–327 CrossRef CAS PubMed.
- M. F. Hughes, Arsenic toxicity and potential mechanisms of action, Toxicol. Lett., 2002, 133, 1–16 CrossRef CAS.
- A. E. Pasquinelli, B. J. Reinhart, F. Slack, M. Q. Martindale, M. I. Kuroda, B. Maller, D. C. Hayward, E. E. Ball, B. Degnan, P. Muller, J. Spring, A. Srinivasan, M. Fishman, J. Finnerty, J. Corbo, M. Levine, P. Leahy, E. Davidson and G. Ruvkun, Conservation of the sequence and temporal expression of let-7 heterochronic regulatory RNA, Nature, 2000, 408, 86–89 CrossRef CAS PubMed.
- Y. G. Zhu and B. P. Rosen, Perspectives for genetic engineering for the phytoremediation of arsenic-contaminated environments: from imagination to reality?, Curr. Opin. Biotechnol., 2009, 20, 220–224 CrossRef CAS PubMed.
- P. Brodersen, L. Sakvarelidze-Achard, M. Bruun-Rasmussen, P. Dunoyer, Y. Y. Yamamoto, L. Sieburth and O. Voinnet, Widespread translational inhibition by plant miRNAs and siRNAs, Science, 2008, 320, 1185–1190 CrossRef CAS PubMed.
- O. Voinnet, Origin, biogenesis, and activity of plant microRNAs, Cell, 2009, 136, 669–687 CrossRef CAS PubMed.
- M. Aida, T. Ishida and M. Tasaka, Shoot apical meristem and cotyledon formation during Arabidopsis embryogenesis: interaction among the cup-shaped cotyledon and shoot meristemless genes, Development, 1999, 126, 1563–1570 CAS.
- C. C. Baker, P. Sieber, F. Wellmer and E. M. Meyerowitz, The early extra petals1 mutant uncovers a role for microRNA miR164c in regulating petal number in Arabidopsis, Curr. Biol., 2005, 15, 303–315 CrossRef CAS PubMed.
- P. Sieber, F. Wellmer, J. Gheyselinck, J. L. Riechmann and E. M. Meyerowitz, Redundancy and specialization among plant microRNAs: role of the MIR164 family in developmental robustness, Development, 2007, 134, 1051–1060 CrossRef CAS PubMed.
- S. Kumar, M. H. Asif, D. Chakrabarty, R. D. Tripathi and P. K. Trivedi, Differential expression and alternative splicing of rice sulphate transporter family members regulate sulphur status during plant growth, development and stress conditions, Funct. Integr. Genomics, 2011, 11, 259–273 CrossRef CAS PubMed.
- G. Liang, F. Yang and D. Yu, MicroRNA395 mediates regulation of sulfate accumulation and allocation in Arabidopsis thaliana, Plant J., 2010, 62, 1046–1057 CAS.
- C. A. Matthewman, C. G. Kawashima, D. Huska, T. Csorba, T. Dalmay and S. Kopriva, miR395 is a general component of the sulfate assimilation regulatory network in Arabidopsis, FEBS Lett., 2012, 586, 3242–3248 CrossRef CAS PubMed.
- H. S. Guo, Q. Xie, J. F. Fei and N. H. Chua, MicroRNA directs mRNA cleavage of the transcription factor NAC1 to downregulate auxin signals for arabidopsis lateral root development, Plant Cell, 2005, 17, 1376–1386 CrossRef CAS PubMed.
- J. Curaba, M. Talbot, Z. Li and C. Helliwell, Over-expression of microRNA171 affects phase transitions and floral meristem determinancy in barley, BMC Plant Biol., 2013, 13, 6 CrossRef CAS PubMed.
- G. Liang, H. He and D. Yu, Identification of nitrogen starvation-responsive microRNAs in Arabidopsis thaliana, PLoS One, 2012, 7, e48951 CAS.
- A. C. Mallory and H. Vaucheret, Functions of microRNAs and related small RNAs in plants, Nat. Genet., 2006, 38(suppl), S31–S36 CrossRef CAS PubMed.
- S. Dwivedi, R. D. Tripathi, P. Tripathi, A. Kumar, R. Dave, S. Mishra, R. Singh, D. Sharma, U. N. Rai, D. Chakrabarty, P. K. Trivedi, B. Adhikari, M. K. Bag, O. P. Dhankher and R. Tuli, Arsenate exposure affects amino acids, mineral nutrient status and antioxidants in rice (Oryza sativa L.) genotypes, Environ. Sci. Technol., 2010, 44, 9542–9549 CrossRef CAS PubMed.
- M. Shri, R. Dave, S. Diwedi, D. Shukla, R. Kesari, R. D. Tripathi, P. K. Trivedi and D. Chakrabarty, Heterologous expression of Ceratophyllum demersum phytochelatin synthase, CdPCS1, in rice leads to lower arsenic accumulation in grain, Sci. Rep., 2014, 4, 5784 Search PubMed.
- D. Shukla, R. Kesari, S. Mishra, S. Dwivedi, R. D. Tripathi, P. Nath and P. K. Trivedi, Expression of phytochelatin synthase from aquatic macrophyte Ceratophyllum demersum L. enhances cadmium and arsenic accumulation in tobacco, Plant Cell Rep., 2012, 31, 1687–1699 CrossRef CAS PubMed.
- H. Z. Zhang, Y. M. Luo, H. B. Zhang, J. Song, Y. S. Chen, J. Q. Xia and Q. G. Zhao, Characterizing the plant uptake factor of As, Cd and Pb for rice and wheat cereal, HuanJing KeXue, 2010, 31, 488–495 Search PubMed.
- R. Bari, B. Datt Pant, M. Stitt and W. R. Scheible, PHO2, microRNA399, and PHR1 define a phosphate-signaling pathway in plants, Plant Physiol., 2006, 141, 988–999 CrossRef CAS PubMed.
- F. J. Zhao, J. F. Ma, A. A. Meharg and S. P. McGrath, Arsenic uptake and metabolism in plants, New Phytol., 2009, 181, 777–794 CrossRef CAS PubMed.
- M. Kasuga, Q. Liu, S. Miura, K. Yamaguchi-Shinozaki and K. Shinozaki, Improving plant drought, salt, and freezing tolerance by gene transfer of a single stress-inducible transcription factor, Nat. Biotechnol., 1999, 17, 287–291 CrossRef CAS PubMed.
- B. Zhao, R. Liang, L. Ge, W. Li, H. Xiao, H. Lin, K. Ruan and Y. Jin, Identification of drought-induced microRNAs in rice, Biochem. Biophys. Res. Commun., 2007, 354, 585–590 CrossRef CAS PubMed.
- X. Qi, Y. Zhang and T. Chai, Characterization of a novel plant promoter specifically induced by heavy metal and identification of the promoter regions conferring heavy metal responsiveness, Plant Physiol., 2007, 143, 50–59 CrossRef CAS PubMed.
- L. Zhou, Y. Liu, Z. Liu, D. Kong, M. Duan and L. Luo, Genome-wide identification and analysis of drought-responsive microRNAs in Oryza sativa, J. Exp. Bot., 2010, 61, 4157–4168 CrossRef CAS PubMed.
Footnote |
† Electronic supplementary information (ESI) available: Fig. S1: Venn diagram showing the numbers of common and unique differentially expressed miRNAs induced by As-stress. (A) Up/down-regulated miRNAs identified with a 1-fold change in expression level, respectively, in rice cultivars HARG under As(III) and As(V) stress. (B) Up/down-regulated miRNAs identified with a 1-fold change in expression level, respectively, in rice cultivars LARG under As(III) and As(V) stress. Table S1 list of oligonucleotides used for the expression analysis of target genes. Table S2 list of differentially expressed miRNAs in HARG and LARG rice cultivars in As(III) and As(V) stress. Table S3 identified common motifs in the upstream regions of As-stress induced miRNAs. Table S4 metal-responsive elements in As-responsive miRNAs promoters. See DOI: 10.1039/c4mt00264d |
|
This journal is © The Royal Society of Chemistry 2015 |
Click here to see how this site uses Cookies. View our privacy policy here.