Cationic azacryptands as selective three-way DNA junction binding agents†
Received
29th August 2014
, Accepted 15th October 2014
First published on 15th October 2014
Abstract
DNA damaging agents are among the most powerful anticancer drugs currently in clinical use. As an alternative to irreversible nucleobase damage and DNA strand breaks, the non-covalent stabilization of unusual, non-B DNA structures is currently emerging as a promising way to cause DNA damage with a high level of specificity. One of such non-B DNA structures is the three-way DNA junction: this Y-shaped multi-stranded architecture may act as an impediment to many DNA transactions, being therefore regarded as an invaluable target to create genomic defects that are improperly dealt with by cancer cells only. Herein, we report on a series of cationic azacryptands that make excellent candidates for assessing and harnessing the actual therapeutic potential of three-way DNA junction interacting compounds.
Introduction
DNA transactions, chiefly replication and transcription, lead to local DNA deformations as a way to relax the helical stress that arises from the progression of the DNA/RNA-polymerases.1 This torsional stress, known as supercoiling, leads to the formation of a panel of unusual DNA structures (plectonemic and toroidal supercoils, branched DNA junctions, triplex- and quadruplex-DNA, etc.) which could act as impediments to DNA transactions (Fig. 1) if not effectively processed by the cellular machinery (helicases, resolving enzymes). While great emphasis has been recently placed on quadruplexes,2 branched DNA like three- and four-way DNA junctions have been far less studied,3 despite an initial impetus more than 20 years ago.4 Recent investigations have highlighted that DNA junctions could be valuable in cellulo targets:5 indeed, their stabilization by external agents might prevent normal enzymatic processing of DNA, thus triggering genomic instability, notably via stalled replication fork or stumbled RNA polymerase progression, and activating the DNA damage response machinery. Junction-specific small molecules can thus be considered as a novel class of DNA transaction inhibitors, sequestering sequences under unusual conformations (blocking the activity of junction-resolving enzymes) rather than directly inhibiting the polymerases (competitive binding to their active sites).6
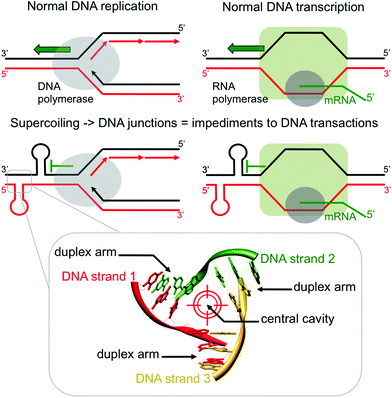 |
| Fig. 1 Schematic representations of the formation of three-way DNA junctions and their implications as impediments to DNA transaction (upper panel) and of the detailed topological specificities of a three-way DNA junction (lower panel). | |
In recent years, Michael J. Hannon and co-workers have created momentum across the field of DNA junction-specific binding agents, notably via their thorough studies of dimetallic supramolecular cylinders as three-way interacting compounds (Fig. 2).7 These complexes, in which three multidentate organic ligands (often named helicands, for disambiguation) wrap around two metallic centres, thrive from the pioneering work Jean-Marie Lehn and co-workers undertook more than 30 years ago on metallo-organic helices (or helicates).8 Since then, such supramolecular assemblies have continued to fascinate organic, inorganic and thus, more recently, biological chemists: a growing number of studies are now reported on metal-templated helices as molecular tools to decipher key biological questions. Notably, metallohelices – referred to as helicates8 or flexicates9 depending on the homo- or heterochiral relationship between the two metallic centres – have been thoroughly studied for their interactions with nucleic acids, since their high cationic nature makes them prone to interact readily with negatively charged DNA and RNA. Dimetallic cylinders have thus proven invaluable multitasking tools for targeting a panel of higher-order nucleic acid structures including duplex-DNA (major groove binding),10 quadruplex-DNA (external stacking on both monomeric11 and dimeric quadruplexes)12 and both DNA and RNA three-way junctions (junction central cavity binding).7,13 The anti-proliferative properties of metallohelices (anticancer and antibiotic activities of helicates and flexicates, respectively)9,14 have also been investigated; these properties, admittedly very interesting and promising, are still to be thoroughly investigated since the relationship between the DNA interaction and the growth inhibitory effect is not straightforward and has been recently questioned.15
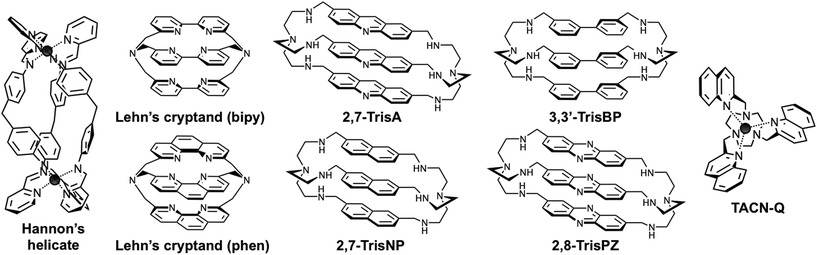 |
| Fig. 2 Structures of pioneering Hannon's helicate and Lehn's azacryptands, and compounds used in the present study. | |
In the present study, we will focus on the small molecule-based recognition of three-way DNA junctions. Interestingly, supramolecular cylinders interact efficiently with three-way junctions thanks to an exquisite shape complementarity between the metallohelices and the central cavity of the junctions, as firmly demonstrated by X-ray analyses of crystals of metallohelices/three-way junction complexes.7,13 Herein, we would like to make another leap on the road toward efficient junction interacting compounds, reporting on cationic azacryptands that are found to be valuable three-way junction binding agents. The cylinder-like shape of the azacryptands makes them capable of fitting snugly into the junction cavity (like the supramolecular cylinders) but, in contrast to metallohelices, they contain flexible aromatic arms, free to rotate and consequently able to adjust their orientation to create efficient π-stacking interactions with the base pairs that form the side walls of the cavity. Therefore, azacryptands reported herein are found to cling firmly to the cavity, eliciting not only high affinity and selectivity levels for three-way DNA junctions but also enticing three-way chaperone activities. These results, along with interesting yet preliminary anti-proliferative activities against melanoma cells, make cationic azacryptands invaluable candidates to better assess and harness the actual therapeutic potential of three-way DNA junction interacting compounds.
Results and discussion
Cationic azacryptands as three-way junction binding agents
The very first prototypes of cryptands – also referred to as macrobicyclic ligands, photoactive cryptands or cyclo-tris-intercalators (CTI) – were, again, originally reported by Jean-Marie Lehn and co-workers in 1984 (Fig. 2).16 Bipyridine and phenanthroline were first used as “intercalator” moieties to make cryptands prone to firmly chelate metals within their intramolecular “crypt”-like cavities, notably high-valent lanthanides (terbium, europium) to exploit the luminescence properties of the resulting complexes in water.17 Herein, cationic azacryptands were selected as models for three-way junction ligands not only for their shapes but also because prototypes like acridine trimers (named 2,7-TrisA, Fig. 2)18 were already known to interact with DNA (via electrostatic and π-stacking interactions only).19 More recently, naphthalene (NP), biphenyl (BP) and phenazine (PZ) trimers (named 2,7-TrisNP, 3,3′-TrisBP and 2,8-TrisPZ, respectively, Fig. 2) were also included in high-throughput screening of cyclo-bis-intercalator and cyclo-tris-intercalator libraries to identify promising quadruplex ligands, with varying degrees of success (in terms of affinity and selectivity).20 In these evaluations, the extent to which the aromatic nature of the “intercalator” moieties was influential (stacking interactions with the accessible G-quartet versus electrostatically driven interactions with loops and grooves of quadruplexes) remained to be elucidated. Herein, we would like to fully exploit all the specific features of the azacryptands to make them invaluable three-way junction interacting agents, that is their charges to promote the electrostatic interactions with the junctions, their shapes to slide into the junction central cavity, and their aromaticity to firmly cling to this structurally unique binding site. As a result, azacryptands should display high affinity and selectivity levels for three-way junctions.
Syntheses and characterization of cationic azacryptands
The synthesis of azacryptands is fully detailed in the ESI.† Briefly, they were obtained via a [3 + 2]-type condensation21 of aromatic dialdehydes22 with tris(2-aminoethyl)amine, followed by reduction of macrobicyclic hexaimines with NaBH4. The azacryptands were obtained with moderate overall yields (21–31%) and used as water-soluble hydrochloride salts (2,7-TrisNP and 3,3′-TrisBP) or as a free base (2,8-TrisPZ), readily soluble in a diluted acid. All synthetic procedures, purifications and characterization can be found in the ESI.†
DNA junction interacting properties of cationic azacryptands
To gain insights into the affinity of azacryptands for three-way junctions, we implemented a series of FRET-melting experiments with the doubly labelled junction-forming sequence fam-TWJ-tamra (fam-A(CT)2(TC)2G-T6-C(GA)2GCGAC-T6-GTCGC(AG)2T-tamra, with fam standing for 6-fluorescein amidite and tamra standing for 6-carboxytetramethylrhodamine).23 Briefly, the stability of the DNA junction is evaluated through its thermal unfolding readily monitored via a modification of the fluorescence resonance energy transfer (FRET) relationship that occurs between fam and tamra probes present at both ends of the sequence. Semi-quantitative evaluation of the ligand binding affinity is obtained by measuring the increase in melting temperature imparted by the ligand (ΔT1/2, in °C). Herein, experiments were carried out with fam-TWJ-tamra (0.2 μM) and increasing amounts (0–1 μM) of 2,7-TrisNP, 3,3′-TrisBP and 2,8-TrisPZ as well as TACN-Q, a small molecule previously reported as displaying fair affinity but an exquisite selectivity for three-way junctions.24 As seen in Fig. 3A and Table 1, all compounds stabilize fam-TWJ-tamra in a dose–response manner (i.e., the more, the better), but to a significantly different extent, with ΔT1/2 values between 3.8 and 14.6 °C at 1 μM ligand (2,7-TrisNP > 3,3′-TrisBP > 2,8-TrisPZ > TACN-Q). Both 2,7-TrisNP and 3,3′-TrisBP can thus be considered as high-affinity ligands, with ΔT1/2 values higher than 10 °C. We thus decided to assess the selectivity of these compounds for the DNA junction against an excess of unlabelled duplex-DNA (ds26, selected for its high thermal stability (Tm > 85 °C) under the conditions of the assay). As seen in Fig. 3B (Table 1 and ESI†), they exhibit excellent selectivity for DNA junctions, with remaining fam-TWJ-tamra stabilizations between 82 and 92% in the presence of 50 equiv. of ds26 (3,3′-TrisBP > 2,8-TrisPZ = TACN-Q > 2,7-TrisNP). The lack of duplex-DNA stabilization was also directly assessed via FRET-melting experiments performed with the doubly labelled duplex-forming sequence fam-DS-tamra (fam-(TA)2GC(TA)2-T6-(TA)2GC(TA)2-tamra):23 the presence of 1 μM of ligands results in very low stabilizations only, with ΔT1/2 values between 0 and 2.0 °C (see ESI† and Table 1). Finally, because 2,7-TrisNP, 3,3′-TrisBP and 2,8-TrisPZ were previously studied for their quadruplex-interacting properties (vide supra), we decided to investigate their selectivity against an excess of unlabelled quadruplex-DNA (TG5T, also selected for its high thermal stability (Tm > 85 °C) under the conditions of the assay). As seen in Fig. 3C (Table 1 and ESI†), all compounds are indeed found sensitive to the presence of quadruplexes, with remaining fam-TWJ-tamra stabilizations between 64 and 87% in the presence of 50 equiv. of TG5T (TACN-Q > 2,7-TrisNP = 2,8-TrisPZ > 3,3′-TrisBP), but to a limited extent only given the fierce competition. Again, their quadruplex affinity was also directly monitored via FRET-melting experiments performed with the doubly labelled quadruplex-forming sequence fam-GQ-tamra (also known as F21T, fam-G3(T2AG3)3-tamra):25 the presence of 1 μM of ligands results in low (TACN-Q, 3,3′-TrisBP) to high stabilizations (2,8-TrisPZ, 2,7-TrisNP), with ΔT1/2 values between 1.7 and 11.5 °C (see ESI† and Table 1). Collectively, these results indicate that azacryptands (like supramolecular cylinders) indeed exhibit a significant quadruplex affinity, but the competitive experiments clearly indicate that azacryptands, when given a choice between junctions and quadruplexes, elicit a preferential affinity for the junctions. Altogether, this series of FRET-melting results clearly shows that azacryptands are valuable DNA junction ligands, especially enlightening 3,3′-TrisBP as the best compromise between affinity and selectivity.
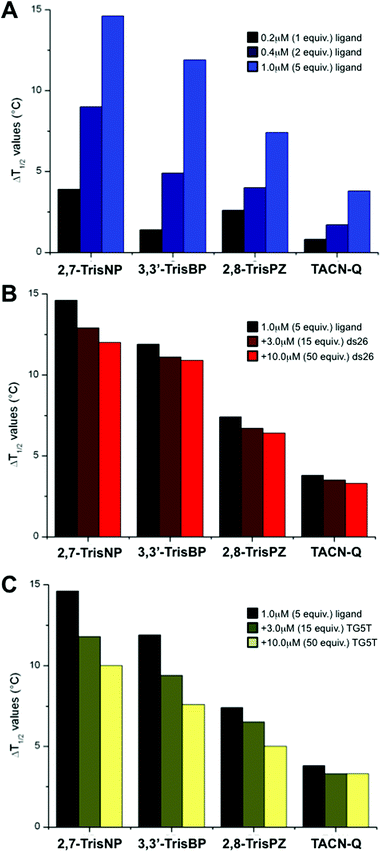 |
| Fig. 3 (A) FRET-melting results of experiments carried out with fam-TWJ-tamra (0.2 μM) and increasing amounts (0–1.0 μM) of azacryptands and TACN-Q. (B, C) Competitive FRET-melting results of experiments carried out with fam-TWJ-tamra (0.2 μM) with azacryptands/TACN-Q (1.0 μM) and increasing amounts (3.0–10.0 μM) of unlabelled competitors (i.e., ds26 (B) or TG5T (C)). All experiments were performed in 10 mM lithium cacodylate buffer (pH 7.2) + 99 mM LiCl/1 mM KCl. | |
Table 1 Affinity of cationic azacryptands and TACN-Q for three-way DNA junctions and selectivity versus both duplex- and quadruplex-DNAa
|
2,7-TrisNP |
3,3′-TrisBP |
2,8-TrisPZ |
TACN-Q |
All experiments were carried out in 100 μL final volume of 10 mM lithium cacodylate buffer (pH 7.2) + 99 mM LiCl/1 mM KCl, with 0.2 μM of labelled DNA, without or with ligands (0–1.0 μM, i.e., 0–5 equiv.), in the absence or presence of competitive unlabelled oligonucleotides (ds26 or TG5T, 0–10.0 μM).
CD-melting experiments carried out in 600 μL final volume of 10 mM lithium cacodylate buffer (pH 7.2) + 99 mM LiCl/1 mM KCl, with 1.0 μM of TWJ, without or with ligands (3.0 μM, i.e., 5 equiv.).
|
|
Dose response FRET-melting experiments (fam-TWJ-tamra) |
0.2 μM |
3.9 °C |
1.4 °C |
2.6 °C |
0.8 °C |
0.4 μM |
9.0 °C |
4.9 °C |
4.0 °C |
1.7 °C |
1.0 μM |
14.6 °C |
11.9 °C |
7.4 °C |
3.8 °C |
|
Competition of fam-TWJ-tamra versus duplex-DNA (ds26) |
0 |
14.6 °C |
11.9 °C |
7.4 °C |
3.8 °C |
3.0 μM |
12.9 °C (88%) |
11.1 °C (93%) |
6.7 °C (91%) |
3.5 °C (92%) |
10.0 μM |
12.0 °C (82%) |
10.9 °C (92%) |
6.4 °C (86%) |
3.3 °C (86%) |
|
Control FRET-melting experiments (fam-DS-tamra) |
1.0 μM |
2.0 °C |
1.4 °C |
0 °C |
0 °C |
|
Competition of fam-TWJ-tamra vs. quadruplex-DNA (TG5T) |
0 |
14.6 °C |
11.9 °C |
7.4 °C |
3.8 °C |
3.0 μM |
11.8 °C (81%) |
9.4 °C (79%) |
6.5 °C (88%) |
3.3 °C (87%) |
10.0 μM |
10.0 °C (68%) |
7.6 °C (64%) |
5.0 °C (68%) |
3.3 °C (87%) |
|
Control FRET-melting experiments (fam-GQ-tamra) |
1.0 μM |
11.5 °C |
2.5 °C |
6.1 °C |
1.7 °C |
|
CD-melting experiments (TWJ)b |
3.0 μM |
11.1 °C |
10.0 °C |
6.9 °C |
4.4 °C |
EMSA analysis of the cationic azacryptand/junction assemblies
The electrophoretic mobility shift assay (EMSA) is another interesting technique since it enables assessment of the ability of ligands not only to interact with but also to assemble DNA junctions. We decided to perform polyacrylamide gel electrophoresis (PAGE) with the aforementioned candidates along with several control compounds to gain insights into the molecular features that might govern the efficiency of the ligand–junction interactions: two highly selective quadruplex ligands, PNADOTASQ26 and AuTMX2,27 known to interact efficiently with non-B DNA structures, π-stacking on accessible guanine assemblies (chiefly the G-quartet), and the “universal” DNA ligand TMPyP4, able to bind to DNA whatever its secondary structures,23,28 and historically, among the very first reported branched DNA interacting compounds.29 PAGE was performed with a three-way junction that results from the trimolecular assembly of CG2A2CG2CACTCG (strand 1 or s1), CGAGTGCAGCGTG2 (s2) and C2ACGCTCGT2C2G (s3), already investigated by Viktor Brabec and co-workers.30 As seen in Fig. 4A, the first experiment (20% polyacrylamide, 1× TBE, 4 °C, 2.5 hours, post-staining with SYBR® Safe) performed at 1
:
1 DNA
:
ligand ratio (5 μM) clearly shows that three compounds only display very interesting properties under these conditions, namely 3,3′-TrisBP, 2,7-TrisNP and TACN-Q: they are indeed able to assemble the three-way junction from a mixture of the three constitutive strands (“s1 + s2 + s3”), as demonstrated by the three slower migration bands that correspond to the folded junction/ligand complexes (2,7-TrisNP ≥ 3,3′-TrisBP > TACN-Q, in light of the remaining “s1 + s2 + s3” bands). These results provide also valuable insights into the affinity of the ligand for their DNA target since the resulting non-covalent DNA/ligand adducts are stable enough to withstand the harsh electrophoretic conditions. Interestingly, the compounds with the highest affinity (FRET-melting results), namely 3,3′-TrisBP and 2,7-TrisNP, gave the most intense bands, while TACN-Q leads to a stable adduct when 2,8-TrisPZ, despite a higher stabilizing ability, is hardly visible. The discrepancies between FRET-melting and EMSA results certainly originate from the mechanisms involved and monitored by the two assays, that is stabilization to prevent thermal unfolding (FRET-melting) versus chaperone activities and electric field-directed migration (PAGE). Satisfyingly, none of the control compounds (PNADOTASQ, AuTMX2 and TMPyP4) provides discernable adducts (traces can be detected with TMPyP4), thereby clearly demonstrating the specificity of the three-way junction/azacryptands and TACN-Q associations. To fathom further their junction folding properties, we implemented dose–response experiments with both 3,3′-TrisBP (owing to its enticing FRET-melting and PAGE results) and 2,8-TrisPZ (because of its rather disappointing PAGE results): as shown in Fig. 4B and C, concentrations higher than 5 μM (1 equiv.) of 3,3′-TrisBP are enough to almost fully assemble the junction, while concentrations higher than 10 μM (2 equiv.) are required to visualize the junction/2,8-TrisPZ adducts, but to a marginal extent only. Collectively, these results emphasize another important feature of the ligands studied herein (azacryptands and TACN-Q), in addition to their exquisite junction stabilizing properties, that is their capability to promote the formation of three-way DNA structures from unfolded sequences.
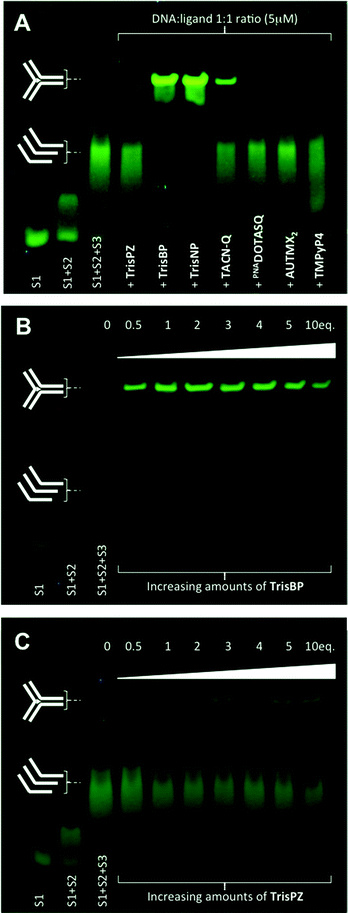 |
| Fig. 4 Native PAGE analysis (20% polyacrylamide, 1× TBE, 4 °C, 2.5 hours, fluorescence visualization (λex = 254 nm) after post-staining (10 min, 25 °C) with SYBR® Safe) of the three-way junction assembling properties either of a panel of small molecules (A) at 1 : 1 DNA : ligand ratio (5 μM) or of increasing concentrations (from 0 to 10 equiv.) of both 3,3′-TrisBP (B) or 2,8-TrisPZ (C). | |
Investigations about the junction binding mode of azacryptands
We tried to gain insights into the actual binding mode of azacryptands via circular dichroism (CD) experiments.31 Experiments were performed with azacryptands and a trimolecular DNA junction that results from the assembly of G2TG2C(GA)2GCGACGATC2, G2A(TCG)2C(AG)2T2GAC2 and G2TCA2(CT)2(TC)2GC2AC2.23 Azacryptands are achiral ligands, therefore devoid of any CD signal; however, if they nestle in the cavity, the chiral pocket may affect differentially their electronic absorption bands, making them eliciting CD properties, known as induced CD signals. Herein, the overlap between azacryptands and DNA absorbance makes the results difficult to interpret. However, we found that addition of 2,8-TrisPZ (5 μM) to TWJ (1 μM) results in the emergence of a small positive CD band centred on its own absorbance maximum (see ESI†), inferring a yet efficient but rather ill-defined interaction. Interestingly, the addition of 3,3′-TrisBP (see ESI†) and even more significantly of 2,7-TrisNP (Fig. 5) leads to split bands with balanced positive and negative contributions in its absorbance region (exciton splitting), as usually observed for tight binding to chiral hosts. These results thus suggest that these azacryptands actually bind to the junction cavity. We found that CD spectra were also suitable for performing melting experiments (a CD signal at 278 nm as a function of temperature); we therefore performed CD-melting experiments with A(CT)2(TC)2G-T6-C(GA)2GCGAC-T6-GTCGC(AG)2T, which is the unlabelled counterpart of the intramolecular three-way forming oligonucleotide used for FRET-melting experiments, in the presence of 5 equiv. of ligands. Quite satisfyingly the obtained results (see ESI†) confirm not only the stabilizing capability but also the ranking determined by the FRET-melting assay (2,7-TrisNP > 3,3′-TrisBP > 2,8-TrisPZ > TACN-Q, with ΔTm = 11.1, 10.0, 6.9 and 4.4 °C, respectively, Table 1). To further assess the cavity binding ability of azacryptands, we tried to label the cavity with a turn-on fluorescent reporter, in order to implement ligand displacement assays (also termed fluorescent intercalator displacement (FID) experiments).32 TO-PRO-332,33 was selected as a cavity-selective dye in light of its clear 1
:
1 association model (see ESI†); upon addition of increasing amounts (0–5 equiv.) of azacryptands, all compounds were found to displace quite efficiently TO-PRO-3 from the DNA junction (with displacement concentrations, DC50 = 1.21, 1.32 and 1.36 μM for 2,7-TrisNP, 3,3′-TrisBP and 2,8-TrisPZ, respectively), implying again that they might indeed interact with the cavity. Of course, these results are not definitive proof but, along with CD measurements, they provide converging arguments to support the cavity binding ability of the cationic azacryptands.
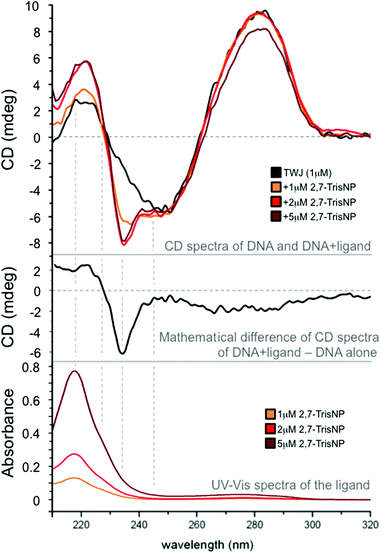 |
| Fig. 5 CD spectra of a DNA junction (1 μM) upon addition of increasing amounts (0–5 μM) of 2,7-TrisNP (upper panel); UV-Vis spectra of increasing amounts (0–5 μM) of 2,7-TrisNP (lower panel); mathematical difference between CD spectra of the TWJ/2,7-TrisNP assembly (1 : 5 ratio) and that of the DNA alone to highlight the exciton splitting that results from the ligand binding to a chiral pocket (the junction cavity). All experiments were performed in 10 mM lithium cacodylate buffer (pH 7.2) at 25 °C. | |
Preliminary anti-proliferative investigations
In light of these promising results, in terms of affinity, selectivity and chaperone activity, we thus decided to evaluate the anti-proliferative properties of this series of compounds against B16 cells,34 a mouse model for human melanoma. Briefly, the adherent cell line was subcultured in a 96-well plate in supplemented RPMI medium at 37 °C for 48 hours in the presence of increasing concentrations (0–100 μM) of ligands (2,7-TrisNP, 3,3′-TrisBP, 2,8-TrisPZ and TACN-Q). MTT (3-(4,5-dimethylthiazolyl-2)-2,5-diphenyl-tetrazolium bromide) was then used to assess the mitochondrial reductive function of the cells, a marker of cell viability.35 The lethal concentrations (LC50) of each compound were evaluated using the absorbance of the blue formazan crystals that results from the cellular reduction of the MTT, via an optical density determination at 530 nm. As shown in Fig. 6, both 2,7-TrisNP and 3,3′-TrisBP are found to be quite active (with LC50 = 3.42 ± 0.16 and 3.55 ± 0.24 μM, respectively), while TACN-Q exhibits moderate anti-proliferative properties only (LC50 = 10.65 ± 1.28 μM) when 2,8-TrisPZ does not inhibit the cell growth. Quite interestingly, these results fairly parallel the PAGE results (2,7-TrisNP ≥ 3,3′-TrisBP > TACN-Q ⋙ 2,8-TrisPZ): this implies that the toxicity of these compounds might be related to their ability to fold three-way junctions rather than to stabilize already folded ones; this assumption will nevertheless require in depth in cellulo investigations to be firmly demonstrated. Anyway, these results further demonstrate that azacryptands and TACN-Q might be considered as interesting candidates for further evaluations that will allow us to better assess and harness the actual therapeutic potential of three-way junction ligands, and beyond this, make EMSA a more accurate, reliable assay for the identification of future candidates with promising anti-proliferative properties.
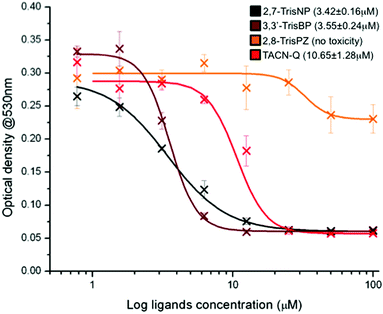 |
| Fig. 6 Anti-proliferative activity of azacryptands and TACN-Q evaluated through a MTT cell proliferation assay conducted with B16 cells, via optical density measurements at 530 nm (characteristic of formazan crystals that results from the mitochondrial reduction of the MTT in living cells) as a function of the ligand concentrations. | |
Conclusions
Most of the drugs in current clinical use derive their effects from the generation of DNA lesions.36 This class of chemotherapeutics, called DNA damaging agents, poses a threat to cell survival, notably for cancerous cells that are generally more defective in DNA repair machinery (termed DNA damage response, DDR) than their healthy counterparts.37 Beyond direct DNA lesions, via covalent nucleobase modifications for instance (alkylation, platination, photo-oxidation, etc.), the stabilization of non-B DNA structures is currently emerging as a novel and promising strategy to trigger DNA defects: alternative higher-order DNA structures are normal physical consequences of a transient single-stranded DNA exposure or torsional stress related to the translocation along the DNA template of the proteins in charge of the DNA transactions. Therefore, unusual structures must be dealt with efficiently by the cellular machinery to ensure viable and reliable replication/transcription. If stabilized, alternative structures might pose a serious challenge to DNA transactions, impeding the normal DNA/RNA polymerase processivity; persistent stalling provides a strong DNA damage signal that leads to the recruitment of the DDR machinery.38
The correlation between small molecule stabilization of alternative higher-order DNA structures and DDR was recently firmly established: the selective quadruplex interacting agent, pyridostatin, creates genome-wide DNA damage hotspots that trigger DDR signals.39 Other quadruplex ligands induce DDR via alternative mechanisms, notably telomere dysfunctions (uncapping)40 or phosphorylation-dependent pathways (ataxia-telangiectasia mutated (ATM)41 or ATM-Rad3-related (ATR)42 kinases). Herein, we expand the repertoire of small molecules with specific unusual DNA structure interacting properties that may act as valuable tools to decipher the anticancer potential of these novel DNA targets.43 To this end, we report on a series of compounds that exhibit not only high affinity for three-way DNA junctions but also exquisite selectivity over duplex-DNA, along with an enticing ability to fold DNA junctions from unassembled sequences. Three of the compounds studied herein (2,7-TrisNP, 3,3′-TrisBP and 2,8-TrisPZ) belong to the family of cationic azacryptands, while TACN-Q is a simplified model of metallohelices, the pioneering prototypes of three-way DNA junction ligands. Three of these compounds (i.e., 2,7-TrisNP, 3,3′-TrisBP and TACN-Q) display promising anti-proliferative properties, although it is premature to establish a firm relationship between junction stabilization/folding and growth inhibitory activities. Additionally, all compounds display marginal to significant quadruplex stabilizing properties; this might be a therapeutic advantage since it may imply a concomitant deregulation of multiple unusual DNA structure-involving cellular processes.
In conclusion, even if admittedly preliminary, the wealth of data presented herein are important since they cast light on a novel family of promising three-way DNA junction ligands, paving the way for innovative molecular designs and providing a solid basis for future investigations to be launched. Massive efforts are now to be invested to decipher the actual origin of their cellular activity; nevertheless, we can already wager that many future studies will soon confirm the great therapeutic potential of this new class of unusual DNA structure binding agents.
Acknowledgements
The authors sincerely thank Loic Stefan for fruitful discussions and preliminary investigations, the Centre National de la Recherche Scientifique (CNRS), Université de Bourgogne (uB) and the Conseil Régional de Bourgogne (CRB) for funding (3MIM and PARI-SSTIC projects), the Ministère de l'Enseignement Supérieur et de la Recherche for a Ph.D. grant to Aurélien Laguerre and the European Action Scheme for the Mobility of University Students (ERASMUS) program and the Institute of Chemical Technology of Prague for funding the Jana Novotna's traineeship.
Notes and references
- B. P. Belotserkovski, S. M. Mirkin and P. C. Hanawalt, Chem. Rev., 2013, 113, 8620 CrossRef PubMed.
- G. W. Collie and G. N. Parkinson, Chem. Soc. Rev., 2011, 40, 5867 RSC; N. Maizels and L. T. Gray, PLOS Genet., 2013, 9, e1003468 Search PubMed.
- D. M. J. Lilley, Q. Rev. Biophys., 2000, 33, 109 CrossRef CAS PubMed.
- M. Lu, Q. Guo and N. R. Kallenbach, Crit. Rev. Biochem. Mol. Biol., 1992, 27, 157 CrossRef CAS PubMed.
- J. Zhao, A. Bacolla, G. Wang and K. Vasquez, Cell. Mol. Life Sci., 2010, 67, 43 CrossRef CAS PubMed; G. Wang and K. M. Vasquez, DNA Repair, 2014, 19, 143 CrossRef PubMed.
- E. V. Mirkin and S. M. Mirkin, Microbiol. Mol. Biol. Rev., 2007, 71, 13 CrossRef CAS PubMed.
- A. Oleski, A. G. Blanco, R. Boer, I. Uson, J. Aymami, A. Rodger, M. J. Hannon and M. Coll, Angew. Chem., Int. Ed., 2006, 45, 1227 CrossRef PubMed.
- J.-M. Lehn, A. Rigault, J. Siegel, J. Harrowfield, B. Chevrier and D. Moras, Proc. Natl. Acad. Sci. U. S. A., 1987, 84, 2565 CrossRef CAS; R. Krämer, J.-M. Lehn and A. Marquis-Rigault, Proc. Natl. Acad. Sci. U. S. A., 1993, 90, 5394 CrossRef.
- S. E. Howson, A. Bolhuis, V. Brabec, G. J. Clarkson, J. Malina, A. Rodger and P. Scott, Nat. Chem., 2012, 4, 31 CrossRef CAS PubMed.
- J. Malina, M. J. Hannon and V. Brabec, Nucleic Acids Res., 2008, 36, 3630 CrossRef CAS PubMed; Y. Parajo, J. Malina, I. Meistermann, G. J. Clarkson, M. Pascu, A. Rodger, M. J. Hannon and P. Lincoln, Dalton Trans., 2009, 4868 RSC.
- H. Yu, X. Wang, M. Fu, J. Ren and X. Qu, Nucleic Acids Res., 2008, 36, 5695 CrossRef CAS PubMed; C. Zhao, J. Geng, L. Feng, J. Ren and X. Qu, Chem. – Eur. J., 2011, 17, 8209 CrossRef PubMed.
- C. Zhao, L. Wu, J. Ren, Y. Xu and X. Qu, J. Am. Chem. Soc., 2013, 135, 18786 CrossRef CAS PubMed.
- S. Phongtongpasuk, S. Paulus, J. Schabl, R. K. O. Sigel, B. Spingler, M. J. Hannon and E. Freisinger, Angew. Chem., Int. Ed., 2013, 52, 11513 CrossRef CAS PubMed.
- G. I. Pascu, A. C. G. Hotze, C. Sanchez-Cano, B. M. Kariuki and M. J. Hannon, Angew. Chem., Int. Ed., 2007, 46, 4374 CrossRef CAS PubMed; A. C. G. Hotze, N. J. Hodges, R. E. Hayden, C. Sanchez-Cano, C. Paines, N. Male, M.-K. Tse, C. M. Bunce, J. K. Chipman and M. J. Hannon, Chem. Biol., 2008, 15, 1258 CrossRef PubMed; C. Ducani, A. Leczkowska, N. J. Hodges and M. J. Hannon, Angew. Chem., Int. Ed., 2010, 49, 8942 CrossRef PubMed; L. Cardo, V. Sadovnikova, S. Phongtongpasuk, N. J. Hodges and M. J. Hannon, Chem. Commun., 2011, 47, 6575 RSC.
- V. Brabec, S. E. Howson, R. A. Kaner, R. M. Lord, J. Malina, R. M. Phillips, Q. M. A. Abdallah, P. C. McGowan, A. Rodger and P. Scott, Chem. Sci., 2013, 4, 4407 RSC.
- J.-C. Rodriguez-Ubis, B. Alpha, D. Plancherei and J.-M. Lehn, Helv. Chim. Acta, 1984, 67, 2264 CrossRef CAS.
-
B. Dietrich, P. Viout and J.-M. Lehn, Macrocyclic Chemistry, Wiley-VCH, Weinheim, 1993 Search PubMed.
- M.-P. Teulade-Fichou, J.-P. Vigneron and J.-M. Lehn, J. Chem. Soc., Perkin Trans. 2, 1996, 2169 RSC.
- S. Amrane, A. De Cian, F. Rosu, M. Kaiser, E. De Pauw, M.-P. Teulade-Fichou and J.-L. Mergny, ChemBioChem, 2008, 9, 1229 CrossRef CAS PubMed.
- A. Granzhan, D. Monchaud, N. Saettel, A. Guédin, J.-L. Mergny and M.-P. Teulade-Fichou, J. Nucleic Acids, 2010, 2010, ID460561 Search PubMed.
- N. E. Borisova, M. D. Reshetova and Y. A. Ustynyuk, Chem. Rev., 2007, 107, 46 CrossRef CAS PubMed.
- A. Granzhan, E. Largy, N. Saettel and M.-P. Teulade-Fichou, Chem. – Eur. J., 2010, 16, 878 CrossRef CAS PubMed.
- L. Stefan, B. Bertrand, P. Richard, P. Le Gendre, F. Denat, M. Picquet and D. Monchaud, ChemBioChem, 2012, 13, 1905 CrossRef CAS PubMed.
- S. Vuong, L. Stefan, P. Lejault, Y. Rousselin, F. Denat and D. Monchaud, Biochimie, 2012, 94, 442 CrossRef CAS PubMed.
- A. De Cian, L. Guittat, M. Kaiser, B. Saccà, S. Amrane, A. Bourdoncle, P. Alberti, M.-P. Teulade-Fichou, L. Lacroix and J.-L. Mergny, Methods, 2007, 42, 183 CrossRef CAS PubMed; P. A. Rachwal and K. R. Fox, Methods, 2007, 23, 291 CrossRef PubMed; A. Benz, V. Singh, T. U. Mayer and J. S. Hartig, ChemBioChem, 2011, 12, 1422 CrossRef PubMed.
- R. Haudecoeur, L. Stefan, F. Denat and D. Monchaud, J. Am. Chem. Soc., 2013, 135, 550 CrossRef CAS PubMed; R. Haudecoeur, L. Stefan and D. Monchaud, Chem. – Eur. J., 2013, 19, 12739 CrossRef PubMed.
- B. Bertrand, L. Stefan, M. Pirrotta, D. Monchaud, E. Bodio, P. Richard, P. Le Gendre, E. Warmerdam, M. H. H. de Jager, G. M. M. Groothius, M. Picquet and A. Casini, Inorg. Chem., 2014, 53, 2296 CrossRef CAS PubMed.
- J. Ren and J. B. Chaires, Biochemistry., 1999, 38, 16067 CrossRef CAS PubMed; P. Wang, L. Ren, H. He, F. Liang, X. Zhou and Z. Tan, ChemBioChem, 2006, 7, 1155 CrossRef PubMed; E. W. White, F. Tanious, M. A. Ismail, A. P. Reszka, S. Neidle, D. W. Boykin and W. D. Wilson, Biophys. Chem., 2007, 126, 140 CrossRef PubMed; A. Granzhan, H. Ihmels and K. Jäger, Chem. Commun., 2009, 1249 RSC; P. Murat, R. Bonnet, A. Van der Heyden, N. Spinelli, P. Labbé, D. Monchaud, M.-P. Teulade-Fichou, P. Dumy and E. Defrancq, Chem. – Eur. J., 2010, 16, 6106 CrossRef PubMed.
- M. Lu, Q. Guo, R. F. Pasternack, D. J. Wink, N. C. Seeman and N. R. Kallenbach, Biochemistry, 1990, 29, 1614 CrossRef PubMed.
- J. Malina, M. J. Hannon and V. Brabec, Chem. – Eur. J., 2007, 13, 3871 CrossRef CAS PubMed.
-
N. Berova, K. Nakanishi and R. W. Woody, Circular Dichroism: Principles and Applications, Wiley-VCH, Weinheim, 2000 Search PubMed.
- D. L. Boger, B. E. Fink, S. R. Brunette, W. C. Tse and M. P. Hedrick, J. Am. Chem. Soc., 2001, 123, 5878 CrossRef CAS PubMed; W. C. Tse and D. L. Boger, Acc. Chem. Res., 2004, 37, 61 CrossRef PubMed; D. Monchaud, C. Allain and M.-P. Teulade-Fichou, Bioorg. Med. Chem. Lett., 2006, 16, 4842 CrossRef PubMed; E. Largy, F. Hamon and M.-P. Teulade-Fichou, Anal. Bioanal. Chem., 2011, 400, 3419 CrossRef PubMed.
- K. M. Sovenyhazy, J. Bordelon and J. T. Petty, Nucleic Acids Res., 2003, 31, 2561 CrossRef CAS PubMed.
- W. W. Overwijk and N. P. Restifo, Curr. Protoc. Immunol., 2001, 39, 20.1.1 Search PubMed.
- N. J. Marshall, C. J. Goodwin and S. J. Holt, Growth Regul., 1995, 5, 69 CAS.
- S. P. Jackson and J. Bartek, Nature, 2009, 461, 1071 CrossRef CAS PubMed; A. Ciccia and S. J. Elledge, Mol. Cell, 2010, 40, 179 CrossRef PubMed.
- S. A. Martin, C. J. Lord and A. Ashworth, Curr. Opin. Genet. Dev., 2008, 18, 80 CrossRef CAS PubMed.
- S. Lagerwerf, M. G. Vrouwe, R. M. Overmeer, M. I. Fousteri and L. H. F. Mullenders, DNA Repair, 2011, 10, 743 CrossRef CAS PubMed.
- R. Rodriguez, K. M. Miller, J. V. Forment, C. R. Bradshaw, M. Nikan, S. Britton, T. Oelschlaegel, B. Xhemalce, S. Balasubramanian and S. P. Jackson, Nat. Chem. Biol., 2012, 8, 301 CrossRef CAS PubMed.
- D. Gomez, M.-F. O'Donohue, T. Wenner, C. Douarre, J. Macadre, P. Koebel, M.-J. Giraud-Panis, H. Kaplan, A. Kolkes, K. Shin-ya and J.-F. Riou, Cancer Res., 2006, 66, 6908 CrossRef CAS PubMed.
- G. Pennarun, C. Granotier, F. Hoffschir, E. Mandine, D. Biard, L. R. Gauthier and F. D. Boussin, Nucleic Acids Res., 2008, 36, 1741 CrossRef CAS PubMed.
- A. Rizzo, E. Salvati, M. Porru, C. D'Angelo, M. F. Stevens, M. D'Incalci, C. Leonetti, E. Gilson, G. Zupi and A. Biroccio, Nucleic Acids Res., 2009, 37, 5353 CrossRef CAS PubMed.
- L. A. Howell and M. Searcey, ChemBioChem, 2009, 10, 2139 CrossRef CAS PubMed; G. Song and J. Ren, Chem. Commun., 2010, 46, 7283 RSC.
Footnote |
† Electronic supplementary information (ESI) available: Fully detailed synthetic procedures and characterization of cationic azacryptands; preparation and folding of oligonucleotides; experimental procedures for FRET-melting, UV-Vis, fluorescence (TO-PRO-3 titrations, FID), CD and CD-melting experiments, EMSA analyses as well as cell culture protocols and cytotoxicity evaluations. See DOI: 10.1039/c4ob01846j |
|
This journal is © The Royal Society of Chemistry 2015 |
Click here to see how this site uses Cookies. View our privacy policy here.